-
PDF
- Split View
-
Views
-
Cite
Cite
Mrunal Jadhav, Arati Prabhu, Metallic and polymeric green nanoplatforms in oncology, Journal of Applied Microbiology, Volume 134, Issue 1, January 2023, lxac044, https://doi.org/10.1093/jambio/lxac044
- Share Icon Share
Abstract
Chemotherapy, the cornerstone of cancer treatment, although invaluable, is plagued with unbearable and occasionally life-threatening side effects due to its inability to discriminate between tumorous and healthy cells. Anticancer nanomedicines have gained prominence due to their site-specific delivery of chemotherapeutic agents. In comparison to traditional chemical and physical procedures, which add to the chemical burden of an already ailing body, biosynthesis of nanomaterials by plants and microorganisms has evolved as safer ‘green’ nano-manufacturing technology. While nanomedicines from plant extracts have been exhaustively researched, the use of microbes as potential nano factories for the production of metal nanoparticles has recently piqued interest. Many bacteria develop defence mechanisms to detoxify hazardous metal ions, which results in formation of nano scaled metals that can be used for numerous therapeutic applications. The intrinsic variability of microbiological systems, however, poses its own set of challenges, necessitating more stringent standardization protocols in order to create nanomaterials with reproducible attributes. In this paper, we review the emerging trends in the green biosynthesis of nanomaterials and their potential applicability in cancer therapeutics. We probe the microbial biosynthetic mechanistic pathways and the efforts taken to control the physicochemical characteristics of nanoparticles. The applications of metallic nanoparticles obtained from microbes as well as polymeric systems obtained from bacteria, fungi and seaweed in oncology are described in detail. The development of these nanomaterials as next-generation green anticancer drugs may result in a revolution in cancer therapeutics.
Introduction
Cancer is a deadly disease causing the death of nearly 10 million people every year, with approximately 75% of these fatalities being reported from low and middle-income countries (Soerjomataram and Bray 2021). Emerging paradigms in nanotechnology have resulted in major advances in the detection, diagnosis, and treatment of cancers (Yesilot and Aydin 2019). Nanoparticles are solid materials of approximately 1–100 nm in size. They have distinctive properties, which make them useful in focused drug delivery and therapeutics (Sriram et al. 2012). Targeted therapies are of utmost importance in cancer treatment as they cause fewer unwanted side effects, need lesser doses, and are less susceptible to the development of drug resistance (Aghebati‐Maleki et al. 2020). Nanoparticulate drug delivery systems have been shown to exhibit improved anticancer effects in comparison to the therapeutic entities they contain. This is due to their enhanced pharmacokinetics and pharmacodynamics, and active intracellular delivery of the drug resulting in more specific targeting to tumour tissues (Davis et al. 2008).
Nanomedicine in cancer theranostics
The domain of nanotechnology has undergone explosive growth in biomedical sciences. Nanomedicines have been extensively explored for the diagnosis and treatment of several diseases such as diabetes, cancer, bacterial infections, cardiovascular and neurological diseases. Different nanoparticulate agents such as metal nanoparticles (Kuchur et al. 2020), liposomal nanoparticles (Mehta et al. 2021), protein nanoparticles (Miao et al. 2022), lipid nanoparticles (Bukhari et al. 2021) and dendrimers (Gao Mingwu Shen Xiangyang Shi et al. 2021) have given better results than conventional therapeutic strategies. Nanoparticles offer several advantages such as small size, multivalency, ease of functionalization, enhanced drug loading, easy penetration, and improved retention inside target tissue, which has resulted in improved diagnostics and therapeutics of various cancers (Gavas et al. 2021, Woythe et al. 2021). In addition, excellent biocompatibility and biodegradability have increased their application in bio-imaging, bio-sensing, diagnostics, and therapeutics (Madamsettyet al. 2019). The excellent drug likeness bestowed by these systems has resulted in a significant number of regulatory approvals for anticancer nanotherapeutic drugs. Shan et al. has recently published an exhaustive review on the clinical application of nanomedicines in various diseases, including cancer (Shan et al. 2022). A few notable examples are enlisted in Table 1.
Name . | Company . | Drug . | Nano formulation . | Cancer . | Reference . |
---|---|---|---|---|---|
Doxil® | Janssen | Doxorubicin | Liposome-PEG | Metastatic breast cancer, metastatic ovarian cancer | (James et al. 1994) |
Eligard® | Tolmar | Leuprolide acetate | PLGA | Prostate cancer | (Berges 2005) |
Abraxane® | Celgene | Paclitaxel | Albumin | Metastatic breast cancer Pancreatic cancer | (Berges 2005) |
Cynviloq | Sorrento Therapeutics, Inc. | Paclitaxel | PEG-PDLLA block copolymer | Metastatic breast cancer | (Houdaihed et al. 2017) |
Nanoxel | Samyang Biopharmaceuticals Corporation | Docitaxel | PEG-PDLLA block copolymer | Metastatic breast cancer | (Houdaihed et al. 2017) |
Genexol PM® | Samyang Corporation | Paclitaxel | mPEG-PLA | Metastatic breast cancer | (Ventola 2017) |
Onivyde® | Merrimack | Irinotecan | Liposome | Pancreatic cancer | (Oncology Times 2015) |
Name . | Company . | Drug . | Nano formulation . | Cancer . | Reference . |
---|---|---|---|---|---|
Doxil® | Janssen | Doxorubicin | Liposome-PEG | Metastatic breast cancer, metastatic ovarian cancer | (James et al. 1994) |
Eligard® | Tolmar | Leuprolide acetate | PLGA | Prostate cancer | (Berges 2005) |
Abraxane® | Celgene | Paclitaxel | Albumin | Metastatic breast cancer Pancreatic cancer | (Berges 2005) |
Cynviloq | Sorrento Therapeutics, Inc. | Paclitaxel | PEG-PDLLA block copolymer | Metastatic breast cancer | (Houdaihed et al. 2017) |
Nanoxel | Samyang Biopharmaceuticals Corporation | Docitaxel | PEG-PDLLA block copolymer | Metastatic breast cancer | (Houdaihed et al. 2017) |
Genexol PM® | Samyang Corporation | Paclitaxel | mPEG-PLA | Metastatic breast cancer | (Ventola 2017) |
Onivyde® | Merrimack | Irinotecan | Liposome | Pancreatic cancer | (Oncology Times 2015) |
Name . | Company . | Drug . | Nano formulation . | Cancer . | Reference . |
---|---|---|---|---|---|
Doxil® | Janssen | Doxorubicin | Liposome-PEG | Metastatic breast cancer, metastatic ovarian cancer | (James et al. 1994) |
Eligard® | Tolmar | Leuprolide acetate | PLGA | Prostate cancer | (Berges 2005) |
Abraxane® | Celgene | Paclitaxel | Albumin | Metastatic breast cancer Pancreatic cancer | (Berges 2005) |
Cynviloq | Sorrento Therapeutics, Inc. | Paclitaxel | PEG-PDLLA block copolymer | Metastatic breast cancer | (Houdaihed et al. 2017) |
Nanoxel | Samyang Biopharmaceuticals Corporation | Docitaxel | PEG-PDLLA block copolymer | Metastatic breast cancer | (Houdaihed et al. 2017) |
Genexol PM® | Samyang Corporation | Paclitaxel | mPEG-PLA | Metastatic breast cancer | (Ventola 2017) |
Onivyde® | Merrimack | Irinotecan | Liposome | Pancreatic cancer | (Oncology Times 2015) |
Name . | Company . | Drug . | Nano formulation . | Cancer . | Reference . |
---|---|---|---|---|---|
Doxil® | Janssen | Doxorubicin | Liposome-PEG | Metastatic breast cancer, metastatic ovarian cancer | (James et al. 1994) |
Eligard® | Tolmar | Leuprolide acetate | PLGA | Prostate cancer | (Berges 2005) |
Abraxane® | Celgene | Paclitaxel | Albumin | Metastatic breast cancer Pancreatic cancer | (Berges 2005) |
Cynviloq | Sorrento Therapeutics, Inc. | Paclitaxel | PEG-PDLLA block copolymer | Metastatic breast cancer | (Houdaihed et al. 2017) |
Nanoxel | Samyang Biopharmaceuticals Corporation | Docitaxel | PEG-PDLLA block copolymer | Metastatic breast cancer | (Houdaihed et al. 2017) |
Genexol PM® | Samyang Corporation | Paclitaxel | mPEG-PLA | Metastatic breast cancer | (Ventola 2017) |
Onivyde® | Merrimack | Irinotecan | Liposome | Pancreatic cancer | (Oncology Times 2015) |
Nanoparticulate delivery systems in combination with agents used in chemotherapeutics and radiotherapeutics are being used in the treatment of various cancers (Laprise-Pelletier et al. 2018). Chemotherapeutical nanoparticulate formulations have several advantages over conventional chemotherapy dosage forms such as enhanced bioavailability and decreased metabolism and elimination through hepatic and renal routes (Matsumura 2008). Besides this, increased permeability and selective accumulation of chemotherapeutical nanoparticles inside the tumour tissue results in low systemic toxicity and higher efficiency of treatment as compared to treatment with standard chemotherapy (El-Readi and Althubiti 2019).
The development of multi-functional particle systems is another advantageous attribute of nanomaterial techniques, where the therapeutic ligands can be bundled along with imaging agents to develop a drug delivery system of a single functionalized nanoparticle and used for selective imaging and therapeutics (Fan et al. 2014). Multi-functional nanoplatforms that combine both therapeutic components and multimodality imaging can deliver therapeutic agents and concomitantly monitor the therapy response in real-time, thereby permitting customized dosing. Such non-invasive imaging techniques have been used to quantitatively monitor the drug delivery processes. Imaging of tumours and other lesions has also been explored using radiolabelled nanomaterials. Some examples of inorganic nanoparticles which have been used for developing multifunctional theranostic platforms are gold, silver, and silica-based nanomaterials (Karantas 2021).
Nanoparticles are synthesized by a variety of physical, chemical, and biological methods (Mohanpuria et al. 2008). The physical methods such as grinding, milling, and thermal ablation are more resource exhaustive and require high amounts of source energy while giving lower production yields of the nanoscale materials (Shedbalkar et al. 2014). Chemical methods such as electrochemical techniques, and chemical and photochemical reduction have gained preference over the last few years because of lower energy requirements during the reduction step and homogeneity of the particles in terms of size and shape (Albanese et al. 2012). However, these methods necessitate the use of various toxic substances such as hydrazine and potassium bitartrate which may cause genotoxicity, carcinogenicity, and cytotoxicity (Gahlawat and Choudhury 2019). This greatly limits the clinical applicability of these physical and chemical methods. Biosynthesis of nanoparticles using various green approaches involving plants and microorganisms have evolved as non-hazardous, eco-friendly, and economical approaches to overcome the drawbacks of the traditional methods of synthesis (Singh et al. 2016). This review showcases some of the green metallic and polymeric nanoparticle systems, which have been developed for cancer therapy. Nanomedicine developed from natural sources such as plants and microbes are inherently capable of overcoming some of the limitations of those obtained by physical and chemical methods. Besides their own integral anticancer mechanisms of action, they have shown excellent potential for targeted delivery of chemotherapeutic drugs along with additional attributes of good bioavailability and safety.
Green metallic nanoparticles for cancer therapeutics
Various plants and microorganisms such as bacteria, actinomycetes, fungi, algae, and yeast have been exploited to produce metallic nanoparticles with a broad range of sizes, shapes, compositions, and physicochemical properties (Begum et al. 2022, Jeevanandam et al. 2022). This green approach is scalable and enables the synthesis of nanoparticles in aqueous media with low cost and energy requirements (Wang et al. 2021).
Metallic nanoparticles synthesized using plants
Semisynthetic approaches for nanoparticle synthesis using plants have been widely reported (Das et al. 2017, Al-Radadi 2022, Habeeb Rahuman et al. 2022). Metal salt solutions are processed along with purified plant extracts to obtain metallic nanoparticles of well-defined geometries. The presence of different kinds of phytoconstituents in the extracts such as flavonoids, alkaloids, phenolics and polysaccharides as well as secondary metabolites aid in the bio-reductive synthesis and stabilization of the metal nanoparticles (Marslin et al. 2018, Hano and Abbasi 2022). The morphology of the nanoparticles can be fine-tuned by appropriate control of reaction conditions such as temperature, time, pH, sand salt concentration (Zhang et al. 2020). Numerous excellent reviews have been published on plant-derived metal nanoparticles and their cytotoxic potential for cancer therapy. Table 2 lists some recent research work of green synthesis of anticancer metal nanoparticles processed using plant extracts.
Anticancer activity of metal nanoparticles synthesized using plant extracts.
Plant . | Extract . | Nano Particle . | Cancer Cell line . | Anticancer potential . | Reference . |
---|---|---|---|---|---|
Brazilian Red Propolis | Hydroethanolic extract | Gold | T24, PC-3 | Bladder, Prostate | (Botteon et al. 2021) |
Benincasa hispida | Aqueous extract | Gold | HeLa | Cervical | (Saqr et al. 2021) |
Trachyspermum ammi | Seed extract | Gold | HepG2, MCF-7 | Liver, Breast | (Perveen et al. 2021) |
Orchid | Whole plant extract | Gold | AMJ 13 | Breast cancer | (Yas et al. 2021) |
Argemone mexicanaL. | Aqueous extract | Gold | HCT-15 | Colon | (Datkhile et al. 2021) |
Nigella sativa | Ethanolic leaf extract | Gold | HepG2, MCF-7 | Liver | (Gothainayagi and Josephine 2020) |
Walnut green | Shell extract | Gold | MCF7 | Breast | (Rabori et al. 2021) |
Crassocephalum rubens | Aqueous leaf extract | Gold | MCF-7, Caco-2 | Breast, Colorectal | (Adewale et al. 2020) |
Backhousia citriodora | Aqueous leaf extract | Gold | MCF-7, HepG2 | Breast, Liver | (El-Borady et al. 2020) |
Endophytic Cladosporium | Leaf extract | Gold | MCF-7 | Breast cancer | (Munawer et al. 2020) |
Brassica oleracea | Aqueous leaf extract | Silver | MCF-7 | Breast cancer | (Ansar et al. 2020) |
Syzygium aromaticum | Fruit | Silver | A549 | Lung cancer | (Venugopal et al. 2017) |
Phaseolus vulgaris Calotropis | Seed | Cu/CuO | HeLa | Cervical Cancer | (Nagajyothi et al. 2017) |
Azadirachta indica | Leaf | Cu/CuO | HeLa, MCF-7 | Cervical Cancer, Breast cancer | (Rehana et al. 2017) |
Acalypha indica | Leaf | Cu/CuO | MCF-7 | Breast cancer | (Nisar et al. 2019) |
Alchornea cordifolia Cervical | Leaf extract | Cu2O/CuO–ZnO | HeLa | Cervical | (Elemike et al. 2020) |
Deverra tortuosa | Aqueous extract of aerial parts | ZnO | Caco-2 | Human colon adenocarcinoma | (Selim et al. 2020) |
Bambusa arundinacea | Hydroalcoholic leaf extract | ZnO | MCF-7 | Breast cancer | (Jayarambabu et al. 2021) |
Tea | Ethanolic extract | ZnO + Chitosan + Paclitaxel | MCF-7 | Breast cancer | (Akbarian et al. 2020) |
Lawsonia inermis | Aqueous leaf extract | Zinc ferrite | MCF-7 | Breast cancer | (Sarala et al. 2020) |
Plant . | Extract . | Nano Particle . | Cancer Cell line . | Anticancer potential . | Reference . |
---|---|---|---|---|---|
Brazilian Red Propolis | Hydroethanolic extract | Gold | T24, PC-3 | Bladder, Prostate | (Botteon et al. 2021) |
Benincasa hispida | Aqueous extract | Gold | HeLa | Cervical | (Saqr et al. 2021) |
Trachyspermum ammi | Seed extract | Gold | HepG2, MCF-7 | Liver, Breast | (Perveen et al. 2021) |
Orchid | Whole plant extract | Gold | AMJ 13 | Breast cancer | (Yas et al. 2021) |
Argemone mexicanaL. | Aqueous extract | Gold | HCT-15 | Colon | (Datkhile et al. 2021) |
Nigella sativa | Ethanolic leaf extract | Gold | HepG2, MCF-7 | Liver | (Gothainayagi and Josephine 2020) |
Walnut green | Shell extract | Gold | MCF7 | Breast | (Rabori et al. 2021) |
Crassocephalum rubens | Aqueous leaf extract | Gold | MCF-7, Caco-2 | Breast, Colorectal | (Adewale et al. 2020) |
Backhousia citriodora | Aqueous leaf extract | Gold | MCF-7, HepG2 | Breast, Liver | (El-Borady et al. 2020) |
Endophytic Cladosporium | Leaf extract | Gold | MCF-7 | Breast cancer | (Munawer et al. 2020) |
Brassica oleracea | Aqueous leaf extract | Silver | MCF-7 | Breast cancer | (Ansar et al. 2020) |
Syzygium aromaticum | Fruit | Silver | A549 | Lung cancer | (Venugopal et al. 2017) |
Phaseolus vulgaris Calotropis | Seed | Cu/CuO | HeLa | Cervical Cancer | (Nagajyothi et al. 2017) |
Azadirachta indica | Leaf | Cu/CuO | HeLa, MCF-7 | Cervical Cancer, Breast cancer | (Rehana et al. 2017) |
Acalypha indica | Leaf | Cu/CuO | MCF-7 | Breast cancer | (Nisar et al. 2019) |
Alchornea cordifolia Cervical | Leaf extract | Cu2O/CuO–ZnO | HeLa | Cervical | (Elemike et al. 2020) |
Deverra tortuosa | Aqueous extract of aerial parts | ZnO | Caco-2 | Human colon adenocarcinoma | (Selim et al. 2020) |
Bambusa arundinacea | Hydroalcoholic leaf extract | ZnO | MCF-7 | Breast cancer | (Jayarambabu et al. 2021) |
Tea | Ethanolic extract | ZnO + Chitosan + Paclitaxel | MCF-7 | Breast cancer | (Akbarian et al. 2020) |
Lawsonia inermis | Aqueous leaf extract | Zinc ferrite | MCF-7 | Breast cancer | (Sarala et al. 2020) |
Anticancer activity of metal nanoparticles synthesized using plant extracts.
Plant . | Extract . | Nano Particle . | Cancer Cell line . | Anticancer potential . | Reference . |
---|---|---|---|---|---|
Brazilian Red Propolis | Hydroethanolic extract | Gold | T24, PC-3 | Bladder, Prostate | (Botteon et al. 2021) |
Benincasa hispida | Aqueous extract | Gold | HeLa | Cervical | (Saqr et al. 2021) |
Trachyspermum ammi | Seed extract | Gold | HepG2, MCF-7 | Liver, Breast | (Perveen et al. 2021) |
Orchid | Whole plant extract | Gold | AMJ 13 | Breast cancer | (Yas et al. 2021) |
Argemone mexicanaL. | Aqueous extract | Gold | HCT-15 | Colon | (Datkhile et al. 2021) |
Nigella sativa | Ethanolic leaf extract | Gold | HepG2, MCF-7 | Liver | (Gothainayagi and Josephine 2020) |
Walnut green | Shell extract | Gold | MCF7 | Breast | (Rabori et al. 2021) |
Crassocephalum rubens | Aqueous leaf extract | Gold | MCF-7, Caco-2 | Breast, Colorectal | (Adewale et al. 2020) |
Backhousia citriodora | Aqueous leaf extract | Gold | MCF-7, HepG2 | Breast, Liver | (El-Borady et al. 2020) |
Endophytic Cladosporium | Leaf extract | Gold | MCF-7 | Breast cancer | (Munawer et al. 2020) |
Brassica oleracea | Aqueous leaf extract | Silver | MCF-7 | Breast cancer | (Ansar et al. 2020) |
Syzygium aromaticum | Fruit | Silver | A549 | Lung cancer | (Venugopal et al. 2017) |
Phaseolus vulgaris Calotropis | Seed | Cu/CuO | HeLa | Cervical Cancer | (Nagajyothi et al. 2017) |
Azadirachta indica | Leaf | Cu/CuO | HeLa, MCF-7 | Cervical Cancer, Breast cancer | (Rehana et al. 2017) |
Acalypha indica | Leaf | Cu/CuO | MCF-7 | Breast cancer | (Nisar et al. 2019) |
Alchornea cordifolia Cervical | Leaf extract | Cu2O/CuO–ZnO | HeLa | Cervical | (Elemike et al. 2020) |
Deverra tortuosa | Aqueous extract of aerial parts | ZnO | Caco-2 | Human colon adenocarcinoma | (Selim et al. 2020) |
Bambusa arundinacea | Hydroalcoholic leaf extract | ZnO | MCF-7 | Breast cancer | (Jayarambabu et al. 2021) |
Tea | Ethanolic extract | ZnO + Chitosan + Paclitaxel | MCF-7 | Breast cancer | (Akbarian et al. 2020) |
Lawsonia inermis | Aqueous leaf extract | Zinc ferrite | MCF-7 | Breast cancer | (Sarala et al. 2020) |
Plant . | Extract . | Nano Particle . | Cancer Cell line . | Anticancer potential . | Reference . |
---|---|---|---|---|---|
Brazilian Red Propolis | Hydroethanolic extract | Gold | T24, PC-3 | Bladder, Prostate | (Botteon et al. 2021) |
Benincasa hispida | Aqueous extract | Gold | HeLa | Cervical | (Saqr et al. 2021) |
Trachyspermum ammi | Seed extract | Gold | HepG2, MCF-7 | Liver, Breast | (Perveen et al. 2021) |
Orchid | Whole plant extract | Gold | AMJ 13 | Breast cancer | (Yas et al. 2021) |
Argemone mexicanaL. | Aqueous extract | Gold | HCT-15 | Colon | (Datkhile et al. 2021) |
Nigella sativa | Ethanolic leaf extract | Gold | HepG2, MCF-7 | Liver | (Gothainayagi and Josephine 2020) |
Walnut green | Shell extract | Gold | MCF7 | Breast | (Rabori et al. 2021) |
Crassocephalum rubens | Aqueous leaf extract | Gold | MCF-7, Caco-2 | Breast, Colorectal | (Adewale et al. 2020) |
Backhousia citriodora | Aqueous leaf extract | Gold | MCF-7, HepG2 | Breast, Liver | (El-Borady et al. 2020) |
Endophytic Cladosporium | Leaf extract | Gold | MCF-7 | Breast cancer | (Munawer et al. 2020) |
Brassica oleracea | Aqueous leaf extract | Silver | MCF-7 | Breast cancer | (Ansar et al. 2020) |
Syzygium aromaticum | Fruit | Silver | A549 | Lung cancer | (Venugopal et al. 2017) |
Phaseolus vulgaris Calotropis | Seed | Cu/CuO | HeLa | Cervical Cancer | (Nagajyothi et al. 2017) |
Azadirachta indica | Leaf | Cu/CuO | HeLa, MCF-7 | Cervical Cancer, Breast cancer | (Rehana et al. 2017) |
Acalypha indica | Leaf | Cu/CuO | MCF-7 | Breast cancer | (Nisar et al. 2019) |
Alchornea cordifolia Cervical | Leaf extract | Cu2O/CuO–ZnO | HeLa | Cervical | (Elemike et al. 2020) |
Deverra tortuosa | Aqueous extract of aerial parts | ZnO | Caco-2 | Human colon adenocarcinoma | (Selim et al. 2020) |
Bambusa arundinacea | Hydroalcoholic leaf extract | ZnO | MCF-7 | Breast cancer | (Jayarambabu et al. 2021) |
Tea | Ethanolic extract | ZnO + Chitosan + Paclitaxel | MCF-7 | Breast cancer | (Akbarian et al. 2020) |
Lawsonia inermis | Aqueous leaf extract | Zinc ferrite | MCF-7 | Breast cancer | (Sarala et al. 2020) |
Green metal oxide nanocomposites for cancer therapy
Nanocomposites are matrix-based materials that are used to incorporate nano-sized entities in order to improve their properties and biological effects. ZnO nanocomposites have been reported to exhibit enhanced biocompatibility as well as ROS generation potential. Ahamed et al. (2021c) synthesized tin oxide-doped ZnO nanoparticles embedded in reduced graphene oxide nanocomposites and found them to be selectively cytotoxic to human breast cancer cells (MCF-7) over human normal breast epithelial cells (MCF10A). In another example, Ahamed et al. (2022) reported a facile, economical method for the synthesis of ZnO-reduced graphene oxide nanocomposites using the extract of garlic clove. The aqueous garlic extract acted as a reducing and stabilizing agent for the ZnO nanocomposites which showed two-fold higher cytotoxic effect in human breast cancer and colorectal cancer cell lines than pure ZnO nanoparticles. Ahamed et al. reported the development of silver/reduced graphene oxide nanocomposites using orange peel extract (Ahamed et al. 2021a). Besides acting as a bio-reductant and stabilizer, the flavonoids present in the orange peel added to the anticancer effects of the preparation. These green nanocomposites showed potent oncogenic potential via ROS-mediated oxidative stress generation and depletion of antioxidant glutathione levels.
Microbes as nano-factories
Green synthesis of microbial nanoparticles using bacteria, fungi, and yeast, has many favourable attributes. Their ease of cultivation and ability to grow rapidly at ambient conditions of temperature, pH, and pressure makes them a useful resource for nanoparticle synthesis. Many microbes produce inorganic nanoparticles in response to toxic metal environments.
Synthesis mechanisms
During metal-stress situations, they resort to several survival mechanisms such as efflux of metal ions through cell membranes and reduction to non-toxic forms in order to eliminate the heavy toxic metals (Issazadeh et al. 2013). Microbes have the inherent ability to trap these metal ions from the surrounding environment by various mechanisms such as endocytosis, use of ion pumps and carrier-mediated transport, lipid permeation, and siderophore-mediated absorption (Venkat Kumar et al. 2017). The detoxification mechanism itself involves vacuole compartmentalization and metal binding. Molecules like glutathione or metallothionines isolated from Pseudomonas putida, Cyanobacteria, and Escherichia coli have been documented to be involved in metal detoxification (Menon et al. 2017).
Microbial synthesis routes
Microbial synthesis of nanoparticles takes place via intracellular or extracellular routes, as depicted in Fig. 1. The production of nanoparticles by the intracellular route involves pelletization of the microbial biomass by centrifugation and its subsequent cultivation with an aqueous solution of the metals under specific incubation conditions. During this process, positively charged metal ions are transported inside the microbial cell via carrier-mediated transport and electrostatic interactions through the negatively charged cell wall and undergo enzymatic bioreduction from toxic to nontoxic metal nanoparticles (Kalabegishvili et al. 2012).
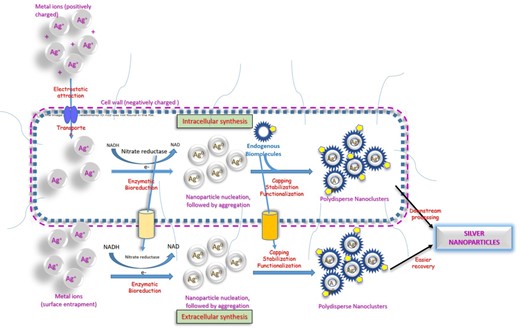
Schematic representation of intracellular and extracellular pathways of microbial synthesis of nanoparticles.
The formation of nanoparticles manifests as chromatic change of solution colour. This helps in monitoring the reaction process and optimizing the manufacturing parameters. Waghmare et al.developed a method for the intracellular production of silver nanoparticles using Candida utilis and optimized the reaction temperature and incubation time using uv-visible spectroscopic monitoring for appearance of intense brown colour that signified the formation of silver nanoparticles (Waghmare et al. 2015).
Actinomycetes belonging to the Rhodococci and Thermomonospora genus have been used for efficient intracellular synthesis of gold nanoparticles from aqueous solutions of AuCl4 ions (Ahmad et al.2003a), whereas fungi such as Verticillium have been used for the production of silver nanoparticles as well as gold nanoparticles (Mukherjee et al. 2001). Silver nanoparticles were reported to be formed in the bacterial periplasmic space when Pseudomonas stutzeri was exposed to concentrated silver nitrate (Klaus et al. 1999). Intracellular enzyme-mediated synthesis of gold nanoparticles has been reported to occur after treatment of various bacteria like Plectonema boryanum (Lengke et al. 2006), Phanerochaete chrysosporium (Sanghi et al. 2011), Shewanella algae (Konishi et al. 2007), and Brevibacterium casei (Kalishwaralal et al. 2010) with inorganic gold salt solutions.
In the extracellular method, the metal ions are trapped on the surface of the cells and converted into metallic nanoparticles in response to enzymes such as nitrate reductase or hydroquinone synthesized by many fungi or prokaryotic organisms (Kalabegishvili et al. 2012) Gold nanoparticles are synthesized from Rhodomonas capsulate by this mechanism (Khandel and Shahi 2016). These enzymes, facilitated by their associated cofactors such as NADH and NADPH act as electron carriers (Bose and Chatterjee 2016) and catalyze the bioreduction of metal ions to metal nanoparticles. Gold nanoparticles have been obtained via electron-mediated reduction by NADH-dependent enzymes secreted by Rhodopseudomonas capsulate (He et al. 2007). Fungal nitrate reductase-mediated reduction of silver nitrate is another useful extracellular method that has been exploited for the production of silver nanoparticles (Ingle et al. 2008). Fusarium oxysporum (Durán et al. 2005),Cladosporium cladosporioides, and Coriolus versicolor (Balaji et al. 2009),Aspergillus niger (Gade et al. 2008),Aspergillus fumigatus (Bhainsa and D'Souza 2006),Penicillium brevicompactum (Shaligram et al. 2011) have been successfully employed to produce metal nanoparticles by the extracellular method.
Optimization parameters
The topographical properties of nanoparticles such as size, shape, and mono-dispersity influence their biological activity (Kladko et al. 2021). Microbial synthesis of nanoparticles is often plagued by low production rates and poor dispersibility of the obtained particles. Various parameters, including selection of microorganisms, growth medium, and synthesis conditions influence the morphology of the nanoparticles and need careful optimization (Narayanan and Sakthivel 2010). Well-dispersed, small, spherical, silver nanoparticles were obtained from Fusarium oxysporum after extensive consideration of several reaction conditions. The optimal parameters were established as temperature of 50°C, pH of 6, incubation time of 72 hours, metal ion concentration to 10 mM silver nitrate and fungal age of 7 days (Husseiny et al. 2015). The concentration of silver ions was the most significant parameter for obtaining silver nanoparticles from Klebsiella pneumonia, with 1 mM solution giving the best results (Mokhtari et al. 2009). A direct correlation was found between the size of silver nanoparticles and pH of the medium (Kathiresan et al. 2009) when obtained from Penicillium fellutanum. Silver ion concentration was found to influence the size and dispersion of nanoparticles from Hormoconis resinae (Varshney et al. 2009), while temperature was found to influence the morphology and sizes of nanoparticles synthesized using cyanobacteria (Lengke et al. 2007). Different precursor silver ions solutions gave different sized nanoparticles (Mokhtari et al. 2009), while the incubation time had a direct effect on the monodispersity as well as the particle size of gold nanoparticles (Gericke and Pinches 2006b). The growth phase of microorganisms also had a direct influence over the nanoparticle yield, with cells harvested during the early exponential phase producing a better yield than those obtained from the late exponential phase. This could be because of higher concentrations of enzymes and proteins being available for the uptake and reduction of metal ions (Gericke and Pinches 2006b).
Various biophysical methods such as X-ray diffraction, scanning electron microscopy, transmission electron microscopy and Fourier transform infrared spectroscopy are used to characterize the nanoparticles obtained from microbial biosynthesis (Jeevanet al. 2012).
Scalability
The scalability of the biosynthetic methods must be given due consideration before finalizing the production protocol. In comparison to synthetic approaches, microbial methods produce medium to low yield of nanoparticles, but the abundant availability and ease of growth of the source microbes, minimal requirements of reagents and solvents, and simple constraints of reaction conditions more than offsets the costs of production (Mukherjee and Patra 2016). A distinct advantage of the microbial method over chemical synthesis is its ability to produce highly stable metal nanoparticles that do not agglomerate for long periods due to the in situ capping of the nanoparticles by microbial proteins or biomolecules (Balakrishnan et al. 2017).
Several researchers have demonstrated the use of bacteria in metal ion reduction. The primary advantages of bacteria-mediated production are its sustainability, adaptability to scaling up, and less involvement of toxic chemicals. However, the bacterial culturing processes can be labour-intensive and allow limited control over the physicochemical properties of the nanoparticles. Fungi are another valuable resource that are capable of intracellular and extracellular nanoparticle synthesis and produces nanoparticles with well-defined geometries. In addition, higher yields are obtained as compared to bacteria due to the larger biomass of fungi. However, fungi-mediated production is time-consuming and necessitates costly down-streaming processing which presents challenges for commercial-scale production of nanoparticles (Fariq et al. 2017). Adaptation of microalgae and cyanobacteria can be beneficial for economical nanoparticle synthesis as their photoautotrophic metabolism needs only carbon dioxide, light, inorganic nutrients and water for survival, which lowers the cost of production (Kuznetsov et al. 2011).
Biosynthetic strategy selection is another aspect which influences the large-scale production quality of nanoparticles. Extracellular routes of production are amenable to multiple cycles of microorganism usage and generally yield more polydisperse nanoparticles (Feng et al. 2019). In addition, this approach involves simpler downstream processing and purification in comparison to intracellular routes (Baro et al. 2012).
Anticancer mechanisms
Metal nanoparticles of biosynthetic origin have been found to induce cytotoxicity to tumour cells via multiple mechanisms, which have been compiled in Fig. 2.
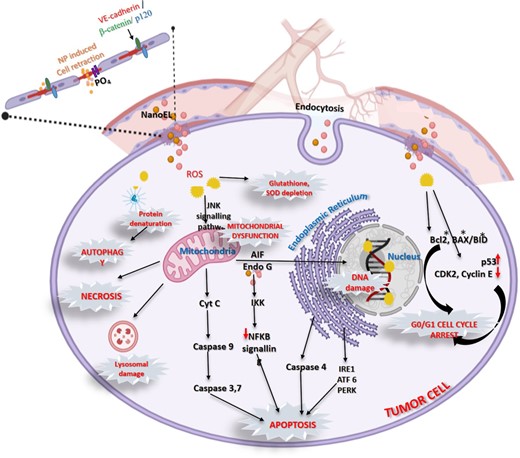
Schematic representation of anticancer mechanisms of metallic nanoparticles.
Cellular internalization
The primary routes of uptake of nanoparticles by tumour cells involve nanoparticle induced endothelial leakiness (NanoEL) (Setyawatiet al. 2013, Ni et al. 2022) that help the nanoparticles to leave the systemic circulation and endocytosis that helps in uptake of the nanoparticles into the tumour cells (Yang et al. 2019).
NanoEL
NanoEL refers to the tendency of certain nanoparticles to widen the gaps between endothelial cells in specific tissues, thereby allowing them to leave the systemic vasculature at the tumour site. Endothelial cells from continuous layers, connected at their extracellular junctions by stable vascular endothelial cadherin proteins. The intracellular ends of cadherin form ternary complexes with other proteins such as β-catenin, and p120 that help to stabilize the intercellular junction. Certain nanoparticles are able to move into adherens junction due to their small sizes and attach themselves to the cadherin molecules at their extracellular ends and disrupt the interaction between neighbouring endothelial cells. This triggers phosphorylation of multiple residues at the intracellular ends of cadherin with subsequent loss of interactions with β-catenin, and p120. This destabilization leads to remodeling of actin and retraction of the endothelial cells. The resultant micrometer-sized gaps between cells causes increased vascular permeability and leakiness, permitting nanoparticles to overcome the endothelial barrier and leave the systemic circulation near certain tissues including tumours (Setyawati et al. 2017, Peng et al. 2019) dysfunction (Jain et al. 2021).
Endocytosis
Nanoparticles in close vicinity of tumour cells form various interactions with their cell membranes depending on their size, shape, stiffness, surface charge, and other physicochemical properties. This induces membrane wrapping of the nanoparticles and uptake into the cells by different types of endocytosis such as phagocytosis, macropinocytosis, clathrin-dependent and caveolin-dependent endocytosis (Zhang et al. 2015). Phagocytosis and micropinocytosis cause internalisation of nanoparticles through polymerization of actin molecules that induce membrane ruffling, invagination of the cellular membrane to form a pocket which then pinches off with the embedded nanoparticles (Kim and Choi 2012). Opsonization by immunoglobulins aid in cellular recognition by phagocytes. Endocytosis can be mediated by clathrin which forms lattice coats around the endocytosed vesicles followed by scission (Sousa De Almeida et al. 2021) or caveolin proteins which form association with lipid molecules like cholesterol to induce spontaneous membrane surface curvature and budding and vesicle formation (Sousa De Almeida et al. 2021). Caveolin-aided penetration and receptor mediated endocytosis via cellular proteins like clathrin have been reported for charged and neutral nanoparticles (Harush-Frenkel et al. 2007). Various endocytic strategies lead to engulfment of the nanoparticles and their cleaving off to form endocytic vesicles for transport into the cells.
Oxidative stress leading to ROS-mediated mitochondrial dysfunction
Inside the tumour cells, nanoparticles induce the generation of excessive amounts of ROS that cause oxidative damage to proteins, lipids (Ahamed et al. 2017) and DNA (Ahamed et al. 2021b) and ultimately mitochondrial dysfunction leading to apoptosis, autophagy and necrosis (Elmore 2007).
Apoptosis pathways
High levels of intracellular ROS in the mitochondria affects the integrity of its membrane, leading to activation of the C-Jun N-terminal kinase (JNK) pathway and subsequent release of cytosolic cytochrome c, which triggers the intrinsic caspase apoptosis cascade (Ahamed et al. 2021b). Additionally, activation of ER stress-related proteins such as IRE1 (inositol-requiring protein-1), ATF-6 (activating transcription factor-6), and PERK (protein kinase RNA (PKR)-like ER kinase in the endoplasmic reticulum further supplements apoptosis (Noël et al. 2016). A third effect of nanoparticle mediated damage is disruption of the NF-κB signal transduction pathway due to their interaction with the cysteine residues of IκB kinases (IKK), which are the key components of this pathway that regulates cancer progression (Rónavári et al. 2021).
Downregulation of antiapoptotic transcription factors: elevated ROS also exhausts the levels of cellular antioxidants, glutathione, and superoxide dismutase, further exaggerating oxidative damage in tumour cells (Ahamed et al. 2019).
Cell cycle arrest
Metallic nanoparticles have also been reported to induce the upregulation of genes such as BID, BAX/BCl2, which lead to cell cycle arrest in the G0/G1 phases which lead to cell death (Abdel-Ghany et al. 2020).
Autophagy
Organelle stress due to aggregation of the damaged proteins negatively affects the gene expression and enzyme-mediated regulatory pathways, thereby triggering autophagy pathways (Sun et al. 2012).
Gold nanoparticles
The medicinal uses of gold and its compounds have been demonstrated for a variety of ailments such as cancer, rheumatic diseases, discoid lupus erythematosus (Shedbalkar et al. 2014), and inflammatory skin disorders such as pemphigus, urticaria, and psoriasis (Fricker 1996). The unique optical and physicochemical properties and biocompatibility of gold nanoparticles have resulted in their being explored for tumour targeting, imaging, and photothermal therapy of cancer (Verma et al. 2019). Conventional methods of synthesis of gold nanoparticles by physical and chemical methods, although extensively studied, are plagued by various drawbacks such as the need for harsh chemicals, restrictive reaction conditions, exhaustive energy requirements (Marsili et al. 2008), moderate productivity (Riddin et al. 2006), expensive purification procedures (Murphy 2002) and environmental hazards due to toxic solvents and additives. Microbial synthesis of gold nanoparticles provides an environmentally benign, economical, scalable, and sustainable alternative for the development of gold nanoparticles (Narayanan and Sakthivel 2010, Lee and Nagajyothi 2011). Biosynthesis of gold nanoparticles can be accomplished in shorter timespans than chemical synthesis and does not require external stabilization as the biogenic components of microbes act as stabilizing as well as capping agents. The entire process including the functionalization for biological activation of the gold nanoparticles requires a lesser total number of reaction steps as compared to chemical synthesis. In addition, these biogenic nanoparticles do not show the presence of toxic by-products that contaminate the nanoparticles during chemical synthesis (Singh et al. 2016).
The biosynthesis of gold nanoparticles by different microorganisms via both intracellular and extracellular methods has been reported (Nangia et al. 2009, Suresh et al. 2011). The extracellular method facilitates easier recovery of the nanoparticles and is preferred (Sharma et al. 2007).
Gold nanoparticles are produced by a host of microorganisms. The first step in the synthesis is the microbial enzyme-catalyzed reduction of Au3+ ions, leading to nanoparticle nucleation and growth (Durán et al. 2005). Amino acid residues in microbial enzyme proteins influence the biosynthesis, capping, and stabilization of the gold nanoparticles by either donating electrons to the gold metal, thereby converting it to the reduced state, or binding to the nanoparticles leading to their stabilization (Si and Mandal 2007). The rate of particle formation and consequently the size of the nanoparticles is reported to be influenced by the operating parameters such as temperature and pH. Various bacteria, fungi, actinomycetes, yeast, algae, and plants (Mourato et al. 2011) are known to accumulate gold ions from solution. The synthesis of gold nanoparticles using various organisms such as Bacillus subtilis. (Satyanarayana Reddy et al. 2010), fungal species such as Verticillium and Fusarium (Ahmad et al. 2003b), actinomycete such as Rhodococcus and Thermomonospora (Ahmadet al. 2003a) and lactic acid bacteria (Nair and Pradeep 2002) have been documented.
Mechanisms of NP production
Several diverse mechanisms and reductive strategies for the production of gold nanoparticles have been detected in different species of microorganisms. Table 3 highlights some of the mechanisms utilized by various microorganisms for producing gold nanoparticles.
Reductive strategies used by microorganisms for production of gold nanoparticles.
Microorganism . | Mechanism of NP production . |
---|---|
Shewanella algae | Anaerobic hydrogen gas-mediated reduction of Au3+ ions |
E. coli | Periplasmic hydrogenase-mediated reduction of Au3+ ions |
Desulfovibrio desulfuricans | Periplasmic hydrogenase-mediated reduction of Au3+ ions |
Plectonema boryanum | Temperature-dependent precipitation of amorphous Au(I)S NP from aqueous AuCl |
F. oxysporum | NADH-dependent reductase-mediated reduction of Au3+ ions |
R. capsulata | NADH-dependent reductase-mediated reduction of Au3+ ions |
Verticillum species | Lysine amino acid-mediated entrapment and reduction of charged Au |
Phanerochaete chrysosporium | Extracellular laccase enzyme-mediated entrapment and reduction to Au NP |
Phanerochaete chrysosporium | Intracellular ligninase enzyme-mediated entrapment and reduction to Au NP |
Microorganism . | Mechanism of NP production . |
---|---|
Shewanella algae | Anaerobic hydrogen gas-mediated reduction of Au3+ ions |
E. coli | Periplasmic hydrogenase-mediated reduction of Au3+ ions |
Desulfovibrio desulfuricans | Periplasmic hydrogenase-mediated reduction of Au3+ ions |
Plectonema boryanum | Temperature-dependent precipitation of amorphous Au(I)S NP from aqueous AuCl |
F. oxysporum | NADH-dependent reductase-mediated reduction of Au3+ ions |
R. capsulata | NADH-dependent reductase-mediated reduction of Au3+ ions |
Verticillum species | Lysine amino acid-mediated entrapment and reduction of charged Au |
Phanerochaete chrysosporium | Extracellular laccase enzyme-mediated entrapment and reduction to Au NP |
Phanerochaete chrysosporium | Intracellular ligninase enzyme-mediated entrapment and reduction to Au NP |
Reductive strategies used by microorganisms for production of gold nanoparticles.
Microorganism . | Mechanism of NP production . |
---|---|
Shewanella algae | Anaerobic hydrogen gas-mediated reduction of Au3+ ions |
E. coli | Periplasmic hydrogenase-mediated reduction of Au3+ ions |
Desulfovibrio desulfuricans | Periplasmic hydrogenase-mediated reduction of Au3+ ions |
Plectonema boryanum | Temperature-dependent precipitation of amorphous Au(I)S NP from aqueous AuCl |
F. oxysporum | NADH-dependent reductase-mediated reduction of Au3+ ions |
R. capsulata | NADH-dependent reductase-mediated reduction of Au3+ ions |
Verticillum species | Lysine amino acid-mediated entrapment and reduction of charged Au |
Phanerochaete chrysosporium | Extracellular laccase enzyme-mediated entrapment and reduction to Au NP |
Phanerochaete chrysosporium | Intracellular ligninase enzyme-mediated entrapment and reduction to Au NP |
Microorganism . | Mechanism of NP production . |
---|---|
Shewanella algae | Anaerobic hydrogen gas-mediated reduction of Au3+ ions |
E. coli | Periplasmic hydrogenase-mediated reduction of Au3+ ions |
Desulfovibrio desulfuricans | Periplasmic hydrogenase-mediated reduction of Au3+ ions |
Plectonema boryanum | Temperature-dependent precipitation of amorphous Au(I)S NP from aqueous AuCl |
F. oxysporum | NADH-dependent reductase-mediated reduction of Au3+ ions |
R. capsulata | NADH-dependent reductase-mediated reduction of Au3+ ions |
Verticillum species | Lysine amino acid-mediated entrapment and reduction of charged Au |
Phanerochaete chrysosporium | Extracellular laccase enzyme-mediated entrapment and reduction to Au NP |
Phanerochaete chrysosporium | Intracellular ligninase enzyme-mediated entrapment and reduction to Au NP |
Shewanella algae utilize hydrogen gas in an anaerobic environment to reduce Au3+ ions forming 10–20 nm gold nanoparticles (Iravani 2014). Escherichia coli and Desulfovibrio desulfuricans use hydrogen gas in presence of periplasmic hydrogenases to effect the reduction of Au3+ ions (Deplanche and Macaskie 2008). Filamentous cyanobacterium, Plectonema boryanum UTEX 485 interacts with aqueous gold chloride and precipitates amorphous gold (I) sulfide nanoparticles at the cell walls and subsequently deposits metallic gold near the cell surface and in solutions (Lengke et al.2006) in a temperature-dependent manner. This process takes up to one month at temperatures between 25°C and 100°C and is completed within a day at 200°C. Fusarium oxysporum and Rhodopseudomonas capsulata use species-specific NADH-dependent reductases for the bio-reduction of Au3+ ions to carry out the synthesis of gold nanoparticles (Shedbalkar et al. 2014). In Verticillum species, positively charged groups such as lysine residues in cell wall enzymes trap Au3+ ions on the surface of fungal cells via electrostatic interactions and subsequently cause their reduction and aggregation to form gold nanoparticles (Gericke and Pinches 2006a). Phanerochaete chrysosporium exploits both extracellular and intracellular modes and uses an enzyme-mediated route for gold nanoparticle production via lignolytic enzymes such as laccase and ligninase respectively. These enzymes use the charged amino acids to trap and reduce gold ions which form the seed nuclei, which subsequently aggregate to form into gold nanoparticles of sizes 128 ± 70 nm (Sanghi et al. 2011).
Anticancer potential
Microorganism-assisted synthesis of gold nanoparticles has been used for the detection and treatment of different types of cancers. Gold nanoparticles obtained from Candida albicans have shown promising results in the detection of liver cancer (Chauhan et al. 2011). The gold nanoparticles were synthesized by treating a cytosolic extract of Candida albicans with chloroaurate ions. Glutathione and dithiothreitol, were used as reducing agents leading to the formation of nanosized gold structures. Various analytical methods such as ultraviolet-visible spectroscopy, Fourier transform infrared spectroscopy, transmission electron microscopy, atomic force microscopy, dynamic light scattering and photon cross-correlation spectroscopy were used to characterize these nanoparticles. These gold colloids were then bioconjugated with liver cancer cell surface-specific antibodies and successfully used as a fluorescence-based biosensor to differentiate cancer cells specifically in a mixed cell population along with normal cells.
Mishra et al. synthesized gold nanoparticles by the extracellular route by utilizing Penicillium brevicompactum and studied its effect on mouse myoblast cancer C2C12 cell lines. Cytotoxicity occurred in a time-dependent manner. A direct correlation was found between the concentration of the gold salt and incubation time on the percentage of cell death (Mishra et al. 2011).
The cytotoxicity of gold nanoparticles synthesized from Enterococcus was evaluated in human hepatocellular cancer cell lines (HepG2). The biogenic gold nanoparticles inhibited the proliferation of HepG2 cells via intracellular reactive oxygen species mediated apoptosis and decreased proliferating cell nuclear antigen expressions, and demonstrated potential in treating hepatocellular carcinoma (Nandhini et al. 2020).
Silver nanoparticles
Microbial synthesized silver nanoparticles are an environment-friendly, economical, and biocompatible class of agents with numerous medical applications, including cancer therapeutics. They can cause oxidative stress and cytotoxicity to the cancer cells, alter their morphology, and reduce their viability (Aziz et al. 2019).
Green synthesis of silver nanoparticles using plants (Singh et al. 2020) (Paulkumar et al. 2014), bacteria (Nair and Pradeep 2002), fungi (Fayaz et al. 2009) (Makarov et al. 2014), seaweed (Shiny et al. 2013), algae (Mie et al.2013), and lichen (Mie et al. 2013) have been reported as eco-friendly alternatives to chemical and physical syntheses (Sakhare et al. 2021, Mustapha et al. 2022).
Mechanisms of NP production
Biosynthesis of nanoparticles may occur extracellularly or intracellularly. Silver nanoparticles have been synthesized via the extracellular route using several bacterial species such as Streptomyces sp. LK3 (Karthik et al. 2014) and Bacillus licheniformis (Vaidyanathan et al. 2010). Enzyme-mediated reduction of silver ions (Ag+) using NADH-dependent nitrate reductase has been noted as the mechanism, where the nitrate ions (NO3−) in silver nitrate accept two protons and are reduced to nitrite (NO2–) accompanied by the release of two electrons and water. The released electrons are responsible for the reduction of Ag+ ions to elemental silver (Ag0) (Kalimuthu et al. 2008).
Intracellular synthesis via non-enzymatic routes has also been reported in Lactobacillus A09. The anionic surface groups of the gram-positive bacterial cell walls function as silver ion biosorption sites (Zinov'ev and Sole 2004). At higher pH values, the bacterial cell wall monosaccharide rings get oxidized to open chain aldehydes, yielding electrons that can reduce the adsorbed silver ions to elemental silver Ag0 (Sintubin et al. 2009).
Fungi are another source of silver nanoparticle production (Mohanpuria et al.2008). Using a Liu Verticillium model system for the synthesis of silver nanoparticles, it has been postulated that the Ag+ ions are first trapped within the mycelial cell wall via electrostatic interactions with the negatively charged carboxylate groups and subsequently reduced by specific fungal reductase enzymes (Dhillon et al. 2012).
Anticancer potential
Silver nanoparticles have shown promising activity against a range of cancer cell lines. Khalifaet al. synthesized silver nanoparticles from the marine strain Bacillus sp. KFU36 and evaluated their effects in the MTT, wound healing, and flow cytometry assays. The silver nanoparticles showed excellent cytotoxic effects as well as induced apoptosis (61% at 50 μg/ml) in MCF-7 cell lines which manifested as a significant decrease in the cell viability (15% at 50 μg/ml), cell density, and adhesion capacity and loss of normal shape and size (Almalki and Khalifa 2020).
Yanget al. used cell-free culture filtrate of Pseudomonas aeruginosa for reducing silver nitrate to silver nanoparticles. Concentration-dependent cytotoxicity of the silver nanoparticles was observed on thyroid cancer cells (TPC1) cells along with a concomitant increase in intracellular reactive oxygen species generation and apoptotic morphological changes (Yang et al. 2020). Silver nanoparticles have shown size-dependent cytotoxic effects on human lung epithelial A549 cells with less than 10 nm being the optimal size for efficient cellular uptake and intracellular localization. The smaller-sized particles present a larger total surface area and consequently higher release rates of silver from the nanoparticles (Gliga et al. 2014).
Biogenic silver nanoparticles have been found to have anti-angiogenic properties and show efficacy in the treatment of retinal neovascularization (RNV)-like diseases. They function as inhibitors of angiogenic vascular endothelial growth factor (VEGFR) and block the downstream kinase activation by modulating VEGFR2 phosphorylation. Angiogenesis inhibition of silver nanoparticles has been successfully applied in onco-therapies (Shen et al. 2015). Another useful mechanism of the silver nanoparticles is their effect on the cell cycle. Different studies have suggested that green-synthesized silver nanoparticles induce apoptosis by causing cell cycle arrest at the sub-G1 phase (Singh et al. 2020, Noorbazargan et al. 2021).
In an elegant study, Baker et al. have shown that biogenic silver nanoparticles produced extracellularly from the noble fungi of the Alternaria species. are able to mimic the anticancer activity that was shown by the parent extract of fungal metabolites, proteins and enzymes. This proved that the silver nanoparticles were functionalized with these fungal components during synthesis. Moreover, the concentrated accumulation of the endogenous bioactive components in nanoscale levels resulted in higher synergistic anticancer activity (Baker et al. 2021)
Selenium nanoparticles (SeNPs)
Selenium is an essential trace element and part of many oxidoreductase enzymes. Glutathione peroxidases and many thioredoxin reductases contain selenium as a cofactor (Husen and Siddiqi 2014). Selenium nanoparticles are known to possess antimicrobial and anticancer properties (Forootanfar et al. 2014, Wadhwani et al. 2016). Unlike its inorganic and organic counterparts, the nanoparticulate form of selenium which has a narrow therapeutic window is seen to exhibit less toxicity (Shakibaie et al. 2010). Selenium nanoparticles have been produced by physical, chemical, and biological routes with the microbial methods bestowing many favourable attributes such as decreased toxicity, economical processing, eco-friendliness, and particulate stability. (Zhang et al. 2010, Iranifam et al. 2013).
Anticancer potential
Wadhwani et al. did a comparative study in breast cancer of selenium nanoparticles obtained from Acinetobacter sp. SW30 and that produced by chemical synthesis. Chemically synthesized selenium nanoparticles showed cytotoxicity against both cancer cells and normal cells, whereas the biogenic selenium nanoparticles were selectively toxic to the breast cancer cells. (Wadhwani et al. 2017)
Anticancer mechanisms
Xu et al. developed an efficient, economical, and environment-friendly biotechnological method for the biogenic synthesis of selenium nanoparticles using the probiotic Lactobacillus casei ATCC 393. Anaerobic conditions favoured the intracellular synthesis of nanoparticulate selenium which was found to be capped with polysaccharides as well as proteins. These nanoparticles induced apoptosis in HepG2 cells via signalling through the caspase cascade. In addition to their anticancer potential, these biomolecule-capped selenium nanoparticles demonstrated antioxidant properties as evidenced by reduction of diquat/hydrogen peroxide-induced oxidative damage in intestinal epithelial cells and favourable regulation of malondialdehyde and glutathione peroxidase activities (Xu et al. 2018).
Selenium nanoparticles obtained by synthesis from Bacillus strain ZYKT have been found to exhibit a strong, dose-dependent inhibitory effect on the H157 non-small cell lung cancer cell line (Bao et al. 2016). The authors have surmised that the cytotoxic effects of selenium nanoparticles are because of mitochondrial modulation of membrane potential and superoxide anion generation. In addition, selenite-exposed cancer cells have been found to be highly susceptible to cytotoxicity effects where the nanoparticulate selenium sequesters crucial functional proteins inside the cells and disrupts key metabolic and cellular processes including glycolytic pathways and microtubular events (Bao et al.2015).
Ahmad et al. have attributed the ability of selenium to bind divalent copper ions and its subsequent reduction to monovalent copper ions as a possible mechanism of the anticancer activity of selenium nanoparticles. The reversible process where the divalent copper ions are regenerated leads to the production of reactive oxygen species. High levels of copper in cancer cells induce the production of large amounts of reactive oxygen which selectively kills the cancer cells (Ahmad et al. 2015).
Iron oxide nanoparticles
Iron oxide nanoparticles are inorganic nanoparticles that are composed of ferromagnetic materials and show an imitable form of magnetism. This property is responsible for many of its applications such as magnetic separation, magnetic resonance imaging (MRI), magnetic fluid hyperthermia, and thermoablation as well as biosensing and targeted drug delivery. Iron oxide nanoparticles offer several advantages such as low cost of production, high stability, compatibility, and environmental safety. They have gained special attention as therapeutic nanomedicines in cancer treatment. In combination with chemotherapeutic agents, iron oxide nanoparticles have been used for targeted drug delivery or used as hypothermia agents (Hernández-Hernándezet al. 2020). Intracellular synthesis of iron oxide nanoparticles has been demonstrated in magnetotactic bacteria (MTB) (Bazylinski and Frankel 2004). Crystals synthesized by MTB such as Magnetospirillum gryphiswaldense MSR-1 are called magnetosomes (Berny et al. 2020). Iron oxide nanoparticles have also been synthesized extracellularly as seen in Geobacter sulfurreducens and actinomycetes MS2 (Byrne et al. 2015) or at cell surfaces of certain bacteria such as Klebsiella oxytoca or Staphylococcus warneri. The latter reduces ferric citrate under anaerobic conditions to produce biogenic Fe (III)—exopolysaccharides which are polysaccharide-iron hydrogel nanoparticles (Berny et al. 2020).
Alphandry et al. extracted chains of magnetosomes from AMB-1 magnetotactic bacteria and subjected them to suspensions containing MDA-MB-231 breast cancer cells. Exposure of this system to alternate magnetic fields of appropriate frequencies and field strengths resulted in the destruction of up to 100% of the cells. In vivo proof of antitumour activity was established by complete eradication of tumour xenografts under the skin in mouse models (Alphandéry et al. 2011). Figure 3 shows a schematic representation of the process. The authors attributed the antitumour activity of the magnetosome chains to hyperthermia effect triggered under an alternating magnetic field, which resulted in complete irradication of tumour xenografts induced under mouse skins. This effect was more efficient than that induced by superparamagnetic iron oxide nanoparticles.
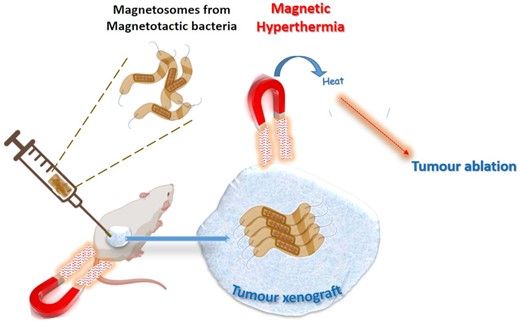
Schematic representation of tumour ablation by hyperthermia effect generated by magnetotactic bacteria under the influence of an applied magnetic field.
Magnesium oxide nanoparticles (MgONPs)
Pugazhendhi et al. documented the application of brown algae, Sargassum wightii in the biogenic synthesis of magnesium oxide nanoparticles, which acted as reducing and capping agents. Cytotoxicity of biogenic magnesium oxide nanoparticles was evaluated on lung cancer cell line A549 and was found to have a dose-dependent cytotoxic effect with an IC50 value of 77.5 ± 0.34 μg/ml. The magnesium oxide nanoparticles were found to be non-toxic during safety evaluation using peripheral blood mononuclear cells (Pugazhendhi et al. 2019).
Zinc oxide nanoparticles (ZnONPs)
ZnO nanoparticles have been synthesized using numerous microorganisms. Taran et al. reported the use of the Taguchi method for the optimization of zinc oxide nanoparticles using Halomonas elongate (Taran et al. 2018), while Rajabairavi et al. used the culture supernatant of endophytic bacterial isolate Sphingobacterium thalpophilum for the synthesis of ZnONP (Rajabairavi et al. 2017).
Saravanan et al. reported the synthesis of ZnO nanoparticles from Bacillus megaterium by the extracellular route which involved incubation of the cell-free culture supernatant to Zinc nitrate (Saravanan et al. 2018). The ZnONP exhibited concentration-dependent ROS induction and apoptotic effect in hMSc cells. While the biological effect on Helicobacter pylori was assessed in this study, the authors proposed further exploration of its anticancer potential.
Zinc oxide nanoparticles have demonstrated enhanced permeability and retention effects as well as cytotoxic potential via ROS generation against cancer cells (Bisht and Rayamajhi 2016). Either in pure metal oxide nanoparticulate form or co-doped preparations with chemotherapeutic agents such as paclitaxel, cisplatin, doxorubicin, and daunorubicin, they have been investigated against different kinds of cancer such as hepatocellular carcinoma, prostate cancer, non-small cell lung carcinoma, melanoma, cervix adenocarcinoma, head and neck squamous cell carcinoma, colon carcinoma and breast adenocarcinoma (Peng et al. 2015, Tripathy et al. 2015, Jiang et al. 2018). ZnONP have been found to be selectively cytotoxic to tumour cells, while preserving the sanctity of normal healthy cells by upregulating the mRNA and protein expression of tumour suppressor gene p53 and proapoptotic BAX and caspase-3, while simultaneously downregulating antiapoptotic Bcl-2. Additionally, ZnONPs also increase ROS generation in cancer cells and lower the levels of antioxidant GSH and ROS scavenging enzymes, superoxide dismutase, glutathione peroxidase, glutathione reductase, and catalase (Alrokayan 2012).
Green polymeric nanoplatforms for cancer therapeutics
Glucan-based nanoparticulate systems
Therapeutic nanoplatforms with microbe-specific activation have evolved considerably for the targeted treatment of cancer. Glucans are polysaccharides extracted from natural resources such as plants, bacteria, and fungi (Muta 2006).
Anticancer mechanisms
Many glucans have been reported to exert a stimulatory effect on the immune system by interacting with many critical receptors such as Dectin-1, complement receptor 3 (CR3), and TLR-2/6 present on innate immunity cells such as macrophages, neutrophils, and granulocytes (Gantner et al. 2003). β-glucan molecules have been explored for their potential as anticancer immune modulators that can exploit innate and adaptive immune responses within the tumour microenvironment (TME).
Systemic administration of certain β-glucans in preclinical mouse models has been demonstrated to effectively manipulate the TME with concomitant reduction of primary tumour growth and metastasis and improvement of clinical responses in cancer immunotherapy (Chan et al. 2009). β-glucans are susceptible to macrophage attack and undergo internalization and fragmentation after first forming association with its Dectin-1 and TLR-2/6 receptors. The β-glucan fragments are delivered to the reticuloendothelial system and released to be taken up by plasma granulocytes or macrophages via the CR3 receptor. This triggers an immune response, against tumours, leading to their opsonization and tumour cell death. Figure 4 is a depiction of the macrophage uptake of β-glucans and subsequent fragmentation, release and anti-tumour immune response.
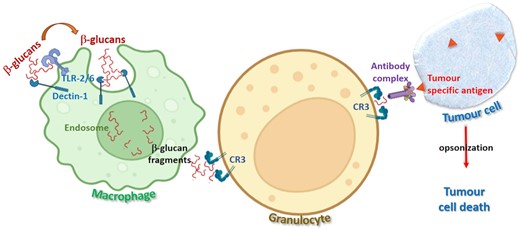
Schematic representation of immunomodulatory anticancer effect of β-glucans.
A BG36 glucan named lentinan derived from mushrooms has been reported to mount an immune response against tumourous cells by triggering the activity of lymphokine-activated killer cells and natural killer cells. Particulate β-glucans derived from yeast have been shown to mediate cytokine triggered antitumour immune responses while activating the innate immune effector cells (Lu et al. 2018).
Sizofiran, a β-glucan which is obtained from the mushroom, Schizophyllun commune exhibited significant anti-cancer effect on mice and rats. A mechanistic study indicated increased infiltration of T cells and Langerhans cells as the basis for the anticancer activity. Sizofiran has been explored for improving patient survival chances in advanced cervical cancer (Dhapte and Pokharkar 2019).
Jintao Huang et al. extracted β-glucan molecules with different chain lengths from yeast Saccharomyces cerevisiae. These β-glucan molecules, when loaded with the anticancer drug doxorubicin self-assembled via chiral interactions to form nanoparticles. These nanoparticles demonstrated enhanced drug loading and immune-potentiation and significantly improved cellular uptake and cytotoxicity against human breast cancer MCF-7 cell lines (Huang et al. 2020).
Bacterial-polymeric nanoplatforms
Sulfated dextran, sulfated curdlan, xanthan, and fucopol have been employed as immune-modulatory, anti-inflammatory, and antitumour agents (Freitas et al. 2011). The anticancer potential of fucoidan-based nanoparticles has been attributed to their targeting of p-selectin, which is expressed on metastatic cancer cells (Lu et al. 2017).
Gellan gum is an exopolysaccharide obtained from Sphingomonas elodea and Sphingomonas paucimobilis bacteria by fermentation technology (Wang et al. 2006). A nanohydrogel formulation using gellan gum for concomitant dual drug delivery of hydrophobic anticancer and anti-inflammatory drugs has been developed with Prednisolone and Paclitaxel as shown in Fig. 5. The nanohydrogel was able to enhance the solubility of the lipophilic drugs as well as showed excellent activity in the treatment of prostate cancer and inflammatory carcinomas. Additionally, there were other favourable effects such as reduction of oedema and elevated calcium levels and suppression of tumour-associated fever (D'Arrigoet al. 2014). This combination formulation was selectively more cytotoxic towards human prostate PC-3 cancer and human ovarian Skov-3 cancer cell lines as compared to murine fibroblast NIH/3T3, murine myocardiocyte H9C2, human ovarian A2780 cancer, and human breast MDAMB-231 cancer cell lines (Dhapte and Pokharkar 2019).
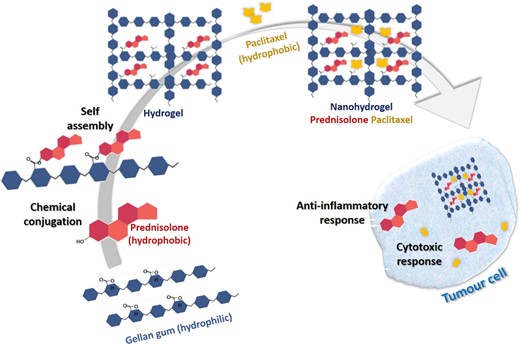
Schematic representation of formulation of a nanohydrogel from Gellan gum for concomitant dual drug delivery of prednisolone and paclitaxel for inflammatory carcinomas.
Fungal-polymeric nanoplatforms
Polysaccharides can also be obtained from fungi and have been used in the fabrication of novel drug delivery systems. Three types of polysaccharides have been obtained viz., extracellular or capsular polysaccharides which exist in the capsulated envelope, structural polymeric materials, and intracellular polysaccharides (Posocco et al. 2015). However, the latter two are obtained in lower yields, which limits their commercial use. Numerous examples of fungal polymeric nanoparticles have been explored in cancer therapeutics.
Chitin and chitosan obtained from fungal and yeast cell walls have been used in the formation of amorphous nanoparticles. Sustained release formulations of paclitaxel have been developed for the treatment of colon cancer cells using this approach (Smitha et al. 2013, Azuma et al. 2015). Arthrobotrys oligospora, a nematophagous fungus was used to fabricate glycosaminoglycan-containing nanoparticles (Wang et al.2013). Various glycosaminoglycans, such as heparin, and chondroitin have been explored as potential anticancer agents owing to their inhibitory effects on tumour cell adhesion, migration, growth, and invasion (Yip et al. 2006). Proteoglycans formed by covalent conjugation of core proteins like decorin to glycosaminoglycans have been found to significantly reduce primary tumour growth and eliminate metastasis in an orthotopic mammary carcinoma model (Reed et al. 2005). Mingjun Zhang explored the in vitro cytotoxic effects of fungus-based nanoparticles from Arthrobotrys oligospora and found them to be cytotoxic to mouse melanoma B16BL6 and human lung cancer A549 cells, thereby demonstrating their potential for tumour therapy (Zhang 2013).
Seaweed-polymeric nanoplatforms
Seaweeds are rich in multifunctional bioactive components such as phenolic and sulphated polysaccharides and have been the focus of research exploring new compounds and therapies for the prevention, control, and reduction of cancer development (Cotas et al. 2021). They also contain poly- and highly unsaturated fatty acids (PUFAs and HUFAs), essential amino acids, vitamins, and minerals (calcium, iron, iodine, magnesium, phosphorus, potassium, zinc, copper, manganese, selenium, and fluoride). Marine algae are a rich source of polysaccharides, mycopolysaccharides, and storage polysaccharides and have found commercial applications as stabilizers, emulsifiers, and thickening agents. Seaweed polysaccharides have many attractive attributes and potential for several therapeutic uses such as anticancer, antiviral, antioxidant, and anticoagulant activities (Noreen et al. 2016).
Hydrophilic seaweed polysaccharides such as alginates, laminarins, and ulvans carry several polar reactive functionalities in their structure and have been explored as drug delivery systems. Alginate nanoparticles are biocompatible, hydrophilic, and bio-adhesive in nature and are capable of excellent drug conjugation. They show selective targeting of tumour cells and significant anticancer efficacy.
Paclitaxel-loaded alginate nanoparticles have been shown to induce apoptosis in human primary patient breast cancer cells and cause targeted cell cycle arrest along with a significant decrease in cell viability in comparison to Taxol (Dhapte and Pokharkar 2019). An elegant study on hyaluronic acid (HA)—agar-based hydrogels showed their potential in intravitreal drug delivery systems via effective drug migration through biological vitreous humor (Thakur et al. 2020). Injectable agar-based hydrogels with MoS2/Bi2S3-PEG (MBP) and doxorubicin (DOX) have been developed for concurrent onco-photothermal and chemotherapy. Direct injection of the formulated solution into the tumour area and subsequent, automatic formation of the static, macro-vessel-like gel at the site of delivery after cooling of the solution helps in protecting the MBP nanosheet and DOX from the body fluid circulation (Wu et al. 2018). Curcumin when loaded on carrier systems made of kappa-carrageenan, a seaweed-derived polymer showed improved delivery and better efficacy against lung cancer cells (Sathuvan et al. 2017). Zinc oxide nanoparticles developed from Sargassum muticum exhibited apoptosis and inhibited angiogenesis in HepG2 cells (Sanaeimehr et al. 2018a).
Green synthesis of nanoparticles has attracted enormous attention in recent times, with numerous research groups exploring this route for its anticancer potential. Table 4 compiles some recent promising preclinical explorations on microbial synthesised nanoparticles and magnetosomes and their evaluation against various cancer cell lines.
Microbial synthesized nanoparticles: Source microorganism, biosynthetic approach, functionalization details, and anticancer potential.
Microorganism . | Source . | Nano Particle . | Mode of synthesis . | Capping agent/s . | Cancer Cell line . | Anticancer potential . | Reference . |
---|---|---|---|---|---|---|---|
Padina tetrastromatica | Brown seaweed | Gold | Extra-cellular | Fucoidan | Hep G2 A549 | LiverLung | (Rajeshkumar et al. 2021) |
Enterococcus sp | Marine bacteria | Gold | Extra-cellular | Compounds with carboxylic and amine groups | Hep G2 A549 | LiverLung | (Rajeshkumar 2016) |
Micrococcus yunnanensis J2 | Bacteria | Gold | Extra-cellular | - | U87 HT1080 PC12Caco2, MCF7 A549 | Glioblastoma Fibrosarcoma Pheochromo-cytoma, Colorectal BreastLung | (Jafari et al. 2018) |
Mycobacterium sp. BRS2A-AR2 | Myco-bacteria | Gold | Extra-cellular | Compounds with carboxylic acid, hydroxy, and amine groups | HeLa | Cervical cancer | (Camas et al. 2018) |
Pleurotus ostreatus | Fungus | Gold | Extra-cellular | Compounds with amine groups | HepG2 HCT-116 | LiverColon | (El Domany et al. 2018) |
Cystoseira baccata | Brown algae | Gold | Extra-cellular | Polyphenols, polysaccharides, proteins | Caco-2 HT-29 | ColorectalColon | (González-Ballesteros et al. 2017) |
Vibrio alginolyticus | Marine bacteria | Gold | Extra-cellular | Compounds having alkenes, carboxylic acid, halogen, and hydroxy groups | HCA-7 | Colon | (Shunmugam et al. 2021) |
Leptolyngbya | Cyano-bacteria | Silver | Extra-cellular | Polyphenols, polysaccharides, proteins | HeLa | Cervical cancer | (Zada et al. 2018) |
Enteromorpha compressa | Green seaweed | Silver | Extra-cellular | Compounds with carboxylic acid and hydroxy groups | EAC | Ehlrich Ascites Carcinoma | (Ramkumar et al. 2017) |
Ganoderma sessiliforme | Wild mushroom | Silver | Extra-cellular | Polyphenols, anthocyanins, flavonoids, and nitrogen compounds (proteins) | MCF-7 MDA-MB231 | Breast | (Mohanta et al. 2018) |
Metschnikowia sp. | Yeast | Silver | Extra-cellular | - | H1975 A579 | Lung | (Liu et al. 2021) |
D. radiodurans | Bacteria | Silver | Extra-cellular | Compounds with carboxylic acid, hydroxyl, disulphide, amides and thioesters groups | MCF-7 | Breast | (Kulkarni et al. 2015) |
Cryptococcus laurentii | Yeast | Silver | Extra-cellular | yeast proteins, polymeric carbohydrates—arabinogalactan | MCF7 T47D | Breast | (Ortega et al. 2015) |
Magnetospirillum gryphiswaldense MSR1 | Bacteria | Magne-tosomes | Extra-cellular | Anthracyclines | HepG2 | Liver | (Geng et al. 2019) |
Magnetospirillum magneticum AMB1 | Bacteria | Magne-tosomes | Extra-cellular | Arabinoside, Anthracyclines | HL-60 | Leukaemia | (Long et al. 2016) |
Aspergillus terreus | Fungus | Zinc oxide | Extra-cellular | Compounds with carboxyl, alkene, amino, imino, and ether groups | MCF-7 | Breast | (Baskar et al. 2015) |
Sargassum muticum | Algae | Zinc oxide | Extra-cellular | - | HepG2 | Liver | (Sanaeimehr et al. 2018b) |
Acinetobacter sp. SW30 | Bacteria | Selenium | Extra-cellular | Proteins (amino acids, amides) | 4T1 MCF-7 | Breast | (Wadhwani et al. 2017) |
Lactobacillus casei | Bacteria | Copper oxide | Extra-cellular | HT-29 AGS | ColonGastric | (Kouhkan et al. 2019) |
Microorganism . | Source . | Nano Particle . | Mode of synthesis . | Capping agent/s . | Cancer Cell line . | Anticancer potential . | Reference . |
---|---|---|---|---|---|---|---|
Padina tetrastromatica | Brown seaweed | Gold | Extra-cellular | Fucoidan | Hep G2 A549 | LiverLung | (Rajeshkumar et al. 2021) |
Enterococcus sp | Marine bacteria | Gold | Extra-cellular | Compounds with carboxylic and amine groups | Hep G2 A549 | LiverLung | (Rajeshkumar 2016) |
Micrococcus yunnanensis J2 | Bacteria | Gold | Extra-cellular | - | U87 HT1080 PC12Caco2, MCF7 A549 | Glioblastoma Fibrosarcoma Pheochromo-cytoma, Colorectal BreastLung | (Jafari et al. 2018) |
Mycobacterium sp. BRS2A-AR2 | Myco-bacteria | Gold | Extra-cellular | Compounds with carboxylic acid, hydroxy, and amine groups | HeLa | Cervical cancer | (Camas et al. 2018) |
Pleurotus ostreatus | Fungus | Gold | Extra-cellular | Compounds with amine groups | HepG2 HCT-116 | LiverColon | (El Domany et al. 2018) |
Cystoseira baccata | Brown algae | Gold | Extra-cellular | Polyphenols, polysaccharides, proteins | Caco-2 HT-29 | ColorectalColon | (González-Ballesteros et al. 2017) |
Vibrio alginolyticus | Marine bacteria | Gold | Extra-cellular | Compounds having alkenes, carboxylic acid, halogen, and hydroxy groups | HCA-7 | Colon | (Shunmugam et al. 2021) |
Leptolyngbya | Cyano-bacteria | Silver | Extra-cellular | Polyphenols, polysaccharides, proteins | HeLa | Cervical cancer | (Zada et al. 2018) |
Enteromorpha compressa | Green seaweed | Silver | Extra-cellular | Compounds with carboxylic acid and hydroxy groups | EAC | Ehlrich Ascites Carcinoma | (Ramkumar et al. 2017) |
Ganoderma sessiliforme | Wild mushroom | Silver | Extra-cellular | Polyphenols, anthocyanins, flavonoids, and nitrogen compounds (proteins) | MCF-7 MDA-MB231 | Breast | (Mohanta et al. 2018) |
Metschnikowia sp. | Yeast | Silver | Extra-cellular | - | H1975 A579 | Lung | (Liu et al. 2021) |
D. radiodurans | Bacteria | Silver | Extra-cellular | Compounds with carboxylic acid, hydroxyl, disulphide, amides and thioesters groups | MCF-7 | Breast | (Kulkarni et al. 2015) |
Cryptococcus laurentii | Yeast | Silver | Extra-cellular | yeast proteins, polymeric carbohydrates—arabinogalactan | MCF7 T47D | Breast | (Ortega et al. 2015) |
Magnetospirillum gryphiswaldense MSR1 | Bacteria | Magne-tosomes | Extra-cellular | Anthracyclines | HepG2 | Liver | (Geng et al. 2019) |
Magnetospirillum magneticum AMB1 | Bacteria | Magne-tosomes | Extra-cellular | Arabinoside, Anthracyclines | HL-60 | Leukaemia | (Long et al. 2016) |
Aspergillus terreus | Fungus | Zinc oxide | Extra-cellular | Compounds with carboxyl, alkene, amino, imino, and ether groups | MCF-7 | Breast | (Baskar et al. 2015) |
Sargassum muticum | Algae | Zinc oxide | Extra-cellular | - | HepG2 | Liver | (Sanaeimehr et al. 2018b) |
Acinetobacter sp. SW30 | Bacteria | Selenium | Extra-cellular | Proteins (amino acids, amides) | 4T1 MCF-7 | Breast | (Wadhwani et al. 2017) |
Lactobacillus casei | Bacteria | Copper oxide | Extra-cellular | HT-29 AGS | ColonGastric | (Kouhkan et al. 2019) |
Microbial synthesized nanoparticles: Source microorganism, biosynthetic approach, functionalization details, and anticancer potential.
Microorganism . | Source . | Nano Particle . | Mode of synthesis . | Capping agent/s . | Cancer Cell line . | Anticancer potential . | Reference . |
---|---|---|---|---|---|---|---|
Padina tetrastromatica | Brown seaweed | Gold | Extra-cellular | Fucoidan | Hep G2 A549 | LiverLung | (Rajeshkumar et al. 2021) |
Enterococcus sp | Marine bacteria | Gold | Extra-cellular | Compounds with carboxylic and amine groups | Hep G2 A549 | LiverLung | (Rajeshkumar 2016) |
Micrococcus yunnanensis J2 | Bacteria | Gold | Extra-cellular | - | U87 HT1080 PC12Caco2, MCF7 A549 | Glioblastoma Fibrosarcoma Pheochromo-cytoma, Colorectal BreastLung | (Jafari et al. 2018) |
Mycobacterium sp. BRS2A-AR2 | Myco-bacteria | Gold | Extra-cellular | Compounds with carboxylic acid, hydroxy, and amine groups | HeLa | Cervical cancer | (Camas et al. 2018) |
Pleurotus ostreatus | Fungus | Gold | Extra-cellular | Compounds with amine groups | HepG2 HCT-116 | LiverColon | (El Domany et al. 2018) |
Cystoseira baccata | Brown algae | Gold | Extra-cellular | Polyphenols, polysaccharides, proteins | Caco-2 HT-29 | ColorectalColon | (González-Ballesteros et al. 2017) |
Vibrio alginolyticus | Marine bacteria | Gold | Extra-cellular | Compounds having alkenes, carboxylic acid, halogen, and hydroxy groups | HCA-7 | Colon | (Shunmugam et al. 2021) |
Leptolyngbya | Cyano-bacteria | Silver | Extra-cellular | Polyphenols, polysaccharides, proteins | HeLa | Cervical cancer | (Zada et al. 2018) |
Enteromorpha compressa | Green seaweed | Silver | Extra-cellular | Compounds with carboxylic acid and hydroxy groups | EAC | Ehlrich Ascites Carcinoma | (Ramkumar et al. 2017) |
Ganoderma sessiliforme | Wild mushroom | Silver | Extra-cellular | Polyphenols, anthocyanins, flavonoids, and nitrogen compounds (proteins) | MCF-7 MDA-MB231 | Breast | (Mohanta et al. 2018) |
Metschnikowia sp. | Yeast | Silver | Extra-cellular | - | H1975 A579 | Lung | (Liu et al. 2021) |
D. radiodurans | Bacteria | Silver | Extra-cellular | Compounds with carboxylic acid, hydroxyl, disulphide, amides and thioesters groups | MCF-7 | Breast | (Kulkarni et al. 2015) |
Cryptococcus laurentii | Yeast | Silver | Extra-cellular | yeast proteins, polymeric carbohydrates—arabinogalactan | MCF7 T47D | Breast | (Ortega et al. 2015) |
Magnetospirillum gryphiswaldense MSR1 | Bacteria | Magne-tosomes | Extra-cellular | Anthracyclines | HepG2 | Liver | (Geng et al. 2019) |
Magnetospirillum magneticum AMB1 | Bacteria | Magne-tosomes | Extra-cellular | Arabinoside, Anthracyclines | HL-60 | Leukaemia | (Long et al. 2016) |
Aspergillus terreus | Fungus | Zinc oxide | Extra-cellular | Compounds with carboxyl, alkene, amino, imino, and ether groups | MCF-7 | Breast | (Baskar et al. 2015) |
Sargassum muticum | Algae | Zinc oxide | Extra-cellular | - | HepG2 | Liver | (Sanaeimehr et al. 2018b) |
Acinetobacter sp. SW30 | Bacteria | Selenium | Extra-cellular | Proteins (amino acids, amides) | 4T1 MCF-7 | Breast | (Wadhwani et al. 2017) |
Lactobacillus casei | Bacteria | Copper oxide | Extra-cellular | HT-29 AGS | ColonGastric | (Kouhkan et al. 2019) |
Microorganism . | Source . | Nano Particle . | Mode of synthesis . | Capping agent/s . | Cancer Cell line . | Anticancer potential . | Reference . |
---|---|---|---|---|---|---|---|
Padina tetrastromatica | Brown seaweed | Gold | Extra-cellular | Fucoidan | Hep G2 A549 | LiverLung | (Rajeshkumar et al. 2021) |
Enterococcus sp | Marine bacteria | Gold | Extra-cellular | Compounds with carboxylic and amine groups | Hep G2 A549 | LiverLung | (Rajeshkumar 2016) |
Micrococcus yunnanensis J2 | Bacteria | Gold | Extra-cellular | - | U87 HT1080 PC12Caco2, MCF7 A549 | Glioblastoma Fibrosarcoma Pheochromo-cytoma, Colorectal BreastLung | (Jafari et al. 2018) |
Mycobacterium sp. BRS2A-AR2 | Myco-bacteria | Gold | Extra-cellular | Compounds with carboxylic acid, hydroxy, and amine groups | HeLa | Cervical cancer | (Camas et al. 2018) |
Pleurotus ostreatus | Fungus | Gold | Extra-cellular | Compounds with amine groups | HepG2 HCT-116 | LiverColon | (El Domany et al. 2018) |
Cystoseira baccata | Brown algae | Gold | Extra-cellular | Polyphenols, polysaccharides, proteins | Caco-2 HT-29 | ColorectalColon | (González-Ballesteros et al. 2017) |
Vibrio alginolyticus | Marine bacteria | Gold | Extra-cellular | Compounds having alkenes, carboxylic acid, halogen, and hydroxy groups | HCA-7 | Colon | (Shunmugam et al. 2021) |
Leptolyngbya | Cyano-bacteria | Silver | Extra-cellular | Polyphenols, polysaccharides, proteins | HeLa | Cervical cancer | (Zada et al. 2018) |
Enteromorpha compressa | Green seaweed | Silver | Extra-cellular | Compounds with carboxylic acid and hydroxy groups | EAC | Ehlrich Ascites Carcinoma | (Ramkumar et al. 2017) |
Ganoderma sessiliforme | Wild mushroom | Silver | Extra-cellular | Polyphenols, anthocyanins, flavonoids, and nitrogen compounds (proteins) | MCF-7 MDA-MB231 | Breast | (Mohanta et al. 2018) |
Metschnikowia sp. | Yeast | Silver | Extra-cellular | - | H1975 A579 | Lung | (Liu et al. 2021) |
D. radiodurans | Bacteria | Silver | Extra-cellular | Compounds with carboxylic acid, hydroxyl, disulphide, amides and thioesters groups | MCF-7 | Breast | (Kulkarni et al. 2015) |
Cryptococcus laurentii | Yeast | Silver | Extra-cellular | yeast proteins, polymeric carbohydrates—arabinogalactan | MCF7 T47D | Breast | (Ortega et al. 2015) |
Magnetospirillum gryphiswaldense MSR1 | Bacteria | Magne-tosomes | Extra-cellular | Anthracyclines | HepG2 | Liver | (Geng et al. 2019) |
Magnetospirillum magneticum AMB1 | Bacteria | Magne-tosomes | Extra-cellular | Arabinoside, Anthracyclines | HL-60 | Leukaemia | (Long et al. 2016) |
Aspergillus terreus | Fungus | Zinc oxide | Extra-cellular | Compounds with carboxyl, alkene, amino, imino, and ether groups | MCF-7 | Breast | (Baskar et al. 2015) |
Sargassum muticum | Algae | Zinc oxide | Extra-cellular | - | HepG2 | Liver | (Sanaeimehr et al. 2018b) |
Acinetobacter sp. SW30 | Bacteria | Selenium | Extra-cellular | Proteins (amino acids, amides) | 4T1 MCF-7 | Breast | (Wadhwani et al. 2017) |
Lactobacillus casei | Bacteria | Copper oxide | Extra-cellular | HT-29 AGS | ColonGastric | (Kouhkan et al. 2019) |
The extracellular route appears to be the preferred approach for the microbe-mediated biosynthesis of nanoparticles. Endogenous biomolecules originating from the microorganisms themselves adopt multifunctional roles, contributing towards metal ion reduction as well as nanoparticle capping and stabilization. Hydroxyl, carboxy, amino, sulphate, amine and amide functional groups are provided by biomolecules such as polyphenols, anthocyanins, flavonoids, and proteins, which play a crucial role in the capping and stabilization of nanoparticles. Unlike chemical synthesis, microbial methods of production with inherent biomolecule-based surface capping leads to polydisperse nanoparticles that do not agglomerate. A number of metallic nanoparticles and magnetosomes derived from microorganisms have shown promising anticancer activity against a variety of tumours such as liver, lung, colorectal, colon, breast, glioblastoma, gastric, and cervical cancers. Green synthesis of nanoparticles holds a lot of potential in minimizing the non-specific toxicity of metallic nanoparticles, while inducing apoptosis of tumorous tissues.
Conclusion
Synthesis of nanoparticles by microbes has emerged as an efficient, eco-friendly, rapid, and scalable approach for the targeted delivery of therapeutic moieties. Microbes can function as potential nano-factories for the biosynthesis of metal nanoparticles or metal-based composite materials and have been exploited for various therapeutic applications. This review is a compilation of several metallic and polymeric microbe-derived nano delivery systems and their applications in cancer therapeutics. Microorganism-derived metal nanoparticles and polymeric nanoplatforms such as exopolysaccharides and glucans have shown significant efficacy in the targeted delivery of anticancer drugs with enhanced therapeutic load, bioavailability, safety, and sustained release characteristics, thereby amplifying the therapeutic efficiency of cancer treatment. Research on seaweed polysaccharides-based formulations has expanded owing to their easy availability, cost-effectiveness, and non-toxic, biodegradable, and biocompatible nature. Additionally, encapsulated forms of hydrophobic anti-cancer agents in natural hydrophilic seaweed polysaccharides help in minimizing side effects and enhancing their dispersion in the human body, thereby magnifying their therapeutic efficacy. Further translation research on microbe-based nanoplatforms from in vitro preclinical to clinical setting and optimization of their scale-up potential will greatly enhance the utility of these promising green drug delivery platforms in cancer therapeutics.
Acknowledgements
This research did not receive any specific grant from funding agencies in the public, commercial, or not-for-profit sectors.
Conflict of interest
The authors have no conflict of interest to declare. In addition, the authors declare that the project was conducted in the absence of any commercial or financial relationships that could be construed as a potential conflict of interest.
Author contributions
MJ and AP conceptualized the idea of the manuscript. MJ wrote the initial draft of the manuscript, which was read and edited by AP. Both authors listed have made a substantial, direct, and intellectual contribution to the work, and approved it for publication.
Data availability statement
Data sharing is not applicable to this article as no new data were created or analysed in this study.