-
PDF
- Split View
-
Views
-
Cite
Cite
Wuen Ee Foong, Heng-Keat Tam, Jan J Crames, Beate Averhoff, Klaas M Pos, The chloramphenicol/H+ antiporter CraA of Acinetobacter baumannii AYE reveals a broad substrate specificity, Journal of Antimicrobial Chemotherapy, Volume 74, Issue 5, May 2019, Pages 1192–1201, https://doi.org/10.1093/jac/dkz024
- Share Icon Share
Abstract
To identify major facilitator superfamily (MFS)-type chloramphenicol transporters of Acinetobacter baumannii AYE, to characterize its substrate specificity and identify CraA substrate and H+ binding sites.
Five ORFs predicted to encode chloramphenicol transporters were heterologously expressed in Escherichia coli and their substrate specificity was determined by drug susceptibility assays on solid agar medium. CraA transport properties were determined via whole cell fluorescence experiments using ethidium and dequalinium. ACMA quenching was used to characterize the H+/drug antiport process in everted membrane vesicles. The function of CraA in A. baumannii was determined by drug susceptibility assay using A. baumannii ATCC 19606 ΔcraA.
CraA, ABAYE0913 and CmlA5 are functionally active when overproduced in E. coli. ABAYE0913 conferred resistance to florfenicol and benzalkonium, CmlA5 conferred resistance to chloramphenicol and thiamphenicol, and craA expression resulted in resistance to chloramphenicol, thiamphenicol, florfenicol, ethidium, dequalinium, chlorhexidine, benzalkonium, mitomycin C and TPP+. Cell expressing craA_E38A showed no resistance to all tested drugs, implying that Glu-38 is involved in the binding of drugs and/or protons. Functional assays indicated that substitution of Asp-46 to Ala resulted in severe susceptibility to cationic drugs, chloramphenicol and thiamphenicol. In contrast, Glu-338 is important for the recognition of chloramphenicol, florfenicol, chlorhexidine and dequalinium.
This study suggests that CraA has a broad substrate specificity, similar to that of E. coli MdfA. However, due to the presence of three charged residues in the transmembrane region conferring different susceptibility profiles upon substitution to Ala, we postulate that CraA has a different substrate recognition mode compared with MdfA.
Introduction
The rapid emergence of MDR bacteria is threatening the effectiveness of currently available antibiotics. Recently, the WHO declared that there is an urgent need to develop new antibiotics to avert the threat of Acinetobacter baumannii. This Gram-negative opportunistic pathogen, which causes nosocomial infections in immunocompromised patients, is highly associated with morbidity and mortality.1,2 The emergence of multidrug or even pan-drug resistance in A. baumannii results from its high level of genomic plasticity, allowing it to acquire a repertoire of antimicrobial resistance genes.1,3–6 Indeed, genome sequencing of A. baumannii AYE (AbAYE) revealed a Tn7-like transposon, comprising an 86 kb AbaR1 resistance island harbouring 45 genes associated with resistance to antimicrobial drugs, heavy metals and antiseptics.7,8
One of the main factors contributing to MDR in A. baumannii is the up-regulation of various chromosomally encoded efflux pumps, both intrinsic and/or acquired, which expel toxic compounds from the cells with high efficiency.9,10 Among different classes of MDR transporters, AdeABC, AdeFGH and AdeIJK, which belong to the resistance nodulation and cell division (RND) superfamily as part of multicomponent tripartite system, have a significant role in extruding a broad range of substrates.11–13 Single-component transporters including CraA and AmvA from the major facilitator superfamily (MFS),14,15 AbeS from the small multidrug resistance family,16,17 AbeM from the multidrug and toxic compound extrusion family,18 and AceI from the proteobacterial antimicrobial compound efflux family19 extrude the drugs from the cytoplasmic side into the periplasmic space, from where RND-type transporters take up and expel drugs across the outer membranes. CraA of A. baumannii ATCC 19606 (Ab19606) as a homologue of MdfA and MdtM was reported to confer resistance specifically against chloramphenicol.14 In contrast, MdfA and MdtM from Escherichia coli confer resistance to a variety of neutral, monovalent cationic and long divalent cationic (LDC) drugs (Table 1; Figure S1, available as Supplementary data at JAC Online).20–25 In A. baumannii, it has been shown that AdeABC, AdeFGH and AdeIJK also contribute to resistance to chloramphenicol.10 It is likely that these tripartite systems act in synergy with CraA towards the overall chloramphenicol resistance phenotype,14,26 particularly if chloramphenicol acetyltransferase is absent.27
Proteins . | Substrate . | Substrate type . |
---|---|---|
MdfA | chloramphenicol20 | antibiotic |
norfloxacin20 | antibiotic | |
puromycin20 | antibiotic | |
tetracycline20 | antibiotic | |
thiamphenicol25 | antibiotic | |
pentamidine21 | antiprotozoal, antifungal agent | |
chlorhexidine21 | antiseptic | |
dequalinium21 | antiseptic | |
benzalkonium20 | biocide | |
ethidium20 | dye | |
TPP+20 | lipophilic cation | |
MdtM | chloramphenicol22 | antibiotic |
furaltadone23 | antibiotic | |
norfloxacin22 | antibiotic | |
puromycin23 | antibiotic | |
tinidazole23 | antiprotozoal agent | |
benzalkonium24 | biocide | |
acriflavine22 | dye | |
crystal violet23 | dye | |
ethidium22 | dye | |
TPP+22 | lipophilic cation | |
benzethonium24 | quaternary ammonium cation | |
cetrimonium24 | quaternary ammonium cation | |
cetylpyridinium24 | quaternary ammonium cation | |
dimethyldioctadecyl ammonium24 | quaternary ammonium cation | |
domiphen24 | quaternary ammonium cation | |
tetraethylammonium24 | quaternary ammonium cation |
Proteins . | Substrate . | Substrate type . |
---|---|---|
MdfA | chloramphenicol20 | antibiotic |
norfloxacin20 | antibiotic | |
puromycin20 | antibiotic | |
tetracycline20 | antibiotic | |
thiamphenicol25 | antibiotic | |
pentamidine21 | antiprotozoal, antifungal agent | |
chlorhexidine21 | antiseptic | |
dequalinium21 | antiseptic | |
benzalkonium20 | biocide | |
ethidium20 | dye | |
TPP+20 | lipophilic cation | |
MdtM | chloramphenicol22 | antibiotic |
furaltadone23 | antibiotic | |
norfloxacin22 | antibiotic | |
puromycin23 | antibiotic | |
tinidazole23 | antiprotozoal agent | |
benzalkonium24 | biocide | |
acriflavine22 | dye | |
crystal violet23 | dye | |
ethidium22 | dye | |
TPP+22 | lipophilic cation | |
benzethonium24 | quaternary ammonium cation | |
cetrimonium24 | quaternary ammonium cation | |
cetylpyridinium24 | quaternary ammonium cation | |
dimethyldioctadecyl ammonium24 | quaternary ammonium cation | |
domiphen24 | quaternary ammonium cation | |
tetraethylammonium24 | quaternary ammonium cation |
Proteins . | Substrate . | Substrate type . |
---|---|---|
MdfA | chloramphenicol20 | antibiotic |
norfloxacin20 | antibiotic | |
puromycin20 | antibiotic | |
tetracycline20 | antibiotic | |
thiamphenicol25 | antibiotic | |
pentamidine21 | antiprotozoal, antifungal agent | |
chlorhexidine21 | antiseptic | |
dequalinium21 | antiseptic | |
benzalkonium20 | biocide | |
ethidium20 | dye | |
TPP+20 | lipophilic cation | |
MdtM | chloramphenicol22 | antibiotic |
furaltadone23 | antibiotic | |
norfloxacin22 | antibiotic | |
puromycin23 | antibiotic | |
tinidazole23 | antiprotozoal agent | |
benzalkonium24 | biocide | |
acriflavine22 | dye | |
crystal violet23 | dye | |
ethidium22 | dye | |
TPP+22 | lipophilic cation | |
benzethonium24 | quaternary ammonium cation | |
cetrimonium24 | quaternary ammonium cation | |
cetylpyridinium24 | quaternary ammonium cation | |
dimethyldioctadecyl ammonium24 | quaternary ammonium cation | |
domiphen24 | quaternary ammonium cation | |
tetraethylammonium24 | quaternary ammonium cation |
Proteins . | Substrate . | Substrate type . |
---|---|---|
MdfA | chloramphenicol20 | antibiotic |
norfloxacin20 | antibiotic | |
puromycin20 | antibiotic | |
tetracycline20 | antibiotic | |
thiamphenicol25 | antibiotic | |
pentamidine21 | antiprotozoal, antifungal agent | |
chlorhexidine21 | antiseptic | |
dequalinium21 | antiseptic | |
benzalkonium20 | biocide | |
ethidium20 | dye | |
TPP+20 | lipophilic cation | |
MdtM | chloramphenicol22 | antibiotic |
furaltadone23 | antibiotic | |
norfloxacin22 | antibiotic | |
puromycin23 | antibiotic | |
tinidazole23 | antiprotozoal agent | |
benzalkonium24 | biocide | |
acriflavine22 | dye | |
crystal violet23 | dye | |
ethidium22 | dye | |
TPP+22 | lipophilic cation | |
benzethonium24 | quaternary ammonium cation | |
cetrimonium24 | quaternary ammonium cation | |
cetylpyridinium24 | quaternary ammonium cation | |
dimethyldioctadecyl ammonium24 | quaternary ammonium cation | |
domiphen24 | quaternary ammonium cation | |
tetraethylammonium24 | quaternary ammonium cation |
As MFS-type MDR transporters are important efflux contributors to the MDR in A. baumannii alongside RND-type transporters, we tested five putative MFS-type chloramphenicol transporters of AbAYE for their ability to confer drug resistance in E. coli. We found that CraA, when overexpressed in E. coli, is a broad-spectrum efflux pump, much like MdfA from E. coli, rather than a highly specific chloramphenicol transporter.14 From the other tested MFS-type transporters, we found that ABAYE0913 exhibits resistance to a broader spectrum of substrates as well, whereas CmlA5 appears to be a more specific chloramphenicol/thiamphenicol transporter.
Materials and methods
Cloning of multidrug efflux pumps genes and site-directed mutagenesis
Five putative MFS-type chloramphenicol transporter genes [12_508 (CraA) or ABAYE0338 (CraAshort), ABAYE0685, ABAYE0913, ABAYE3620 (CmlA5) and ABAYE3640 (CmlA)] were amplified from chromosomal DNA of AbAYE and inserted into pTTQ18 vector by the Gibson Assembly.28 By way of the latter method, a 6×-His-tag was attached to each of the C-termini of the proteins and could be used to detect the production of the proteins via Western blot analysis. Amino acid substitutions were introduced using the ExSite site-directed mutagenesis protocol (Stratagene). The inserted genes were verified by sequencing (Eurofins). All cloning primers are listed in Table S1.
Drug agar plate assay in E. coli
Drug susceptibility assays were performed as previously described29 with slight modifications. Briefly, overnight cell cultures of E. coli BW25113 ΔacrABΔemrEΔmdfA (BW25113 ΔacrABΔemrEΔmdfA) or E. coli BW25113 ΔemrEΔmdfA (BW25113 ΔemrEΔmdfA) harbouring empty vector (pTTQ18), pTTQ18_MFS-type_chloramphenicol_transporters, pTTQ18_CraA_WT and craA mutants were adjusted to OD600 100–10−5 and 4 μL of the diluted cultures was spotted on a LB agar plate supplemented with 100 mg/L ampicillin, 0.2 mM IPTG and tested drugs (Figure S1). Plates were incubated at 37°C overnight. The expression of craA and craA mutants was determined by detecting the proteins produced by Western blot analysis using anti‐His–alkaline-phosphatase antibody (Sigma–Aldrich).
Construction of craA gene knock-out and complementation
Construction of Ab19606 ΔcraA was performed as previously described.30 The detailed method is described in the Supplementary Methods. The phenotype of the Ab19606 WT, Ab19606 ΔcraA and Ab19606 ΔcraA pBAV1K_CraA was tested by drug susceptibility assay using LB agar plates supplemented with 50 mg/L kanamycin and the tested drugs.
Dequalinium transport assay
The experiment for detection of dequalinium accumulation in E. coli was performed as previously described21 with slight modifications. Briefly, 50 μL of the cell suspension was mixed with 950 μL of pre-warmed dequalinium-transport buffer supplemented with 2 μM dequalinium, in the absence or presence of 1 mM chloramphenicol. The dequalinium fluorescence was measured immediately at an excitation and emission wavelength of 330 and 370 nm, respectively. The detailed method is described in the Supplementary Methods.
Ethidium efflux assay
Induced cells (with 0.2 mM IPTG) of BW25113 ΔacrABΔemrEΔmdfA harbouring pTTQ18, pTTQ18-CraA_WT or craA mutants were harvested, washed twice with Dulbecco’s PBS (8 g/L NaCl, 0.2 g/L KCl, 1.44 g/L Na2HPO4.2H2O, 0.2 g/L KH2PO4, 10 mM MgCl2) and resuspended in the same buffer. Cells were incubated with 40 μM CCCP at 37°C for 10 min, before incubation with 10 μM (final concentration) of ethidium bromide for 45 min. To initiate efflux, 0.36% glucose was added to the suspension and the fluorescence was measured using Tecan Microplate Reader (Tecan, Switzerland) (excitation and emission wavelength of 535 and 610 nm, respectively).
Proton transport in everted membrane vesicles
The detection of proton transport into membrane vesicles was conducted as previously described21 with slight modifications. Briefly, 20 μL membrane vesicles (0.4 mg protein) was added into 1 mL of pre-warmed proton-transport-buffer supplemented with 1 μM 9-amino-6-chloro-2-methoxyacridine (ACMA). Fluorescence of ACMA was measured with excitation and emission wavelengths of 409 and 474, respectively. DL-Lactate (final concentration: 2 mM), tested drugs and CCCP (final concentration: 0.01 mM) were added at indicated timepoints. The detailed method is described in the Supplementary Methods.
Homology model of CraA
The homology model of CraA was created by I-TASSER based on MdfA_Q131R_L339E mutant (PDB: 6EUQ).31,32
Results
Identification of putative chloramphenicol transporters in A. baumannii AYE
Genome sequence analysis of AbAYE revealed the presence of five ORF-encoding putative MFS-type chloramphenicol transporters (CraA or CraAshort, ABAYE0685, ABAYE0913, CmlA5 and CmlA) on the basis of comparison with the known chloramphenicol transporter CraA (Figure 1a). Two different ORF annotations of CraA from AbAYE were deposited in the GenBank, where Genoscope ID 12_508 contains an additional 14 amino acids at the N-terminus compared with ABAYE0338.4,7 CraA shares 45% sequence identity (61% similarity) to MdfA and has been previously identified as a chloramphenicol efflux transporter.14In silico analysis of amino acid sequences showed that CmlA5 and CmlA share 100% sequence identity with a plasmid-encoded putative chloramphenicol/florfenicol transporter of Salmonella enterica and Klebsiella pneumoniae, respectively. A protein sequence homology search against the sequence database showed that ABAYE0913 and ABAYE0685 share 73% and 66% sequence identity with a putative Bcr/CflA family drug resistance efflux transporter of Alkanindiges illinoisensis and Pseudomonadales bacterium, respectively.
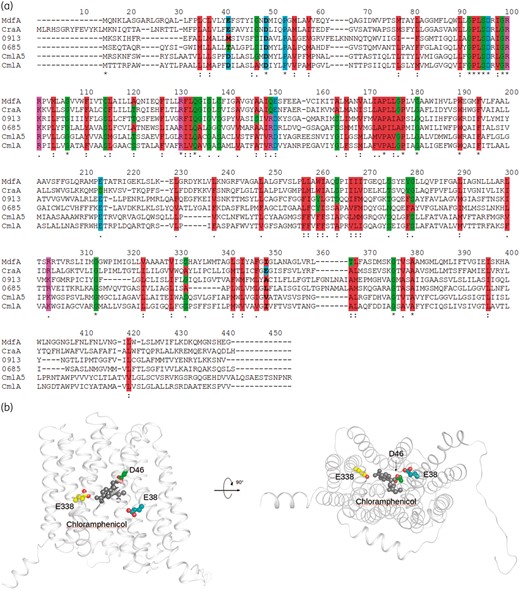
Protein sequence alignment of CraA and putative chloramphenicol transporters, and homology model of CraA. (a) Alignment of CraA, MdfA and other MFS-type chloramphenicol transporters. MdfA, MdfA of Escherichia coli; CraA, CraA of A. baumannii AYE (GenBank accession number: CAJ77876); CmlA, CmlA of A. baumannii AYE (GenBank accession number: CAM88409); CmlA5, CmlA5 of A. baumannii AYE (GenBank accession number: CAM88395); ABAYE0685, ABAYE0685 of A. baumannii AYE (GenBank accession number: CAM85649); ABAYE0913, ABAYE0913 of A. baumannii AYE (GenBank accession number: CAM85858). Membrane-embedded titratable residues are indicated in bold. Colour code for amino acids are shown as: hydrophobic residues = red; negatively charged residues or proline = cyan; hydrophilic residues or cysteine or glycine = green; positively charged residues = magenta. (b) Homology model of CraA was obtained based on the MdfA_Q131R_L339E variant (PDB: 6EUQ).32 CraA homology model cartoon representation is coloured in white. Indicated residues are depicted as spheres (carbon = green or teal or yellow; oxygen = red). Chloramphenicol obtained from the crystal structure of chloramphenicol-bound MdfA_Q131R (PDB: 4ZOW)35 is depicted as a sphere. This figure appears in colour in the online version of JAC and in black and white in the print version of JAC.
Characterization of putative chloramphenicol transporters in A. baumannii AYE
To access the substrate specificity of the putative chloramphenicol transporters of AbAYE, these ORFs were cloned into pTTQ18 and drug susceptibility assays were performed in E. coli BW25113 ΔemrEΔmdfA as host. CmlA was excluded from further studies due to the toxicity of protein production after induction with IPTG, which led to cell death as indicated on the control plate (Figure S2a). Consistent with previous results,14,craA- and cmlA5-expressing cells were resistant to chloramphenicol as compared with the negative control, whereas cells expressing ABAYE0913 and ABAYE0685 were susceptible to chloramphenicol. As MdfA is a well-known MDR transporter with promiscuous substrate specificity,20,21 we extended our experiments with chloramphenicol derivatives (thiamphenicol and florfenicol), monovalent cationic (benzalkonium) and LDC (dequalinium) drugs. Interestingly, cells expressing craA exhibited resistance to thiamphenicol, florfenicol, dequalinium, benzalkonium and a slight resistance to norfloxacin, but not to tetracycline or novobiocin (Figure S2). In addition, cells expressing cmlA5 exhibited a similar degree of resistance to thiamphenicol in comparison with craA-expressing cells but not to florfenicol. Intriguingly, ABAYE0913-expressing cells exhibited some resistance only against florfenicol and benzalkonium. As cells expressing craA or craAshort showed similar resistance profile trends, we continued our study with craA, which was reported by Fournier et al.7 (Figures S2a and S3).
Phenotypic analysis of A. baumannii 19606 ΔcraA and complementation
Expression of craA in E. coli cells led to resistance not only to chloramphenicol, but also to dequalinium, benzalkonium and other phenicols. Since these results indicated a more promiscuous substrate specificity as reported before,14 we addressed the CraA-mediated resistance to these substrates in A. baumannii. We therefore knocked out the craA gene from the chromosome of Ab19606 (the same strain used by Roca et al.14), and measured the drug susceptibility. Ab19606 WT strain, Ab19606 ΔcraA harbouring pBAV1K (empty vector) and Ab19606 ΔcraA harbouring pBAV1K_CraA grew to a similar extent on control plates. Ab19606 ΔcraA harbouring pBAV1K (empty vector) was susceptible to chloramphenicol, thiamphenicol and florfenicol (Figure 2a). The complementation of pBAV1K_CraA to the Ab19606 ΔcraA restored the resistance to these drugs, however, to a lesser extent in comparison to WT Ab19606. Surprisingly, all strains showed resistance to benzalkonium, chlorhexidine and dequalinium (Figure 2b), in contrast to the results obtained with the E. coli deletion strains (Figures S2 and S3). This observation suggests that other efflux systems and/or the outer membrane impermeability might be dominant factors for the resistance to these drugs in A. baumannii.
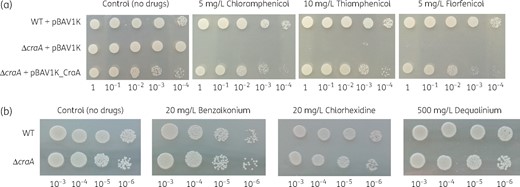
Drug resistance of A. baumannii WT, A. baumannii ΔcraA, and A. baumannii ΔcraA pBAV1K_CraA. (a) Overnight cultures of these strains were diluted to OD600 of 100–10−4 (given below the plate figures) and diluted cultures were spotted on LB agar plates supplemented with 50 mg/L kanamycin and selected drugs (5 mg/L chloramphenicol, 10 mg/L thiamphenicol and 5 mg/L florfenicol). (b) Overnight cultures of these strains were diluted to OD600 of 10−3–10−6 (given below the plate figures) and diluted cultures were spotted on LB agar plates supplemented with selected drugs (20 mg/L benzalkonium, 20 mg/L chlorhexidine and 500 mg/L dequalinium). Experiments were conducted at least three times and the result shown is representative. This figure appears in colour in the online version of JAC and in black and white in the print version of JAC.
Importance of membrane-embedded titratable residues of CraA
Sequence alignment and inspection of an MdfA-based (PDB: 6EUQ) homology model of CraA indicate that the membrane-embedded proton-titratable residues Glu-26 and Asp-34 (in MdfA) are conserved in CraA (Glu-38 and Asp-46 in CraA; Glu-24 and Asp-32 in CraAshort) (Figure 1).32–35 Interestingly, CraA contains an additional titratable residue, Glu-338 (Glu-324 in CraAshort; Ile-327 in MdfA) in the putative binding pocket (Figure 1b). To evaluate the importance of these residues for drug and/or H+ transport, we created alanine-substitution variants of CraA and performed drug susceptibility assays using E. coli BW25113 ΔmdfAΔemrE complemented with CraA and the CraA mutants. All craA mutants were expressed equally well compared with craA_WT from the same expression vector (Figure 3a). Our results indicated that cells expressing craA_E38A had no resistance against all tested substrates (Figure 3b). It is noteworthy that craA_D46A-expressing cells were resistant to a lesser extent to the phenicols and benzalkonium as compared with CraA_WT. In contrast, neutralization of Asp-46 completely abolished the efflux activity against mitomycin C and other cationic substrates including chlorhexidine, dequalinium and ethidium. As expected, neutralizing Glu-38 in the background of D46A completely abolished the resistance to all tested drugs. Interestingly, our data showed that the E338A variant conferred resistance to benzalkonium, ethidium and thiamphenicol, but was compromised in its ability to confer resistance to chloramphenicol, florfenicol, chlorhexidine, dequalinium and mitomycin C (Figure 3b). We hypothesized that the presence of the tripartite RND-type efflux pump AcrAB-TolC in E. coli BW25113 ΔemrEΔmdfA might affect the susceptibility to some of the tested drugs. To test this notion, we performed the drug susceptibility assay with E. coli BW25113 ΔacrABΔemrEΔmdfA lacking the periplasmic adaptor, AcrA and the RND component AcrB, as host. The results showed that craA_WT-expressing cells were resistant to all tested substrates and in addition to tetraphenylphosphonium (TPP+) as compared with cells carrying empty vector (Figure 3c). In this assay system, cells expressing craA_E38A were susceptible to all tested drugs, craA_D46A retained resistance to florfenicol and craA_E338A to thiamphenicol and benzalkonium and to a lesser extent to ethidium. CraA_WT conferred resistance to all tested drugs, confirming that this transporter is a multidrug efflux transporter. The difference in susceptibilities using either E. coli BW25113 ΔacrABΔemrEΔmdfA or BW25113 ΔemrEΔmdfA as host for craA or craA mutants (Figure 3b, c), can therefore be assigned to the activity of the AcrAB-TolC system in E. coli. Of note, the concentrations of the drugs used for the susceptibility test in the E. coli BW25113 ΔacrABΔemrEΔmdfA background were much lower compared with the tests using E. coli BW25113 ΔemrEΔmdfA, a well-known synergistic effect caused by the co-presence of the single-component transporters and AcrAB-TolC in E. coli (Figure 3b, c).26
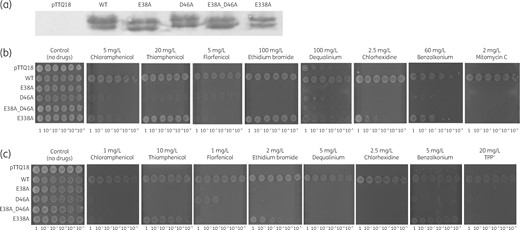
Characterization of CraA_WT and mutants. (a) Expression of various craA mutants was detected via Western blot analysis. The uncropped image of the Western blot analysis is shown in Figure S5. (b) E. coli BW25113 ΔemrEΔmdfA harbouring pTTQ18 (empty vector), pTTQ18_CraA_WT, and mutants were diluted to OD600 of 100–10−5 (given below the plate figures) and diluted cultures were spotted on LB agar plates supplemented with 0.2 mM IPTG, 100 mg/L ampicillin and selected drugs (5 mg/L chloramphenicol, 20 mg/L thiamphenicol, 5 mg/L florfenicol, 100 mg/L ethidium bromide, 100 mg/L dequalinium, 2.5 mg/L chlorhexidine, 60 mg/L benzalkonium and 2 mg/L mitomycin C). Experiments were conducted at least three times and the result shown is representative. (c) E. coli BW25113 ΔacrABΔemrEΔmdfA harbouring pTTQ18 (empty vector), pTTQ18_CraA_WT, and mutants were diluted to OD600 of 100–10−5 and diluted cultures were spotted on LB agar plates supplemented with 0.2 mM IPTG, 100 mg/L ampicillin and selected drugs (1 mg/L chloramphenicol, 10 mg/L thiamphenicol, 1 mg/L florfenicol, 2 mg/L ethidium bromide, 5 mg/L dequalinium, 2.5 mg/L chlorhexidine, 5 mg/L benzalkonium and 20 mg/L TPP+). Experiments were conducted at least three times and the result shown is representative.
Dequalinium transport in whole cells
Dequalinium is an MdfA substrate that contains two quinolinium moieties linked by an acyl chain.21,32 Accumulation of dequalinium in cells causes fluorescence quenching while dequenching occurs when dequalinium is removed from the cells. The fluorescence remained unchanged for E. coli BW25113 ΔacrABΔemrEΔmdfA cells expressing craA_WT, implying that dequalinium was constantly removed from the cells (Figure 4a). In contrast, dequalinium was accumulated in the cells harbouring empty vector, as evidenced by fluorescence quenching. Neutralization of Glu-38, Asp-46 or Glu-338 completely inhibits the extrusion of dequalinium from the cells. Furthermore, the removal of dequalinium from the cells harbouring craA_WT was impeded when chloramphenicol (CraA substrate) was added as a competitive substrate, as evidenced by the fluorescence quenching (Figure 4b). To evaluate whether the quenching of fluorescence is due to the accumulation of dequalinium in the cells, we lysed the dequalinium-loaded cells with SDS. A remarkable increase of the fluorescence was observed for cells harbouring empty vector or CraA mutants when the cells were incubated with dequalinium for 15 min as compared with 0 min (Figure 4c). In contrast, only a slight increase of fluorescence was observed for cells harbouring CraA_WT upon lysis as the intracellular accumulation of dequalinium in the cells was prevented during the 15 min incubation. These results are in line with the drug susceptibility assays implicating that Glu-38, Asp-46 and Glu-338 are important for the transport of dequalinium.
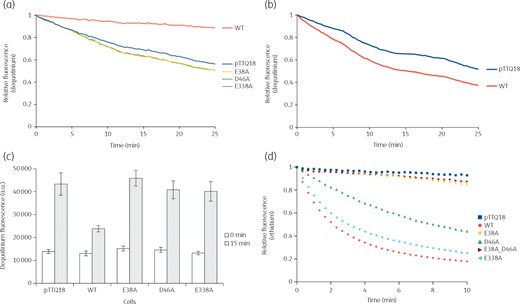
Active extrusion of dequalinium and ethidium. (a) Efflux of dequalinium by cells expressing craA_WT or craA mutants. Fluorescence quenching was observed for cells expressing craA mutants and cells harbouring pTTQ18 (empty vector), indicating an accumulation of dequalinium in the cells. In contrast, the fluorescence remained unchanged for cells expressing craA_WT, indicating a continuous extrusion of dequalinium by CraA. (b) Competition assay between dequalinium and chloramphenicol. Upon addition of chloramphenicol as a competitor, accumulation of dequalinium in the cells expressing craA_WT increased as indicated by fluorescence quenching. (c) Accumulation of dequalinium in the cells harbouring pTTQ18 (empty vector), pTTQ18_CraA_WT and mutants. Substantial increase of fluorescence was observed for cells expressing CraA mutants and cells harbouring pTTQ18 (empty vector) after incubation of cells with dequalinium for 15 min. In contrast, accumulation of dequalinium for cells expressing craA_WT is lower compared with the cells harbouring an empty vector, indicating a constant efflux of dequalinium from the cells. Experiments were conducted at least three times and the error bars represents SEM. Difference in dequalinium accumulation between cells expressing craA_WT and other CraA variants was statistically significant (P < 0.005). (d) Efflux of ethidium was monitored continuously by the fluorescence of ethidium binding to DNA in the cells. Initially, cells expressing craA_WT and mutants were starved with CCCP in the presence of ethidium bromide. Active efflux of ethidium was observed after re-energization (with 0.36% glucose) of the starved cells. Quenching of fluorescence indicates the removal of ethidium by CraA_WT, CraA_D46A or CraAE338A. Experiments were conducted at least three times and the result shown is representative. This figure appears in colour in the online version of JAC and in black and white in the print version of JAC.
Extrusion of ethidium by CraA efflux pump
Active efflux processes of MDR transporters can be easily monitored by whole cell fluorescence experiments with ethidium.17,32 Fluorescence of ethidium is significantly enhanced when binding to DNA and the fluorescence is quenched upon extrusion of ethidium by the efflux pump. Cells harbouring empty vector (negative control), craA_WT, or the craA mutants were starved in the absence of energy source, while in presence of ethidium and CCCP. Re-energization of cells by glucose causes a rapid decrease of fluorescence for craA_WT-expressing cells, corresponding to the active efflux of ethidium out of the cells (Figure 4d). In contrast, ethidium fluorescence remained unchanged for the negative control. Similar observations were found with cells expressing craA_E38A and craA_E38A_D46A, indicating a very low ethidium efflux activity. Neutralization of Glu-338 did not inhibit the removal of ethidium as the rate of the active efflux is similar to CraA_WT (Figure 4d), and confirms the survival of the craA_E338A expressing cells in the plate dilution assay (Figure 3b, c). An intermediate decrease of fluorescence was seen for cells expressing craA_D46A (Figure 4d).
Proton-coupled CraA substrate transport activity
The transport activity of H+-dependent secondary active transporters can be followed by the ΔpH-sensitive fluorescent dye, ACMA.34,36 To examine the substrate dependence of CraA-mediated H+-transport, transport experiments were conducted using everted membrane vesicles containing CraA_WT or each of the CraA variants. Upon addition of lactate to the outside of the everted membrane vesicles, protons are transported into the interior of the membrane vesicles, as evidenced by the ACMA fluorescence quenching. Addition of dequalinium, TPP+ or benzalkonium causes partial dissipation of the sensed ΔpH across the membrane of CraA-containing vesicles, leading to an increase in fluorescence (dequenching) (Figure 5). Unlike CraA-containing vesicles, no dissipation of the ΔpH was observed in control vesicles upon substrate addition, as indicated by the unchanged fluorescence signal. Addition of CCCP, a proton uncoupler (ΔpH and ΔΨ), causes an increase of the fluorescence due to the dissipation of the ΔpH. Our results indicate that CraA catalyses the transport of dequalinium, TPP+ and benzalkonium in exchange for protons. Addition of dequalinium and benzalkonium to CraA variants containing vesicles causes a very small increase of fluorescence, which was negligible compared with CraA_WT, indicating a significant decrease in the ability to transport dequalinium or benzalkonium (and protons) (Figure S4a, b). No change of ACMA fluorescence was observed upon addition of TPP+ to the CraA variants containing vesicles as compared with CraA_WT containing vesicles (Figure S4c), indicating a complete lack of transport activity.
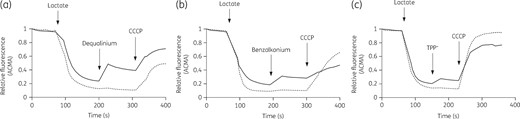
Proton-coupled substrate transport by membrane vesicles containing CraA. Lactate-driven proton pumping across the membrane establishes a transmembrane ΔΨ and ΔpH gradient causing interior acidification of the membrane vesicles as monitored by ACMA fluorescence quenching. Addition of CraA substrate: (a) dequalinium (50 μM), (b) benzalkonium (0.03 mg/L), or (c) TPP+ (250 μM), causes a partial dissipation of the ΔpH in the CraA_WT (solid line) containing membrane vesicles, as concluded from the dequenching of the ACMA fluorescence. In contrast, no fluorescence change was observed in control vesicles (prepared from cells harbouring pTTQ18, empty vector) (dotted line) upon addition of substrates. CCCP, a proton uncoupler, abolishes the ΔΨ and ΔpH, the latter dissipation causing an increase of fluorescence. Experiments were conducted at least three times and the result shown is representative.
Discussion
To date, at least six single-component transporters (AbaF, AbeM, AmvA, CraA, ABAYE0913 and ABUW_0982) and three RND tripartite systems (AdeABC, AdeEFG and AdeIJK) coexist in A. baumannii to confer resistance to chloramphenicol.11–13,15,18,37–39 Our study indicates that CraA, CmlA5 and ABAYE0913 confer resistance to chloramphenicol and other toxic compounds and their substrate specificities overlap (Figure S2). Along with the MDR transporters, drug modification systems such as chloramphenicol acetyltransferase might contribute in combination with efflux pumps to the intrinsic chloramphenicol resistance in A. baumannii,7,10,27,40 but on the other hand, high chloramphenicol resistance has also been observed in A. baumannii isolates lacking the acetyltransferase activity.27 We speculate that both CraA and RND-type systems including AdeABC, AdeFGH and AdeIJK, operate in synergy to expel chloramphenicol from the cells. This synergy is supported by our observation that craA-expressing cells in the ΔacrB (homologue of AdeB) background are more susceptible to the tested drugs as compared with cells expressing acrB (Figure 3b, c). These data are in line with previous reports that the synergistic transport system between single-component and multicomponent transporters are required to enhance the antibiotic resistance as shown in Pseudomonas and E. coli.26,41 Importantly, all these data imply that A. baumannii developed and/or acquired a robust survival mechanism to thrive in stringent environments such as hospitals. Multiple MDR determinants are beneficial for the cells to cope with rapid changes in these environments by regulating the MDR gene expression depending on the environmental stimuli. For example, the regulation of craA expression in A. baumannii is associated with the nutrient availability in the environment.42 Thus, it is interesting to speculate that these MFS-type chloramphenicol transporter genes in AbAYE are likely expressed conditionally depending on the environmental cues, to evade chloramphenicol stress.
All clinical A. baumannii isolates contain chromosomally encoded craA, which is responsible for the intrinsic chloramphenicol resistance. CraA of Ab19606 was reported to confer resistance to chloramphenicol,14 but not against other tested drugs. We tested the Ab19606 ΔcraA knock-out strain using substrates known to be transported by MdfA. We could confirm its role in chloramphenicol resistance, in addition to resistance to thiamphenicol or florfenicol. Whereas its ability to confer benzalkonium, chlorhexidine or dequalinium resistance could not be shown in the A. baumannii background, CraA appears to have the substrate specificity to transport these drugs, since when expressed in E. coli, resistance to these drugs and to ethidium or TPP+ could be shown.20,21 We speculate that synergistic effects with the outer membrane of A. baumannii and/or the presence of other transporters (such as AbeS) could result in the discrepancy observed.16,17,39 Mitomycin C is commonly used as a chemotherapeutic agent to treat bladder, gastric and pancreatic cancers; however, it has been shown to kill effectively persister A. baumannii, E. coli, Staphylococcus aureus and P. aeruginosa.43–45 Importantly, we observed that CraA confers resistance to mitomycin C, when overproduced in E. coli, although it has been proposed as an alternative for the treatment of A. baumannii infections.45 As CraA of Ab19606 is identical to CraA of A. baumannii ATCC 17978, our observation is completely in line with previous observations that this transporter confers a broad-spectrum substrate resistance phenotype when expressed in E. coli. In this work, we show that the substrates include phenicols, monovalent cationic and LDC drugs (Figure 3b, c).39
CraA contains three membrane-embedded potentially charged residues, Glu-38, Asp-46 and Glu-338, which might play an important role in the binding of substrates and/or protons. Previous studies indicated that Asp-34 of MdfA is important for protonation but is not essential for drug binding.34,46–48 Interestingly, both Glu-26 and Asp-34 are required for the transport of cationic drugs by MdfA but the extrusion of neutral drugs requires the presence of only one of these carboxylated residues.33,34,46,47,49,50 In contrast to the results observed for MdfA, our results indicate that Asp-46 (homologous to Asp-34 of MdfA) is important in the recognition of cationic drugs, as neutralization of Asp-46 completely abolished or showed little activity against cationic substrates but retained some resistance to neutral substrates (Figures 3b and c and Figure 4 and Figure S4). These results imply that Glu-38 in CraA might compensate for the absence of Asp-46 for transport of neutral substrates. We speculate that Glu-38 might be involved in the binding of proton and/or drug as neutralization of Glu-38 completely abolished the resistance against all substrates (Figures 3b and c and Figure 4 and Figure S4). Our results indicate furthermore that neutralization of Glu-338 imposes a mixed phenotype. It appears to be an important residue for the recognition of chloramphenicol, florfenicol, chlorhexidine and dequalinium, but not for thiamphenicol, ethidium or benzalkonium (Figure 3b, c). Altogether, our data highlights that both CraA and MdfA share similar substrate specificities but the role of the carboxylates might be different between CraA and MdfA.
It will be interesting to decipher the mode of action of CraA and ABAYE0913, both with a promiscuous substrate specificity but with different mutant phenotypes as compared with the well-studied MdfA from E. coli. It is also noteworthy that the redundancy of chloramphenicol resistance determinants is widely spread among A. baumannii isolates, stressing the ability of A. baumannii to develop strong resistance to this antibiotic.39 Further studies are needed to ascertain the relationships between chloramphenicol stress and the expression of chloramphenicol-related resistance genes. Importantly, elucidation of the environmental stimuli that trigger the MDR gene expression may reveal new drug targets against proteins (transcriptional regulators) involved in the activation of MDR gene expression.
Funding
This work was supported by a grant from the Deutsche Forschungsgemeinschaft through DFG Research Unit FOR 2251 ‘Adaptation and persistence of the emerging pathogen Acinetobacter baumannii’.
Transparency declarations
None to declare.