-
PDF
- Split View
-
Views
-
Cite
Cite
Benoît Desguin, Patrice Soumillion, Robert P. Hausinger, Pascal Hols, Unexpected complexity in the lactate racemization system of lactic acid bacteria, FEMS Microbiology Reviews, Volume 41, Issue Supp_1, August 2017, Pages S71–S83, https://doi.org/10.1093/femsre/fux021
- Share Icon Share
Abstract
Analysis of lactate racemase (Lar) in lactic acid bacteria (LAB) has been a scientific challenge for many years, as indicated by the numerous contradictory reports on this activity. Recently, genetic and biochemical studies of the Lar system of Lactobacillus plantarum have unveiled the complexity of this particular enzymatic system. Lar activity is associated with LarA and its nickel-containing cofactor, synthesized from nicotinic acid adenine dinucleotide by the three biosynthetic enzymes: LarB, LarC, and LarE. In addition to these core Lar enzymes, a nickel transporter (Lar(MN)QO), a lactic acid channel (LarD) and a transcriptional regulator (LarR) which promotes expression of the lar genes in the presence of excess L-lactate are also part of the Lar system of Lb. plantarum and of many other LAB. These proteins promote racemization of external L-lactate, in addition to carrying out intracellular racemization. This additional outcome suggests that racemization of L-lactate is not only required for cell wall biosynthesis, as reported before, but may have additional roles in lactate production and utilization in LAB. Finally, bioinformatics analyses indicate that some Lar homologs probably catalyze reactions other than lactate racemization.
INTRODUCTION
The lactic acid bacteria (LAB) are composed of microaerophilic, non-sporulating Gram-positive rods and cocci that are characterized by their common capacity to produce primarily lactate from hexose sugars. The definition of LAB is biological rather than taxonomic, i.e. the LAB do not comprise a monophyletic group of bacteria although most LAB group with the order Lactobacillales. LAB share a long history with humans as they are associated with mucosal surfaces such as the oral cavity, vagina and gastrointestinal tract, and they are present in all kinds of fermented food such as dairy products, meat, vegetables, sourdough bread and wine (Wood and Holzapfel 1995; Carr, Chill and Maida 2002; Wood and Warner 2003). The LAB include both important pathogens, e.g. several Streptococcus and Enterococcus species, and commensal LAB with beneficial roles in the maintenance of health and the prevention of infection, such as several Lactobacillus strains (Dunny, Cleary and McKay 1991; Dicks and Botes 2010).
The biological racemization of D- and L-lactate was first reported in 1936 for intact cells of Clostridium acetobutylicum and C. beijerinckii (formerly C. butylicum) (Tatum, Peterson and Fred 1936). One year later, lactate racemase (Lar) activity was shown in cell suspensions of Staphylococcus aureus (formerly S. ureae) and suggested to be present in Lactobacillus sakei (formerly Lb. sake), for which the production of lactate was shifted from L-lactate to DL-lactate depending on culture conditions (Katagiri and Kitahara 1937). Lar activity was later found in partially purified cell lysates of Lb. plantarum, which did not show any D- or L-lactate dehydrogenase (Ldh) activity (Kitahara, Obayashi and Fukui 1952). The discovery that nicotinic acid strongly influenced the Lar activity in vivo led to the suggestion that NAD might be the cofactor of Lar in Lb. plantarum (Kitahara and Obayashi 1955); this supposition was later reported to be the case in vitro when cell lysates from this microorganism were assayed (Kitahara and Obayashi 1959). As a result of these early studies, it was postulated that Lar activity of Lb. plantarum was not a single enzyme but a mix of both NAD-dependent D- and L-Ldh (Hiyama, Mizushima and Kitahara 1965) (Fig. 1).
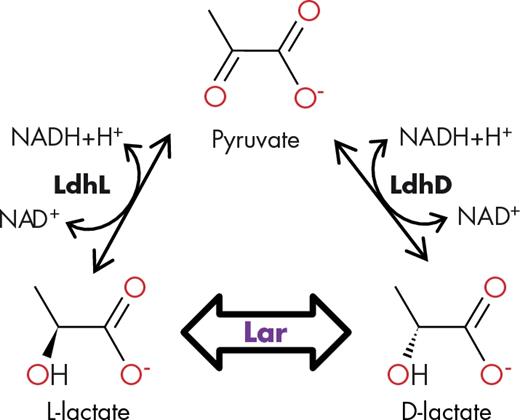
The lactate racemase activity. LdhL and LdhD: L- and D-lactate dehydrogenase, respectively. NAD: nicotinamide adenine dinucleotide. Lar: lactate racemase.
In 1961, the supposed racemase of C. acetobutylicum was purified 20-fold, shown to be inhibited by cyanide, and found to be stimulated by pyridoxamine phosphate and ferrous ions (Katagiri, Sugimori and Imai 1961). Light absorption near 325 nm in the partially purified enzyme suggested the participation of pyridoxamine phosphate in Lar, and comigration of an extracted compound with an ultraviolet spectrum similar to pyridoxamine phosphate appeared to corroborate this hypothesis (Katagiri, Sugimori and Imai 1961).
In Lb. sakei, the supposed Lar activity, which was shown to be induced by L-lactate but not by DL-lactate (Obayashi and Kitahara 1959), was purified 190-fold and characterized. The enriched Lb. sakei enzyme required no added cofactors, was reported to be quite small (25-kDa), exhibited no spectral features beyond that at 274 nm from aromatic residues, possessed an optimum pH of 5.8–6.2, and had similar kinetic parameters for the D- and the L-isomers (Km of 17 mM with kcat of 16.7 s−1 for D-lactate and Km of 8 mM with kcat of 6.7 s−1 for the L-isomer) (Hiyama, Fukui and Kitahara 1968).
In comparison, the Lar purified from C. beijerinkii showed distinct properties. Its size was estimated at 82.4 kDa, activity was detected from pH 4.5–8.5 with an optimum at 5.5, the Km values were around 8 mM for both D- and L-lactate, and inhibition by hydroxylamine was relieved by adding pyruvate (Dennis and Kaplan 1963; Cantwell and Dennis 1974).
A few additional studies of Lar activity have been reported. The activity was confirmed to be present in S. aureus (Stockland and San Clemente 1969), detected in several other Lactobacillus species (Stetter and Kandler 1973), found in the rumen bacteria Selenomonas ruminantium and Megasphaera elsdenii (Melville, Michel and Macy 1988; Hino and Kuroda 1993), and identified in halophilic archaea (Oren and Gurevich 1995). Also, disruption of the gene encoding the sole (L-specific) Ldh in Lb. sakei prevented the formation of both L- and D-lactate, consistent with the proposed cellular role for Lar in this microorganism, which is the formation of D-lactate from L-lactate (Malleret et al. 1998).
In summary, early studies of Lar activity in various microorganisms provided conflicting evidence that depended to some extent on the source of the enzyme. Preliminary conclusions from this work are that three different Lar systems may be present: a mixture of L- and D-Ldh in Lb. plantarum, an iron- and pyridoxamine phosphate-dependent enzyme in C. acetobutylicum, and a cofactor-independent enzyme in Lb. sakei. As will become clear below, these preliminary conclusions are unlikely to be valid. For example, authentic Lar activity was later demonstrated in Lb. plantarum when a strain deleted of its gene for D-Ldh was shown to produce DL-lactate (Ferain et al. 1996). In this review, we will report recent studies on the Lar system of Lb. plantarum that have considerably advanced our understanding of this enzyme. After providing a detailed description of the lar system in Lb. plantarum, a global bioinformatics analysis of lar loci in Lactobacillales genomes will shed light on the possible functions of lactate racemization in LAB and suggest that homologous enzymes may isomerize other substrates than lactate.
The Lar system of Lactobacillus plantarum
Lactobacillus plantarum has both L-Ldh and D-Ldh, so it would appear to not require a Lar enzyme. Nevertheless, when the gene encoding its D-Ldh was inactivated, the organism was shown to use its Lar for supplying D-lactate for cell wall synthesis (Ferain et al. 1996; Goffin et al. 2005). Importantly, D-lactate is the terminal residue of the muramoyl-pentadepsipeptide peptidoglycan precursor and confers natural resistance to vancomycin in this microorganism (Walsh 1993; Ferain et al. 1996; Goffin et al. 2005). Moreover, the presence of D-lactate is essential for Lb. plantarum growth since it cannot be replaced with D-alanine by the cell wall machinery (Ferain et al. 1996; Goffin et al. 2005).
Nine lar genes for the lactate racemization system
Two adjacent gene clusters (larABCDE and larR(MN)QO), transcribed in opposite directions, have been shown to be associated with Lar activity in Lb. plantarum (Fig. 2a) (Goffin et al. 2005; Desguin et al. 2014). The enzyme itself is encoded by larA, but activity requires coexpression of larB, larC and larE (Desguin et al. 2014), the roles of which will be described later in this review. The larD gene is not required for racemase activity, but it encodes an aquaglyceroporin which channels both lactic acid (protonated lactate) isomers through the membrane (Fig. 2b) (Bienert et al. 2013). The larMN, larO and larQ genes encode an ATP-binding cassette (ABC) transporter system for high-affinity nickel uptake (Zhang et al. 2009) and are needed for activity when the medium is not supplemented with high concentration of this metal ion (Fig. 2c) (Desguin et al. 2014). The larABCDE gene cluster is induced by L-lactate that triggers the binding and multimerization of the transcriptional regulator LarR on a central palindromic DNA sequence (called the Lar-box), while D-lactate acts as a repressor, probably by preventing L-lactate binding (Fig. 2d) (Desguin et al. 2015a). A few other genes have been shown to be regulated by an excess of L-lactate, e.g. citrate lyase (repressed) and acetyl-CoA carboxylase (induced) (Desguin et al. 2014), which suggests that LarR could also be involved in the regulation of other functions than lactate racemization
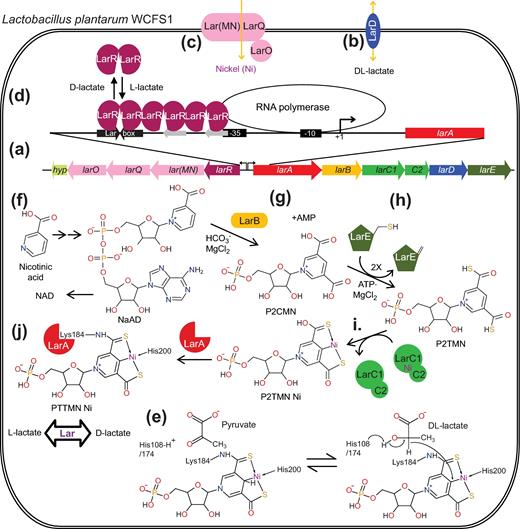
The Lar system of Lb. plantarum WCFS1. (a) Genetic organization of the Lb. plantarum WCFS1 lar locus. (b) LarD is a lactic acid membrane channel. (c) Lar(MN)QO imports nickel into the cell. (d) The larABCDE promoter is stimulated by LarR binding and multimerization on the Lar box, which depends on the presence of L-lactate and the absence of D-lactate. (e) Hypothetical mechanism of Lar. PTTMN Ni: pyridinium-3-thioamide-5-thiocarboxylic acid mononucleotide nickel pincer. (f) NAD biosynthesis pathway. NaAD: nicotinic acid adenine dinucleotide, NAD: nicotinamide adenine dinucleotide. (g) LarB is a NaAD hydrolase/carboxylase. P2CMN: pyridinium-3,5-dicarboxylic acid mononucleotide, AMP: adenosine monophosphate. (h) LarE is an ATP-dependent sacrificial sulfur transferase. P2TMN: pyridinium-3,5-dithiocarboxylic acid mononucleotide. (i) LarC is a nickel chelatase. (j) Ni PTTMN insertion into LarA.
Lar cofactor: a nickel-pincer complex
Nickel is required for Lb. plantarum Lar activity and the purified LarA protein contains this metal ion (Desguin et al. 2014), thus bringing the number of known nickel enzymes to nine (Boer, Mulrooney and Hausinger 2014). Unfortunately, nickel dissociates from the enzyme with rapid loss of activity (Desguin et al. 2014); however, sulfite, a reducing agent and potent mixed inhibitor of Lar, prevents nickel loss and stabilizes the holoenzyme when added during enzyme purification (Desguin et al. 2015b). Mass spectrometric analyses revealed the presence of a covalently tethered, niacin-derived, nickel-containing cofactor in the protein, and the crystal structure of LarA revealed its unique structural features (Desguin et al. 2015b). The catalytic site of LarA possesses a derivatized nicotinic acid mononucleotide in which the pyridinium ring is linked to Lys184 by replacing its C3 carboxylate with a thioamide and a thiocarboxylate is added at C5. The bound nickel ion is coordinated in a near-planar arrangement by the thioamide sulfur atom, the pyridinium C4 carbon, the thioacid sulfur atom and the ε nitrogen of His200 (Fig. 2e) (Desguin et al. 2015b). Previously, C-Ni bonds had only been associated with catalytic intermediates (as opposed to the resting form) of the nickel-containing methyl-coenzyme M reductase, and carbon monoxide dehydrogenase (Harmer et al. 2008; Lindahl 2009; Amara et al. 2011). The LarA metallocenter resembles pincer complexes (in this case a Ni(SCS) pincer complex, S for sulfur and C for carbon) that are widely used in inorganic synthesis and organometallic catalysis (Singleton 2003; Koten and Milstein 2013), and thus was named pyridinium-3-thioamide-5-thiocarboxylic acid mononucleotide nickel pincer (PTTMN Ni). This is the first pincer complex observed in an enzyme (Desguin et al. 2015b). Moreover, whereas pyridine ligands had been utilized in synthetic pincer complexes, a positively charged pyridinium in para orientation relative to the C-metal bond had not been previously described (Xu, Bauer and Hu 2016).
Lar catalytic mechanism: a hydride transfer reaction?
The presence of PTTMN Ni in LarA is consistent with a plausible mechanism in which a hydride is transferred from the α-carbon of lactate to the pyridinium ring, followed by hydride transfer back to the pyruvate intermediate. Two histidinyl residues (His108 and His174) are well positioned to facilitate the hydride transfer reaction by acid-base catalysis on either side of the substrate, which is thought to be positioned where a sulfate is present in the structure (Fig. 3), possibly abstracting the proton of the alcohol function of L- or D-lactate and donating a proton to the keto group of the pyruvate intermediate (Fig. 2e) (Desguin et al. 2015b). In order for the lactate racemization to occur, the α-keto group of the pyruvate intermediate has to shift from one catalytic histidine to the other by rotation of the C1-C2 bond. This enzymatic reaction mechanism was supported by a computational study that showed the C-Ni bond and the distortion imposed by hydride addition increases the energy of the reduced PTTMN Ni intermediate, promoting racemization rather than dehydrogenation (Zhang and Chung 2017). Without such an unusual mechanism to destabilize the intermediate, the thermodynamics of the reaction would drive the equilibrium towards the pyruvate and the reduced cofactor. A analog of the PTTMN Ni cofactor was chemically synthesized and shown to mediate dehydrogenation of alcohols, but demetallation occurred after a single turnover and prevented further reaction (Xu et al. 2017).
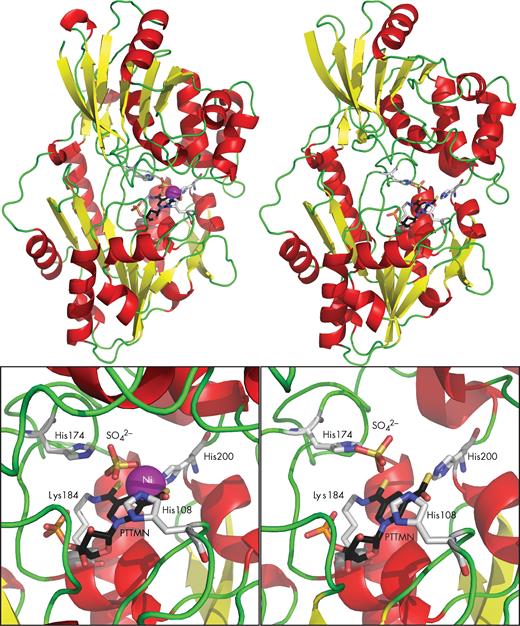
LarA structure. Comparison of the Ni-containing monomer (left) with the Ni-free monomer (right). The LarA structure is shown in cartoon mode with the α-helices in red, β-sheets in yellow and loops in green. The catalytic residues and the pincer cofactor are shown in stick mode with the carbons in black for the cofactor and in light gray for the protein residues, nitrogen in blue, oxygen in red, sulfur in yellow and phosphorus in orange. Ni is shown as a purple ball. Sulfate occupies the predicted position of lactate in the catalytic site. PTTMN: pyridinium-3-thioamide-5-thiocarboxylic acid mononucleotide.
Lar structural aspects
The LarA structure revealed a homodimeric enzyme composed of two 46.2-kDa monomers exhibiting substantial differences in conformation. In one monomer, the C-terminal domain approaches the N-terminal domain, leading to a closed conformation where the Ni-containing catalytic site is well sealed and fully separated from the exterior. In contrast, the other monomer exhibits a ∼12° outward rotation of the C-terminal domain, resulting in an open conformation where the catalytic site is accessible to bulk solvent and is accompanied by loss of nickel (Fig. 3). Distortion of the nickel-coordinating geometry conceivably leads to a reduced stability of the PTTMN Ni, accounting for the absence of nickel from the tethered cofactor in the open conformation. Considering that the substrate must have access to the catalytic site, it is very likely that LarA in solution switches between the open and the closed conformations for catalysis. Nevertheless, such a dynamic process must be rapid enough so that the catalytically essential nickel would not be lost from the catalytic center. Given the usually high concentration of lactate (around 500 mM) in LAB during fermentation (Carvalho et al. 2013) and the KM for LarA substrates (∼11 mM for D-lactate and 46 mM for L-lactate) (Desguin et al. 2014), the catalytic site of LarA must be mostly saturated with substrate and in the closed conformation in vivo.
Biosynthesis of the nickel pincer complex PTTMN Ni: a novel metabolic pathway
Activation of LarA requires the in vivo coproduction of the three biosynthetic enzymes LarB, LarC and LarE (Desguin et al. 2014). No pair of biosynthetic enzymes copurifies, indicating that the proteins do not strongly interact; this behavior is distinct from that reported for the accessory proteins required for the maturation of urease, the best-characterized nickel enzyme (Farrugia, Macomber and Hausinger 2013). Thus, LarB, LarC and LarE appear to participate independently in the biosynthesis of the PTTMN Ni and form a new metabolic pathway. This new pathway starts with an intermediate of the nicotinamide adenine dinucleotide (NAD) biosynthesis pathway, nicotinic acid adenine dinucleotide (NaAD) (Fig. 2f), explaining why Lar activity shows a strong dependence on the nicotinic acid concentration in the medium (Kitahara and Obayashi 1955; Desguin et al. 2015b).
LarB is a 25.3-kDa protein whose C-terminal domain is homologous to PurE, an aminoimidazole ribonucleotide carboxylase/mutase (Tranchimand et al. 2011). We have shown that LarB carboxylates NaAD in the presence of bicarbonate and Mg2+. Interestingly, LarB also catalyzes hydrolysis of the phosphoanhydride linkage of NaAD, resulting in pyridinium-3,5-dicarboxylic acid mononucleotide (P2CMN) and adenosine monophosphate (Fig. 2g) (Desguin et al. 2016). Because NaAD, but not nicotinic acid mononucleotide, is the substrate of LarB, it may be postulated that hydrolysis of the phosphoanhydride linkage is necessary for carboxylation.
Sulfur addition into the cofactor requires LarE, a member of the PP-loop superfamily (Desguin et al. 2014). This enzyme of 30.5 kDa is suggested to first activate a carboxylic group of P2CMN by adenylylation in an ATP-dependent manner, similar to NaAD activation by the homologous enzyme NAD synthetase (Sorci et al. 2009). LarE then uses its Cys176 to form a thioester with the activated substrate, releasing AMP. Deprotonation of the Cys176 alpha carbon leads to release of the thiocarboxylic acid and formation of dehydroalanine at position 176 of LarE. A second LarE-dependent reaction will then transform the pyridinium-3-carboxylic acid-5-thiocarboxylic acid mononucleotide product into the final pyridinium-3,5-dithiocarboxylic acid mononucleotide (P2TMN). LarE is therefore consumed by the reaction and two LarE proteins must undergo sacrificial desulfurization in order to synthesize one P2TMN from one P2CMN (Fig. 2h) (Desguin et al. 2016).
LarC is a nickel-binding protein that exhibits two isoforms: the 28.7-kDa LarC1 is encoded by larC1 and the 46.4-kDa LarC1C2 is derived from a fusion between larC1 and larC2, arising from a programmed ribosomal frameshift (Giedroc and Cornish 2009), and which was shown to be the active isoform (LarC) (Desguin et al. 2014). LarC has been shown to be capable of inserting nickel into P2TMN, forming the pyridinium-3,5-dithiocarboxylic acid mononucleotide nickel pincer (P2TMN Ni) (Fig. 2i) (Desguin et al. 2016). This reaction could happen while the cofactor is still bound to LarE, explaining why LarA apoprotein can be activated by LarE alone when it is co-produced with LarB and LarC (Desguin et al. 2014).
The cofactor is attached to LarA apoprotein, generating LarA-PTTMN Ni, the active Lar enzyme (Fig. 2j). It remains to be assessed whether the condensation of P2TMN Ni with Lys184 of LarA, generating the thioamide linkage, is spontaneous or if additional activation is required.
Occurrence of lar genes in microorganisms
An analysis of over 1000 genomes revealed a wide distribution of lar-like genes among bacteria and archaea. The four genes required for Lar activity (larA, larB, larC and larE) are present in 9% of the analyzed genomes, including many lactate consumers. Thus, Lar may allow consumption of both D- and L-lactate in cells that contain a single Ldh. Strikingly, over 15% of the genomes analyzed encode the Lar cofactor biosynthetic enzymes (larB, larC and larE) while lacking a larA homolog. This observation suggests that this cofactor may be utilized by one or more proteins other than LarA homologs (Desguin et al. 2015b). Some species harbor several larA paralogs, possibly suggesting that other substrates than lactate could be used by other LarA enzymes (Desguin et al. 2014).
As described above, early studies had reported some disparate aspects of Lar from C. acetobutylicum, C. beijerinckii and Lb. sakei. Genomic sequencing efforts, however, have revealed the presence of larA, larB, larC and larE in these species, thus strongly suggesting they also contain a PTTMN Ni-containing LarA enzyme similar to that of Lb. plantarum (Desguin et al. 2014).
The five-gene operon for Lar encodes the enzyme subunit (larA), the cofactor biosynthetic enzymes (larB, larC and larE) and the lactic acid channel (larD), and is only observed in LAB, which compose only a fraction of the genomes harboring the four genes required for Lar activity and represent <1% of the analyzed genomes (Desguin et al. 2014). These bacteria also contain the transcriptional regulator LarR that expresses the lar genes when L-lactate is present in enantiomeric excess (Desguin et al. 2015a). It remains unclear, however, whether the larD and larR genes are always linked to lactate racemization in LAB.
Occurrence of lar genes in LAB
From previous in silico studies, it appeared that the genetic content of lar genes in LAB is usually composed of the core Lar genes (larA, larB, larC and larE) with the addition of larD and larR (Desguin et al. 2014, 2015a), but one can ask is this the case for all LAB? To better investigate the diversity of lar loci in LAB, a more extensive bioinformatics study was carried out.
Distribution of larA genes
The Lactobacillales order, which encompasses most LAB (Wood and Holzapfel 1995), contains more than 400 species with available genomes; these sequences were analyzed for the presence of larA genes (Table 1) (Table S1, Supporting Information). The greatest number and largest percentage of larA genes are found in the Lactobacillaceae family, with a quarter of species harboring larA. In all other families, larA genes are present in <10% of species. In the Aerococcaceae family, not a single larA gene was found (Table 1). In conclusion, larA genes are not more common in Lactobacillales order than among other prokaryotes (Desguin et al. 2014), with the notable exception of the Lactobacillaceae family, which shows a much higher occurrence of larA genes.
Family . | No. of . | No. of species . | Species with . |
---|---|---|---|
. | species . | with larA . | larA (%) . |
Aerococcaceae | 19 | 0 | 0 |
Carnobacteriaceae | 41 | 3 | 7 |
Enterococcaceae | 50 | 3 | 6 |
Lactobacillaceae | 181 | 45 | 25 |
Leuconostocaceae | 33 | 3 | 9 |
Streptococcaceae | 79 | 7 | 9 |
Total | 403 | 61 | 15 |
Family . | No. of . | No. of species . | Species with . |
---|---|---|---|
. | species . | with larA . | larA (%) . |
Aerococcaceae | 19 | 0 | 0 |
Carnobacteriaceae | 41 | 3 | 7 |
Enterococcaceae | 50 | 3 | 6 |
Lactobacillaceae | 181 | 45 | 25 |
Leuconostocaceae | 33 | 3 | 9 |
Streptococcaceae | 79 | 7 | 9 |
Total | 403 | 61 | 15 |
Family . | No. of . | No. of species . | Species with . |
---|---|---|---|
. | species . | with larA . | larA (%) . |
Aerococcaceae | 19 | 0 | 0 |
Carnobacteriaceae | 41 | 3 | 7 |
Enterococcaceae | 50 | 3 | 6 |
Lactobacillaceae | 181 | 45 | 25 |
Leuconostocaceae | 33 | 3 | 9 |
Streptococcaceae | 79 | 7 | 9 |
Total | 403 | 61 | 15 |
Family . | No. of . | No. of species . | Species with . |
---|---|---|---|
. | species . | with larA . | larA (%) . |
Aerococcaceae | 19 | 0 | 0 |
Carnobacteriaceae | 41 | 3 | 7 |
Enterococcaceae | 50 | 3 | 6 |
Lactobacillaceae | 181 | 45 | 25 |
Leuconostocaceae | 33 | 3 | 9 |
Streptococcaceae | 79 | 7 | 9 |
Total | 403 | 61 | 15 |
Genetic organization of lar loci
To assess the diversity in genetic organization of lar loci, 20 representative species of the Lactobacillales order were analyzed. The species were chosen to reflect the diversity of lar loci in this order, with 12 loci selected from Lactobacillaceae, 2 from Enterococcaceae, 2 from Leuconostocaceae, 2 from Streptococcaceae, 1 from Carnobacteriaceae and 1 from Aerococcaceae (Fig. 4) (Table S2, Supporting Information).
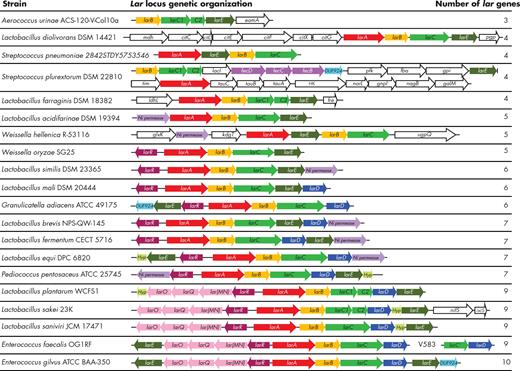
Genetic organization of 20 representative lar loci of Lactobacillales. For E. faecalis, two strains, OG1RF and V583, are represented on the same line. The larR, larA, larB, larC, larD and larE genes are shown in burgundy, red, yellow, green, blue and dark green, respectively. Genes encoding the ABCtype nickel transport system (lar(MN)QO), ABC-type Fe3+-siderophore transport system (fecBCD) and nickel permeases (Ni permease) are shown in pink, purple and light purple, respectively. Hypothetical genes (Hyp) and DUF924-encoding genes (DUF924) are shown in light green and light blue, respectively. Genes encoding an EamA family transporter (eamA), malate dehydrogenase (mdh), citrate lyase ligase (citC), citrate lyase subunits γ, β and α (citD, citE and citF, respectively), apo-citrate lyase phosphoribosyldephospho-CoA transferase (citX), phosphoglycolate phosphatase (pgp), a transcriptional regulator of the LacI/PurR family (lacI), 6-phosphofructokinase (pfk), fructose-bisphosphate aldolase (fba), glucose-6-phosphate isomerase (gpi), triose-phosphate isomerase (tim), ABC-type nitrate/sulfonate/bicarbonate transport system (tauABC), signal transduction histidine kinase (HK), DNA-binding response regulator of the NarL/FixJ family (narL), glucosamine-6-phosphate isomerase (gnpI), N-acetylglucosamine-6-phosphate deacetylase (nagB), galactose mutarotase (galM), L-lactate dehydrogenase (ldhL), NADPH-dependent FMN reductase (fre), glycerate kinase (glxK), 2-keto-3-deoxygluconate permease (kdgT), glycerophosphoryl diester phosphodiesterase (ugpQ), cysteine sulfinate desulfinase/cysteine desulfurase or related enzyme (nifS) and cysteine desulfurase (iscS) are shown in white.
The number of lar genes associated with these loci ranges from 3 for Aerococcus urinae to 10 for Enterococcus gilvus. The larB, larC and larE genes are found in all loci and larA is only absent in the A. urinae locus. A larABCE operon, with the genes in this order, is present in 16 out of 20 lar loci. Fifteen representatives possess a single-ORF larC, whereas five include two ORFs (i.e. LarC1+LarC2). This distribution means that the synteny is generally conserved for the core lar genes (larABCE) and that the programmed ribosomal frameshift of larC is not a general feature (Fig. 4).
The gene encoding the transcriptional regulator, larR, is found in 13 lar loci. This gene is always found in opposite orientation and next to a larA gene, suggesting that LarR regulates these lar loci as shown in the case of Lb. plantarum (Desguin et al. 2015a). The aquaglyceroporin-encoding gene, larD, is affiliated with 11 lar loci and always co-occurs with larR (Fig. 4). Coupling between lactate racemization and transport is reasonable in these bacteria, with LarD transporting lactic acid across the cell membrane as Lar catalyzes intracellular lactate racemization. Because LarD operates in both directions, these reactions will also lead to the racemization of extracellular lactate.
Nickel is transported passively by high-affinity nickel permeases encoded in seven lar loci, whereas active uptake by nickel ABC transporters is encoded in five other loci (Fig. 4). Intriguingly, one lar locus possesses genes coding for homologs of the iron dicitrate transporter FecBCDE (Staudenmaier et al. 1989) (Fig. 4). This observation either suggests that a FEC-type transporter may also be able to transport nickel or that some homologs of Lar may be able to use iron instead of nickel.
Genes encoding a domain of unknown function, DUF924, are found in three lar loci. This finding suggests that this uncharacterized protein may have a function related to lactate racemization or to the PTTMN Ni biosynthetic pathway. Moreover, genes encoding small hypothetical proteins are found associated with five lar loci, although they do not seem to be related to each other (Fig. 4).
The presence of genes coding for cysteine desulfurases in one lar locus leads us to speculate that desulfurized LarE may be regenerated in vivo in Lb. sakei. By contrast, when LarE is overproduced in Lactococcus lactis the dehydroalanine side chain does not appear to be recycled to cysteine (Desguin et al. 2016) despite the presence of cysteine desulfurase genes in this bacterium.
Roles of Lar in LAB
The ratio of L-/D-lactate produced by LAB differs widely from one species to another and can even be used as a criterion for species identification (Manome et al. 1998). The L-/D-lactate ratio in LAB is mainly determined by the NAD-dependent Ldhs (Zheng et al. 2012), by Lar and to a lesser extent by hydroxyisocaproate dehydrogenases (Rico et al. 2008); yet the reason why this ratio varies so much from one LAB to another and the precise role that Lar is playing in the determination of this ratio remain unknown.
D-Lactate production
As the end product of fermentation, LAB need to eliminate lactate from their cells in order to prevent a slow down of their Ldh enzymes due to product accumulation. Excretion of D- and L-lactic acid in Lb. plantarum seems to be enhanced by the lactic acid channel LarD, as shown by studies in which a strain deficient in its lactic acid channels exhibited a delayed growth during exponential phase, especially under conditions of lactate stress (Bienert et al. 2013). For LAB like Lb. sakei which have only L-Ldh (Malleret et al. 1998), Lar allows for the production of D-lactate from L-lactate and could partially relieve product inhibition of L-Ldh. This effect must be very limited, however, because no difference in final L- or D-lactate production was observed for Lb. helveticus, Lb. johnsonii and Lb. plantarum strains producing a single isomer compared to the wild-type strains producing DL-lactate (Liu 2003). D-Lactate is required for cell wall biosynthesis by Lb. plantarum (Ferain et al. 1996) and it is probably required for many other LAB as well (see below); thus, Lar can serve as an alternative pathway for D-lactate synthesis in LAB that lack D-Ldh activity. Furthermore, LAB producing racemic mixtures of lactate in some growth conditions may change their pattern of isomer production under different conditions (Liu 2003). For example, the D-Ldh-encoding gene of Lb. delbrueckii ssp. bulgaricus was downregulated during acid adaptation (Fernandez et al. 2008). Lactobacillus plantarum produced less L-lactate and more D-lactate under acidic conditions or in conditions of nicotinic acid limitation (Kitahara and Obayashi 1955; Bobillo and Marshall 1992), whereas in conditions of zero growth the D-Ldh-encoding gene is downregulated while lar genes are upregulated in Lb. plantarum (Goffin et al. 2010). Lactobacillus casei, on the other hand, produced more D-lactate under conditions of glucose limitations (Liu 2003). Several Lb. sakei strains became L-lactate producers in the presence of acetate, probably due to the inhibition of Lar under this condition, whereas Lb. coryniformis subsp. coryniformis became a D-lactate producer under the same conditions (Iino et al. 2001). These examples show that many growth conditions and enzyme activities can influence the production and balance of the lactate isomers and they demonstrate that there is no simple predictive rule. As a generalization, it is probably best for bacteria to have multiple routes for producing D-lactate, if this isomer is required for growth, but it is not yet clear why LAB would change their isomeric pattern depending on the environmental parameters.
There is one condition in which the type of lactate isomer produced is not influenced by the Ldh specificity(ies). This situation involves malolactic fermentation, where L-malate is directly converted to L-lactate by the malolactic enzyme (Miller et al. 2011). Under these conditions, Lar can be used for the production of D-lactate directly from L-lactate, as reported in several wine and cider fermentations (Liu 2003) and in Lb. plantarum growing in the absence of lactic acid fermentation (Ferain et al. 1996).
D-Lactate utilization
D- and L-Lactate are not only end products of fermentation by LAB, but they may also be further metabolized by some LAB in the presence of oxygen or some other electron acceptor such as oxaloacetate or 3-hydroxypropionaldehyde (Liu 2003). For example, Lb. plantarum is able to convert DL-lactate to acetate in aerobic conditions (Goffin et al. 2004). This bacterium could use the combination of D-Ldh and Lar to consume all L-lactate present in conditions where the L-Ldh is repressed.
If D-lactate is not directly used by a bacterial community, the extent of its production may influence other lactate-consuming organisms. For example, because mammals metabolize L-lactate much better than D-lactate, the racemization of L-lactate to DL-lactate by the microbiome in the gut may cause D-lactate acidosis (Seheult et al. 2017). At the microbial level, the production ratio of lactate isomers generated by fermentation may influence the lactate-consuming microorganisms. For example, the lactate oxidizer Gluconobacter oxydans, the metal-reducer Shewanella oneidensis, the cyanobacterium Synechocystis sp. PCC6803 and several strains of butyrate-producing bacteria were shown to preferentially consume D-lactate over L-lactate (Sato et al. 2008; Brutinel and Gralnick 2012; Angermayr et al. 2015; Sheng et al. 2015). Likewise, a general study in several soil microbial communities clearly showed that D-lactate is the preferred isomer of lactate consumers (Moazeni, Zhang and Sun. 2010). When considering the general scheme of lactate metabolism, the Lar activity of Lactobacillaceae may be the link between L-lactate producing LAB (Carr, Chill and Maida 2002) and D-lactate consumers. The lactate-racemizing bacteria may even benefit energetically if a hypothetical transporter of L-lactate (i.e. acting on the non-protonated species) was present in these species. In this scenario, the bacteria would import the anionic L-lactate, racemize it to DL-lactate and export D-lactic acid thus generating a proton gradient across the cell membrane. If the concentration of D-lactate is kept low by lactate consumers and the concentration of L-lactate is high, this process may account for significant generation of a proton gradient that can be used for ATP synthesis, similar to the mechanism used in malolactic fermentation where the decarboxylation of malate consumes intracellular protons (Kolb et al. 1992).
Lar activity and vancomycin resistance
In Lb. plantarum, it was hypothesized that Lar is used as a rescue pathway for the production of D-lactate for cell wall biosynthesis, thus conferring resistance to the antibiotic vancomycin (Goffin et al. 2005). Intrinsic resistance to vancomycin may be achieved by substituting a conserved tyrosine with a phenylalanine in D-alanine:D-alanine ligase, changing it to a D-alanine:D-lactate ligase (Park and Walsh 1997). This substitution is found in 80% of Lactobacillaceae species and is consistent with the known vancomycin resistance pattern (Swenson, Facklam and Thornsberry 1990; Carr, Chill and Maida 2002; Salminen et al. 2006; Karapetkov et al. 2011; Goldstein, Tyrrell and Citron 2015). Among the larA-containing Lactobacillaceae, this proportion goes up to 98% with only one species combining vancomycin susceptibility with the presence of a larA gene, i.e. Lb. delbrueckii subsp. indicus. Among the other Lactobacillaceae species, the occurrence of vancomycin resistance drops to 74% (Table S3, Supporting Information). This result means that lactate-racemizing species are typically vancomycin resistant, but not all vancomycin-resistant strains harbor lar genes. It is likely that LAB that have evolved towards D-lactate incorporation into their peptidoglycan retain the lar genes in order to secure their supply in D-lactate.
The most common type of vancomycin resistance found in human pathogens is the resistance acquired through the horizontal transfer of van genes, responsible for D-lactate production and incorporation into the cell wall (Ranotkar et al. 2014). However, the presence of lar genes in Enterococcus faecalis (Fig. 4) suggests that lactate racemization may play a role in the formation of D-lactate in acquired vancomycin resistance. To investigate this possibility, the genomes of 36 E. faecalis strains with different pathogenic features and from different body habitats were analyzed in silico for their lar gene content (Table 2) (Table S4, Supporting Information) (Bakshi et al. 2016).
E. faecalis strainsa . | No. of strains . | No. of strains with van operon . | No. of strains with lar operon . |
---|---|---|---|
Vancomycin-resistant pathogenic | 6 | 6 | 0 |
Vancomycin-sensitive pathogenic | 5 | 0 | 1 |
Commensal or uncharacterized | 25 | 0 | 6 |
Blood | 16 | 5 | 2 |
Gastrointestinal tract | 12 | 1 | 5 |
Other | 8 | 0 | 0 |
E. faecalis strainsa . | No. of strains . | No. of strains with van operon . | No. of strains with lar operon . |
---|---|---|---|
Vancomycin-resistant pathogenic | 6 | 6 | 0 |
Vancomycin-sensitive pathogenic | 5 | 0 | 1 |
Commensal or uncharacterized | 25 | 0 | 6 |
Blood | 16 | 5 | 2 |
Gastrointestinal tract | 12 | 1 | 5 |
Other | 8 | 0 | 0 |
Collection of 36 E. faecalis strains described by Bakshi et al. (2016).
E. faecalis strainsa . | No. of strains . | No. of strains with van operon . | No. of strains with lar operon . |
---|---|---|---|
Vancomycin-resistant pathogenic | 6 | 6 | 0 |
Vancomycin-sensitive pathogenic | 5 | 0 | 1 |
Commensal or uncharacterized | 25 | 0 | 6 |
Blood | 16 | 5 | 2 |
Gastrointestinal tract | 12 | 1 | 5 |
Other | 8 | 0 | 0 |
E. faecalis strainsa . | No. of strains . | No. of strains with van operon . | No. of strains with lar operon . |
---|---|---|---|
Vancomycin-resistant pathogenic | 6 | 6 | 0 |
Vancomycin-sensitive pathogenic | 5 | 0 | 1 |
Commensal or uncharacterized | 25 | 0 | 6 |
Blood | 16 | 5 | 2 |
Gastrointestinal tract | 12 | 1 | 5 |
Other | 8 | 0 | 0 |
Collection of 36 E. faecalis strains described by Bakshi et al. (2016).
From this analysis, it appeared that for all strains containing van operons, the complete lar operon was absent. It is striking to observe that the lar operon is limited to a truncated larC and a larD in pathogenic strains which contain a van operon, e.g. E. faecalis V583 (Fig. 4) (Huo et al. 2015), compared to van-free strains, such as E. faecalis OG1RF, where a complete lar locus is observed (Fig. 4). Because vanH codes for a D-Ldh, the presence of the lar genes is probably no longer required in these strains. When the same 36 E. faecalis strains are sorted depending on their isolation sites, it appears that the lar genes are more often found in the gastrointestinal tract than at other sites (Table 2). Thus, it seems that lactate racemization in E. faecalis is more closely linked to the niche of the gastrointestinal tract than to vancomycin resistance. This hypothesis is strengthened by proteomic studies which showed that LarA homologs are ubiquitous in healthy human stool samples (Chatterjee et al. 2016).
The LarA family in LAB: more than lactate racemization?
Interestingly, there are several lar loci which lack larR and larD (Fig. 4). To analyze whether these lar loci all code for closely related Lar proteins, the amino acid sequences of the core Lar enzymes of the 20 representative lar loci were aligned (Fig. 5). From these alignments, it appears that six LarA sequences diverge strongly from the other LarA sequences, whereas no similar divergence is observed in the LarB, LarC or LarE phylogenetic trees (Fig. 5). Strikingly, these six LarA sequences correspond to the only lar loci lacking larR (Fig. 4). Moreover, many of these six lar loci are associated with other genes that are unrelated to lactate racemization such as the citrate lyase genes in Lb. diolivorans or fructose-bisphosphate aldolase in Streptococcus plurextorum (Fig. 4). We hypothesize that these LarA homologs may not racemize lactate, but rather we suggest they may catalyze racemization or isomerization reactions using other related substrates, a hypothesis that in our opinion deserves further investigation.
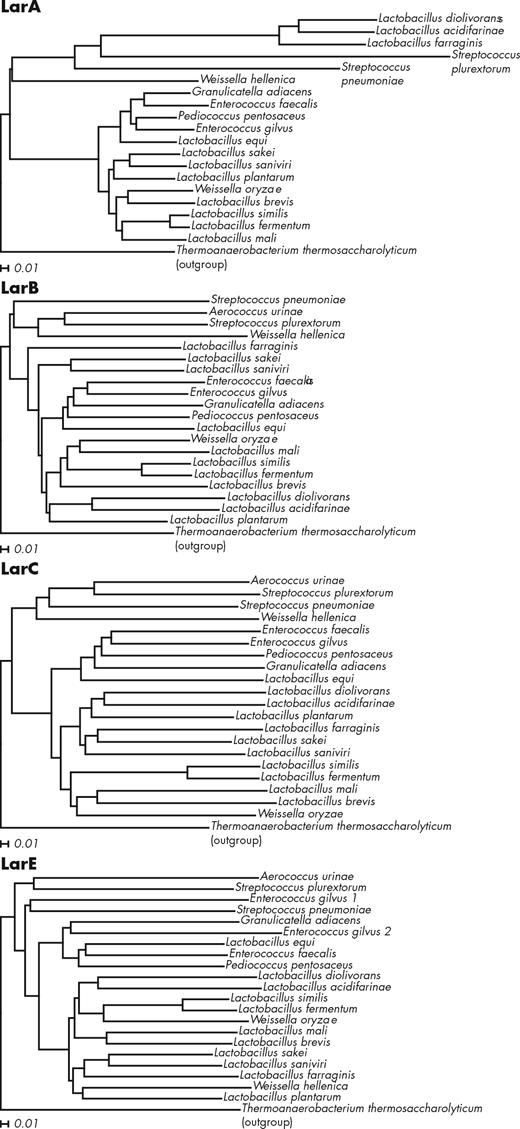
LarA, LarB, LarC and LarE alignments with Lar proteins of Thermoanaerobacterium thermosaccharolyticum DSM 571 as outgroups. Strain names are those reported in Fig. 4. The alignments were created with ClustalX using the neighbor-joining method (Larkin et al. 2007) and the trees were drawn with Dendroscope (Huson and Scornavacca 2012) using default parameters. The distances shown represent the substitutions per sequence position.
CONCLUDING REMARKS
The Lar system exhibits several levels of complexity. First, the biosynthesis of the PTTMN Ni cofactor requires a new biosynthetic pathway of three enzymes that catalyze unusual reactions. For example, LarB both carboxylates the 5-position of the pyridinium ring of NaAD and hydrolyzes the phosphoanhydride linkage. Direct nucleophilic attack of the pyridinium ring on CO2 cannot occur because it would generate a second positive charge. One plausible mechanism to overcome this charge buildup is to first eliminate the charge of the ring by nucleophile attack or hydride reduction, attack of C5 on the CO2, and completing the reaction by eliminating the nucleophile or hydride. This complex mechanism potentially could be driven by the energy released during hydrolysis of the NaAD phosphoanhydride linkage, either by activating the substrate, similarly to four enzymes in the purine biosynthesis pathway which use phosphate transfer from ATP to activate a carboxylate group (Kappock, Ealick and Stubbe 2000), or by protein structural changes that facilitate carboxylation. Either way, the mechanism of LarB reaction is unique.
Concerning LarE, the carboxyl group activation mechanism is relatively straightforward and resembles the activation mechanism of its homolog NAD synthetase (Sorci et al. 2009). The difference here is that LarE also forms a covalent adduct with the substrate and is then used as substrate for sulfur donation. Sulfur-containing cofactors are typically synthesized with the sulfur atom of free cysteine through the action of a persulfide-forming cysteine desulfurase (Black and Dos Santos 2015), but here the sulfur comes from a cysteinyl group of the protein itself. Direct sulfurization from a protein cysteinyl group, also generating a dehydroalanine residue, has been reported in the case of thiamine thiazole synthase of Saccharomyces cerevisiae, THI4p (Chatterjee et al. 2011). Similarly to THI4p, the sulfur-depleted LarE does not appear to be recycled back to its original form when overexpressed in Lactococcus lactis (Desguin et al. 2016); however, this may not be the case under physiological low expression levels in Lactobacillus plantarum or in another species. It is conceivable that under the right conditions, the dehydroalanine formed in LarE could react with a persulfide generated by a cysteine desulfurase and return to its cysteinyl form in vivo (Black and Dos Santos 2015), as suggested by the presence of genes encoding cysteine desulfurases in one lar locus (Fig. 4). This LarE-recycling reaction would prevent the bacteria from having to sacrifice two LarE proteins for the synthesis of one P2TMN.
The LarC reaction is still very mysterious; LarC is the only enzyme known to be able to form a stable Ni-C bond and the reaction mechanism by which it achieves nickel insertion is unknown. As a possible precedent, the pathway for biosynthesis of coenzyme F430 was shown to require a nickel chelatase that inserts Ni2+ into the porphyrin ring without the need of a cofactor (Zheng et al. 2016). Nevertheless, because the PTTMN Ni cofactor is unstable and tends to lose its nickel ion (Desguin et al. 2014) it is possible that LarC could require an energy input of some sort in order to insert the nickel into P2TMN and form the C-Ni bond; this would explain why LarC is absolutely required for activation of Lar activity both in vivo and in vitro (Desguin et al. 2014, 2016). The coordination environment of nickel when bound to LarC is unknown.
A second level of complexity centers on the other lar accessory genes. The role of nickel transporters is quite clear, as nickel is required for the Lar activity. LarR and LarD seem to participate in related functions, as they are generally present together (Fig. 4). LarR is responsible for the sensing of an enantiomeric excess of L-lactate and triggering the expression of the lar genes in consequence. By passive transport of both lactic acid enantiomers across the cell membrane, LarD assures a fast racemization of extracellular lactate. In some Lactobacillaceae species, this extracellular racemization could be used as a rescue pathway for the production of D-lactate when the D-Ldh activity is low.
However, it looks like taking a sledgehammer to crack a nut, as only 0.5 mM of D-lactate is enough to restore growth of a Lb. plantarum strain no longer producing D-lactate (Goffin et al. 2005). Vancomycin-resistant LAB which produce mainly L-lactate as their product of fermentation, e.g. Lb. casei, are able to produce small amounts of D-lactate using D-hydroxycaproate dehydrogenase, without the need for lactate racemization (Manome et al. 1998). These species seem to have another rescue pathway for production of D-lactate, as deletion of the D-hydroxycaproate dehydrogenase-producing gene in Lb. casei resulted in a strain still able to produce some D-lactate (Rico et al. 2008). Nevertheless, in conditions where no lactic acid fermentation is operational, like during malolactic fermentation or at zero growth, lactate racemization is likely the only way to produce D-lactate.
In D-lactate producers, such as Weissella oryzae (Tohno et al. 2013), lar genes would only be expressed in the presence of an excess of externally produced L-lactate, and in Enterococcus faecalis, lar genes seem more linked to the gastrointestinal niche than to vancomycin resistance. In these species, and in others, extracellular racemization of lactate may serve other purposes than producing D-lactate for the cell wall biosynthesis. For example, such racemization may conserve some of the lactate produced away from L-lactate consumers while fostering the growth of D-lactate consumers, and, potentially, gaining some energy from the racemization of extracellular L-lactate. This last hypothesis requires the expression of an L-lactate transporter, potentially encoded by some hypothetical protein present in many lar operons (Fig. 4). As a conclusion, we are just beginning to envision the many possible functions and roles in environmental adaptation of lactate racemization in LAB A third level of complexity is attained when we look at the distribution of the lar genes, not only in Lactobacillales but among prokaryotes in general. The bioinformatics analyses of the lar loci in Lactobacillales lead us to suggest that some LarA homologs may catalyze different reactions and have functions distinct from lactate racemization. The observation that some possess as many as four genes encoding LarA-like proteins in their genomes strengthens this hypothesis. Other proteins, completely unrelated to LarA, might also use the PTTMN Ni cofactor to catalyze yet other reactions. These many hypotheses offer new fields of investigation that are now open for exciting research.
SUPPLEMENTARY DATA
Supplementary data are available at FEMSRE online.
FUNDING
This work was supported by the Belgian National Fund for Scientific Research (FNRS) (to PH and PS), the Université catholique de Louvain-Fonds Spéciaux de Recherche (to P.H. and P.S.), and by the National Science Foundation Grant CHE-1516126 (to RPH).
Conflict of interest. None declared.