-
PDF
- Split View
-
Views
-
Cite
Cite
Guus A.M. Kortman, Manuela Raffatellu, Dorine W. Swinkels, Harold Tjalsma, Nutritional iron turned inside out: intestinal stress from a gut microbial perspective, FEMS Microbiology Reviews, Volume 38, Issue 6, November 2014, Pages 1202–1234, https://doi.org/10.1111/1574-6976.12086
- Share Icon Share
Abstract
Iron is abundantly present on earth, essential for most microorganisms and crucial for human health. Human iron deficiency that is nevertheless highly prevalent in developing regions of the world can be effectively treated by oral iron administration. Accumulating evidence indicates that excess of unabsorbed iron that enters the colonic lumen causes unwanted side effects at the intestinal host–microbiota interface. The chemical properties of iron, the luminal environment and host iron withdrawal mechanisms, especially during inflammation, can turn the intestine in a rather stressful milieu. Certain pathogenic enteric bacteria can, however, deal with this stress at the expense of other members of the gut microbiota, while their virulence also seems to be stimulated in an iron-rich intestinal environment. This review covers the multifaceted aspects of nutritional iron stress with respect to growth, composition, metabolism and pathogenicity of the gut microbiota in relation to human health. We aim to present an unpreceded view on the dynamic effects and impact of oral iron administration on intestinal host–microbiota interactions to provide leads for future research and other applications.
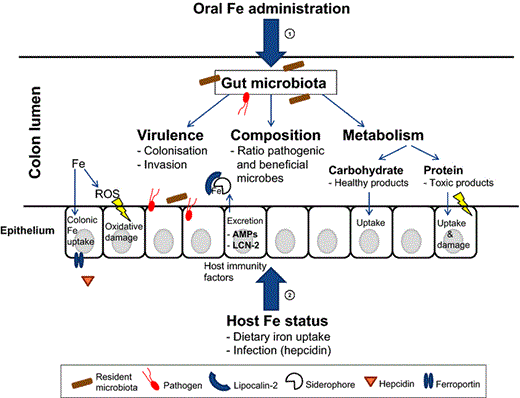
Oral iron administration, host iron status and intestinal inflammation can have a large impact on the gut microbiota composition, metabolism and virulence. This review comprehensively highlights the multifaceted aspects of nutritional iron stress within the colon lumen.
Summary and scope of this review
Iron is a highly abundant metal on earth and is vital for virtually all organisms, including most bacterial species. Nonetheless, iron deficiency is the most prevalent human nutrition disorder worldwide and is generally treated by oral iron administration. Notably, accumulating evidence suggests that unabsorbed iron can stimulate growth and virulence of bacterial pathogens in the intestinal environment. Simultaneously, host iron status influences the local defence against pathogens. Salmonella enterica serovar Typhimurium, a highly prevalent intestinal pathogen around the world, is one example of a pathogenic bacterial species that can deal with the stress of iron limitation, iron abundance and host defence factors. It is able to adapt to these environmental triggers and exploit the environment to thrive at the expense of other bacterial species. In this review, we will thoroughly discuss many different factors that are involved in iron-mediated host–pathogen interactions in the intestinal tract at the molecular level. We will first give a concise introduction in gut microbiology and nutritional stress. The central part of this review will present current knowledge about the effects of luminal iron on gut immunology and on the gut microbiota with respect to bacterial composition, pathogenicity and metabolism. These and other factors will also be related to pathogen stress in the gut lumen and are summarised in Fig. 1. Furthermore, we will mostly focus on S. Typhimurium and its ways to deal with environmental stress and to turn the external situation in favour of its own growth and colonisation. Finally, we will place the topics we discussed in the context of serious threats that persist in tropical Africa, such as infectious diarrhoea and S. Typhimurium infection that is often associated with invasive disease in this region.
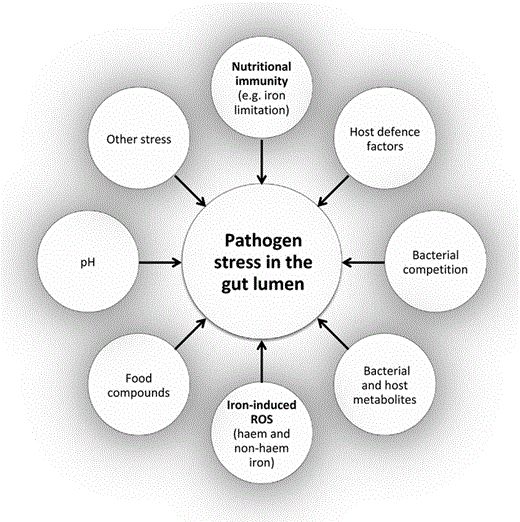
Overview of stress factors towards pathogens in the colon lumen. Schematic summary of the stress factors to which pathogens in the colon lumen are exposed. This is an incomplete list, and only the main factors discussed in this review are depicted.
General introduction
Gut microbiology and health
The human gut is the natural habitat for a unique large and dynamic bacterial community that greatly outnumbers human enterocytes. Major functions of the gut microbiota include important trophic effects on intestinal epithelia, immune structure and function, and protection of the colonised host against invasion by pathogenic microorganisms via the production of trophic metabolites and immunostimulatory molecules (Flint et al., 2007; Stecher & Hardt, 2008; Abreu, 2010; Hooper et al., 2012; Macfarlane & Macfarlane, 2012). The healthy gut microbiota is a balanced community that is shaped by host immunity and metabolism and environmental factors (Sekirov et al., 2010). Perturbations (e.g. antibiotic treatment and/or overgrowth of enteric pathogens) of the balanced gut microbiota have been associated with gastrointestinal disorders such as diarrhoea, gastroenteritis and chronic inflammatory bowel disease (IBD) (Sekirov et al., 2010). The number of studies investigating the effect of diets on the mammalian gut microbiota composition and metabolism has increased greatly during the past years and revealed that the gut microbiota is clearly influenced by the host diet (Power et al., 2014). It is currently not well defined whether the type of diet can significantly contribute to host health or disease via alteration of the gut microbiota composition.
Factors that shape the gut microbiota
Colonisation of the human gastrointestinal tract starts during birth and is known to be influenced by the place and mode of delivery (Penders et al., 2006). Known factors that influence gut colonisation after birth are among others hospitalisation, hygiene and the type of feeding (breast feeding or formula feeding) (Del Chierico et al., 2012). In later life, for example diet, hygiene, illness and aging will influence the gut microbial composition (Power et al., 2014). Importantly, the gut microbiota composition is shaped not only by environmental factors, but also by genetic factors (e.g. genes related to host immunity [see also section Effect of host iron intake on gut physiology and immunology)] and is thus always a result of a combination of the two. This leads to highly variable interindividual gut microbiota profiles. The relative contribution of these factors to the shaping of the gut microbiota composition is not well known yet (Spor et al., 2011). An interesting study of De Filippo et al., in which the gut microbiome of European children was compared with the gut microbiome of children living in African rural area, showed large differences between the groups. In this study, ethnicity and many environmental factors were different, but it was suggested that diet played the dominant role over other variables (De Filippo et al., 2010).
Nutritional and metabolic stress in the gut lumen
The gut microbial community thrives on complex carbohydrates and proteins that are not digested and taken up by the upper intestine. The balanced community also depends on the provision of micronutrients for metabolism and replication. There is a continuous competition for these nutrients, which is reflected in the different requirements and uptake mechanisms that certain species possess. Metabolic competition and interactions are also ways in which the gut microbiota restrains the growth of unwanted pathogens (Kamada et al., 2013). One of the micronutrients that the vast majority of bacterial species require for their growth and metabolism is iron (Andrews et al., 2003). However, in the human body, iron availability to microorganisms is generally extremely limited, due to innate iron withholding mechanisms that aim to prevent growth of pathogenic invaders (Cassat & Skaar, 2013). Such a tightly regulated system of nutritional immunity is not known for the lumen of human gut. However, also in the gut lumen with high amounts of dietary iron present, gut microorganisms have to deal with the stress of iron limitation as the presence of freely available ‘unbound’ iron is probably limited due to the environmental conditions of the colon lumen. The balance of bound and unbound iron can be (re)disturbed by the oral administration of supplementary iron, a common strategy to treat iron deficiency, which is known to cause alterations of the gut microbiota composition and metabolism (see section and ). In Fig. 1, we summarised the (iron-related) stress factors that will be discussed throughout this review.
Although most knowledge on the effects of iron in the gut originates from studies on the colonic microbiota, we predict that the effects of iron availability on the small intestinal microbiota are likely to be different from the effects on the colonic microbiota. It is important to realise that the small intestinal microbiota has a much lower density of residing microorganisms and that its composition and metabolism appears to fluctuate quickly in response to dietary input (Zoetendal et al., 2012). Iron may play a large role within this environment where also pathogenic bacteria like Salmonella spp., Vibrio cholera and pathogenic Escherichia coli may colonise. However, relatively little is known about the small intestinal microbiota, as it is much less accessible compared with the colonic (faecal) microbiota, which makes it difficult to study (Zoetendal et al., 2012). Therefore, the focus of this review is on the colonic microbiota to which we refer to as ‘gut microbiota’.
Redox stress and microorganisms
The redox chemistry of iron
Under physiological conditions, iron is found mostly in the ferrous (Fe2+) or ferric (Fe3+) form and the easy redox cycling properties of this metal make it very well suited as a biocatalyst in proteins or as an electron carrier (Andrews et al., 2003; Crichton, 2009). Under aerobic physiological conditions, iron is mostly present in its oxygenic ferric, virtually insoluble, form, with 10−9 M being the approximate concentration of ferric ion at pH 7 (Crichton, 2009). Fe3+ is practically only soluble in water when it is attained in a strong aqueous complex. Thus, despite the high abundance of iron on earth, its bioavailability can be very low. Fe3+ prefers oxygen ligands, while Fe2+ favours nitrogen and sulphur ligands (Crichton, 2009). Ferrous iron is much more soluble than ferric iron, but it is mainly found in anoxic or acidic environments. Importantly, free redox active iron can be very toxic under aerobic conditions via the formation of reactive oxygen species (ROS), generated through the Fenton and Haber–Weiss reaction (Fig. 2). Of the ROS, one of the most reactive species known is the highly reactive hydroxyl ion OH−, which can induce severe damage to cells and biological molecules.
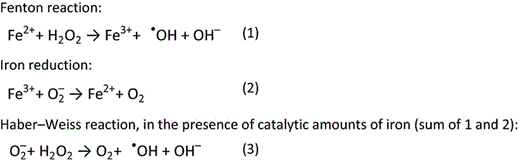
The Fenton and Haber–Weiss reaction. Ferrous iron generates the hydroxyl radical and hydroxyl ion from hydrogen peroxide (1). The superoxide radical (to be formed from dioxygen) can then reduce ferric iron back to the ferrous form (2). The sum of both reactions is therefore described as the formation of molecular oxygen, hydroxyl radicals and hydroxyl anions from the superoxide radical and hydrogen peroxide (3) (Crichton, 2009).
Bacteria and redox stress
The vast majority of life on earth, including most microorganisms in the bacterial kingdom, depends on the presence of the transition metal iron for respiration and various metabolic processes. Only a very limited number of bacteria are known that do not depend on iron for their growth. The most well-known and notable group concerns the beneficial Lactobacillaceae, but also the pathogenic Borrelia burgdorferi does not use iron for its metabolism, both are dependent on manganese instead (Pandey et al., 1994; Imbert & Blondeau, 1998; Posey & Gherardini, 2000). In addition, certain species of Streptococci that normally use iron can utilise manganese as an alternative transition metal (Jakubovics & Jenkinson, 2001). Furthermore, bacteria that utilise iron have to avoid the toxicity of free redox active iron both intracellularly and extracellularly. To this purpose, bacteria possess several mechanisms to detoxify iron or oxidative stress directly, of which a selection is described below.
Detoxification of iron
Iron in its ferric form can be bound to three known types of bacterial high-affinity storage proteins: ferritin (similar to what is found eukaryotes), bacterioferritin and DNA-binding protein from starved cells (DPS). DPS is an iron detoxification/iron storage protein that also binds to DNA and plays hereby a role in the protection of DNA from redox stress (Andrews et al., 2003). These iron storage proteins lower the concentration of intracellular free iron by storing it in a soluble and nontoxic form. Iron can be released from these bacterial stores in times of iron scarcity, for example when residing inside mammalian host cells or in the blood stream (Andrews et al., 2003).
Alternatively, bacteria can export a surplus of iron. Escherichia coli can, for example, export iron via the exporter FieF, and an iron–citrate efflux transporter (IceT) has been described for S. Typhimurium; these iron efflux systems have the supposed purpose to prevent stress from high levels of free intracellular iron (Grass et al., 2005; Frawley et al., 2013). Also, a haem export mechanism (HrtAB or orthologous proteins) has been shown to alleviate the iron stress of haem in certain bacterial species (Cassat & Skaar, 2013). Finally, Bifidobacteriaceae are able to bind iron to their surface, which subsequently reduces radical formation in the surrounding environment and may in the colonic lumen function as a mechanism of iron sequestration to prevent iron uptake by pathogenic species (Kot & Bezkorovainy, 1999) (see also Fig. 3).
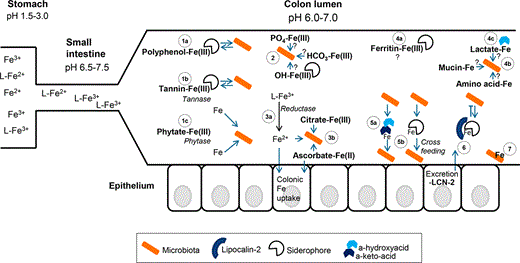
Iron speciation, availability and the battle for iron in the colon lumen. Iron speciation in the gastrointestinal tract probably plays an important role in the accessibility of iron for the gut microbiota. In the stomach (on the left; very simplified), the low pH favours the solubility of both ferric and ferrous iron, not necessarily requiring a ligand (L) for solubility. When the pH increases in the small intestine, the solubility of mainly ferric iron decreases and more complexes with food components and host excretions will be formed. Within the colon, the pH slightly drops due to the production of, for example, lactate and SCFAs by the microbiota. The colonic part of the figure, where the microbiota depicted in orange represents both (beneficial) resident species and pathogenic species, shows the following aspects: (1) On the left, it is shown that iron bound to polyphenols (1a), for example tannins (1b) and phytate (1c), may be accessible via enzymatic degradation or via the removal of the iron by siderophores; (2) The rather insoluble forms like iron in a complex with phosphate, carbonate or oxides are likely not readily accessible for the microbiota, but they may be solubilised by bacterial reduction or siderophoric chelation; (3) Soluble forms of ferric iron may be reduced to ferrous iron after which it can be taken up by both the microbiota and the host (3a), and for instance, the soluble ferric citrate or ferrous ascorbate may be directly taken up by bacteria (3b). Notably, host iron uptake may be a mechanism to withdraw the iron from the colon lumen; (4) On the right, several forms of iron are depicted from which we also do not know very well how accessible they are. We envisage that ferritin–iron (dietary or from sloughed enterocytes) (4a) is difficult to access, but iron bound to mucin, amino acids or lactate (4b) may be relatively easy to access and that may hypothetically be promoted by the low-affinity siderophores α-hydroxyacids and α-keto-acids (4c); (5) These low-affinity siderophores (5a) may also play an important role in the phenomenon of iron cross-feeding by heterologous siderophores within the microbiota (5b); (6) The excretion of lipocalin-2 (LCN-2) in the colon lumen may scavenge iron bound to siderophores and hereby prevent uptake by (pathogenic) bacteria; (7) Finally, at the lower right, it is depicted that bacteria spp. may bind iron to their cell wall (as has been shown for Bifidobacterium), which likely prevents access to this iron by other (pathogenic) species.
Protective enzymes that neutralise oxidants
Several bacterial enzymes are known that directly counteract the formation of oxidative stress. One of these enzymes is superoxide dismutase (SOD), which catalyses the dismutation of superoxide into the less toxic oxygen and hydrogen peroxide. Notably, the various members of the SOD family use different metals as a cofactor for functioning, among which are manganese and zinc. Host sequestration of manganese and zinc by calprotectin (excreted by immune cells) can therefore inhibit this bacterial defence mechanism and this way making bacteria more susceptible for oxidative stress (Damo et al., 2012) (See also section ). Another well-known enzyme that neutralises ROS is catalase, which catalyses the reaction of two hydrogen peroxide molecules to the nontoxic products water and oxygen. Finally, bacteria also possess peroxidases that can contribute to the oxidative stress defence. Peroxidases include glutathione peroxidase and alkylhydroperoxide reductase (Ahp) that can rapidly detoxify hydrogen peroxide, hydroperoxides or peroxynitrite (Crichton, 2009; Mishra & Imlay, 2012).
Iron, a precious and contested nutrient
Bacterial iron uptake
Although iron is present in sufficient amounts to sustain their replication in most habitats, bacteria still have to deal with the low solubility of this metal. Therefore, readily available iron is generally scarce and bacteria have evolved multiple iron uptake mechanisms to be able to fulfil their average need of 10−7–10−5 M for optimal growth. Several mechanisms of iron uptake were previously described for various bacterial species and were most extensively studied in E. coli. In the latter bacterium, iron can be taken up in both its ferrous and its ferric form. One of the mechanisms of ferrous iron uptake is via the Feo-uptake system (Andrews et al., 2003). Moreover, ferric iron can be reduced to ferrous iron via an extracellular reductase, after which it can be taken up in the ferrous form (Cowart, 2002). Alternatively, ferric iron can be taken up as ferric citrate or bound to excreted bacterial siderophores, small iron chelators with a very high affinity for iron (Andrews et al., 2003; Miethke & Marahiel, 2007). After excretion, bacteria re-internalise the ferric–siderophore complex via binding to specific receptors and transporters. For example, the ferric–enterobactin complex binds to the outer membrane receptor FepA of gram-negative bacteria, after which it is transported to the periplasm, a process dependent on the TonB system that provides the energy for this transport. Next, the ferric–enterobactin complex is further transported into the cytoplasm by ABC transporters (Chu et al., 2010). The role of siderophores in the colonic lumen is further described in section . Direct uptake of haem-bound iron and scavenging of haem with haemophores are other specialised ways of iron acquisition (Andrews et al., 2003). It is important to mention that haem iron is much more bioavailable for humans compared with nonhaem iron (Zimmermann & Hurrell, 2007), that haem causes oxidative and cytotoxic stress in the gut lumen and that haem can alter the (mouse) gut microbiota composition (IJssennagger et al., 2012, 2013). In this review, we will, however, focus on the effects of nonhaem iron on the growth, virulence and metabolism of gut microorganisms because dietary iron on average consists for 90% of nonhaem iron (Hulten et al., 1995), which percentage is likely even higher in the tropics. Furthermore, oral iron is always given as nonhaem iron.
Iron and bacterial virulence
It has been known for a long time that the scarcity of iron generally limits bacterial growth, whereas iron availability often enhances bacterial replication and the expression of virulence factors in pathogenic bacteria, thereby exaggerating infection (Payne & Finkelstein, 1978; Weinberg, 1978; Litwin & Calderwood, 1993; Bullen et al., 2005). Furthermore, knockout studies have shown that iron uptake mechanisms such as FeoB are required for full virulence in vivo (Tsolis et al., 1996; Janakiraman & Slauch, 2000; Boyer et al., 2002; Naikare et al., 2006). Bacteria sense the availability of iron in the environment, and a change in the amount of available iron (for example, from high to low) during invasion of the host is an important signal for the expression of virulence genes. On the one hand, under low-iron conditions, the production of siderophores is derepressed and also the production of toxins may be upregulated (Litwin & Calderwood, 1993; Payne, 1993). On the other hand, in vitro studies have shown that freely available iron can directly induce the expression of virulence genes in S. Typhimurium; especially, bacterial adhesion to intestinal epithelial cells seems to be stimulated when iron is highly available (Bjarnason et al., 2003; Kortman et al., 2012). Thus, both high and low-iron conditions seem to be able to stimulate different aspects of bacterial virulence.
Battle for iron in the human body
Both the host and the bacterial pathogen require iron for their cellular functions. As discussed above, free iron can be very toxic and that is one reason iron is bound to high-affinity host proteins such as ferritin, transferrin, lactoferrin and haemoproteins, resulting in extremely low free iron concentrations of about 10−24 M (virtually zero) (Raymond et al., 2003). In the human body, tight binding of iron to proteins is also an effective form of innate defence against microorganisms, as they relatively require much higher free concentrations of 10−7–10−5 M for optimal growth, but which is still rather low. These host defence mechanisms are further enhanced upon infection. For instance on the systemic level, the inflammatory response evokes IL-6 production, which induces the production of the iron-regulating peptide hormone hepcidin in the hepatocytes. Hepcidin, in turn, binds to the iron exporter ferroportin at the membrane of host cells, for example macrophages and duodenal cells, hereby inducing its internalisation and degradation (Kroot et al., 2011). The result of these events is a decrease in intestinal iron uptake, an increase in reticulo-endothelial system (RES) iron stores and consequently a decrease in iron availability to extracellular pathogens. Therefore, the ability to overcome iron withdrawal mechanisms of the human body in general is an important virulence trait of bacteria, and there is no fight for iron without the armour of the pathogenic opponent. To acquire iron in the iron-limiting conditions created by the host, in particular during an inflammatory response, (invading) pathogens may express receptors for host transferrin and lactoferrin, but more well known is the secretion of iron-scavenging siderophores (Andrews et al., 2003; Cairo et al., 2006). These molecules mostly have a very high specificity and affinity for ferric iron and can therefore compete with iron bound to host proteins. To illustrate, transferrin has an affinity constant for ferric iron of about 1022, while the association constant of the siderophore enterobactin for ferric iron is c. 1051 (Carrano & Raymond, 1979; Aisen & Listowsky, 1980). We note that (slightly) different association constants are circulating in literature for transferrin and enterobactin (Supporting Information, Supplementary Data). Fortunately, the fight for iron does not end here as the mammalian host has evolved a mechanism to sequester some bacterial ferric–siderophore complexes with the innate defence peptide lipocalin-2 (a.k.a. neutrophil gelatinase-associated lipocalin (NGAL), siderocalin, 24p3, uterocalin). By binding to the ferric–enterobactin complex, lipocalin-2 prevents the uptake of ferric–enterobactin by bacteria, thereby enhancing nutritional immunity (Flo et al., 2004). However, certain pathogenic bacteria can evade this sophisticated host defence mechanism by the production of ‘stealth siderophores’ (Allred et al., 2013). For instance, S. Typhimurium and pathogenic E. coli can produce a C-glucosylated form of enterobactin, termed salmochelin, which escapes from the binding by lipocalin-2 (Hantke et al., 2003; Raffatellu & Baumler, 2010). Another example is the production of aerobactin, which does not bind to lipocalin-2 either, and is produced by for instance Klebsiella, Shigella, some Salmonella serovars and pathogenic E. coli strains (Wooldridge & Williams, 1993; Flo et al., 2004). If and to what extent the here-discussed host and bacterial mechanisms are involved in a battle for iron at the intestinal host–microbiota interface will be discussed in the next sections.
Iron speciation and bacterial iron uptake mechanisms in the colon
Iron speciation and availability for microorganisms in the colon
As far as we know, bacteria synthesise siderophores only when the availability of iron in their surround environment is limited. In the colonic lumen, large amounts of iron are regularly present, mostly constituted by excess dietary iron not absorbed in the duodenum (the major site of iron absorption). This is illustrated by the high concentration of iron found in faeces of British adults on a standard Western diet and in weaning infants fed with complementary solid foods: c. 100 μg Fe g−1 wet weight faeces, which is roughly equal to 1.8 mM, and which is much more than the minimal iron requirement of most bacterial species (Pizarro et al., 1987; Lund et al., 1999) (see also section ). Moreover, the tight mechanisms of iron withholding for systemic sites as described above are not known to play a role in the gut lumen. Nevertheless, iron speciation and the potential presence of lactoferrin, lipocalin-2 and maybe other yet unidentified defence proteins in the colon mucosa probably contribute to the limitation of iron at this site (Fig. 3). Notably, lipocalin-2 is only expressed at a low level under healthy conditions (Cowland & Borregaard, 1997) and would be more effective when readily available iron in the lumen is limited and thus when siderophores are produced.
Due to the nature of foods and of the oxygenic and acidic environment of the stomach, most of the dietary nonhaem iron that enters the intestine is in the ferric form (Jacobs & Miles, 1969). When the pH in the duodenum and small intestine rises, the solubility of ferric iron decreases (Fig. 3). Simultaneously, the increase in pH favours the oxidation of ferrous iron in the presence of oxygen. Further downstream, the reduced environment in the colon, may favour the reduction of iron into the ferrous form (Hedrich et al., 2011). Also, the colonic microbiota may influence the valence state of iron by the action of extracellular reductases (Cowart, 2002; Takeuchi et al., 2005) (Fig. 3). The possible effect of colon luminal pH on iron solubility was discussed in a study from Romanowski et al., in which surgically injured mice with the oral provision of a phosphate buffer at pH 7.5 showed an increased colonic pH compared with mice provided with the same buffer at pH 6.0. The expression of bacterial siderophore systems was upregulated within the pH 7.5 group, possibly due to a decreased solubility of iron in the intestinal lumen at a higher pH (Romanowski et al., 2011). It should, however, be noted that phosphate also influences siderophore production and that iron is prone to form complexes with phosphate, which could have contributed to their findings. These in vivo results are nevertheless consistent with an in vitro study, which showed that lowering of the pH increased iron uptake (Salovaara et al., 2003).
Iron speciation and solubility in the colon is extremely difficult to predict as many factors can influence this process and the various iron species in the lumen are not easy to measure accurately. Consequently, the amount of iron in the colon lumen that is readily available to bacteria is also difficult to estimate. Besides oxygen and pH, which are two the main influencers of iron speciation, different food components can affect the valence state and solubility. The most famous food component in this matter is ascorbic acid (vitamin C), which chelates iron and also reduces ferric iron to its ferrous form (Fig. 3). For this reason, ascorbic acid is also known for its positive effect on iron absorption by the host (Miret et al., 2003). Other organic acids such as citrate are known to form a soluble chelate with iron and may hereby prevent precipitation of ferric iron when the pH rises after passage through the stomach (van Dokkum, 1992) (Fig. 3). Nonetheless, the neutral to mildly acidic pH of the intestinal lumen favours precipitation with hydroxides and complex formation with mucins, certain amino acids, proteins and other food components. Therefore, iron species such as iron oxides, iron hydroxides, iron phosphates and iron carbonates may be found in the intestinal lumen, but we do not know how accessible these insoluble forms of iron are for bacteria (Simpson et al., 1992; Cremonesi et al., 2002) (Fig. 3). Bacteria may solubilise some of these forms by lowering the external pH, by reducing the iron to the more soluble ferrous form or by binding to siderophores (Ratledge & Dover, 2000; Andrews et al., 2003). Ligands that potentially bind to iron and play a role in the colonic lumen are summarised in Supplementary Data. In Supplementary Data, we envisage how orally administered iron will end up in the colon after ingestion. In conclusion, both ferrous and ferric forms of iron are likely to be present in the colon and there are reasons to believe that iron availability to the colonic microbiota is normally limited (see the following paragraphs).
Quest for food-bound iron sources
Because iron speciation in the colon is difficult to predict and it is likely highly variable, not much is known about the accessibility of colonic luminal iron. Nonetheless, we here discuss a few iron sources that are normally not easy to access, but may become available to certain members of the gut microbiota through specialised mechanisms. It is likely that future research will reveal additional food-bound iron sources that can be utilised by bacteria.
Polyphenol–iron
The human diet often contains polyphenols like tannins and catechols, which are highly present in, for example, tea and coffee. These compounds can bind iron very strongly and hereby prevent uptake of iron by the host, but also prevent the uptake of iron by bacteria. However, in an iron-limited environment, pathogenic bacteria that can produce and/or take up siderophores may benefit from the iron polyphenol withdrawal mechanism, most probably by scavenging the iron bound to the polyphenols (Freestone et al., 2007) (Fig. 3). It is not known whether this mechanism plays a significant role in the gut lumen, but it might be relevant as both polyphenols and siderophores are likely to be present in the gut environment. In addition, certain bacteria, such as Streptococcus gallolyticus or Staphylococcus lugdunensis, can degrade the polyphenol tannate and this way provisionally liberate iron from this potent iron binder (Smith et al., 2005; Noguchi et al., 2007).
Phytate–iron
Another compound with potent iron-binding activity is phytate, whose intestinal availability is high after consumption of a cereal-and-legume-based diet. Similar to tannate, certain gut microorganisms (e.g. coliforms and Bifidobacteriaceae) can degrade phytate, which might be a specialised way of liberating iron, and the iron may become available for utilisation by either the degrading organisms or other bacterial species (Markiewicz et al., 2013) (Fig. 3). Therefore, iron bound to phytate might be a relevant iron source for the colonic gut microbiota. It should, however, be noted that the iron complexed with phytate that reaches the colon is mostly in an insoluble form, thus being less susceptible to degradation (Schlemmer et al., 2009). Nevertheless, degradation products of phytate that could be generated only through microbial action were previously found in the colon of conventional rats, but not in germ-free rats (Schlemmer et al., 2009). These findings indicate that phytate degradation by microorganisms occurs in the colon.
The evidence and need for siderophores in the colon
Even though some food compounds may be a source of iron, there are strong indications that readily available iron in the gut is generally limited, as studies have reported that the production of siderophores by enteric pathogens in the gut improves their survival and colonisation. The findings described herein suggest that siderophores are commonly produced by enteric pathogens in the colonic lumen, particularly if the intestine is inflamed. In the absence of intestinal inflammation, ferrous iron is likely available in the gut to some extent, due to the reducing environmental conditions of the colon, but it may also be formed through the action of microbial reductases. As such, enteric bacteria may utilise ferrous iron as an iron source via the Feo-uptake system. A role for Feo in the gut was shown in both S. Typhimurium and E. coli, as isogenic mutant strains deficient in FeoB are outcompeted by their wild-type strains during colonisation in the mouse intestine (Stojiljkovic et al., 1993; Tsolis et al., 1996). Similarly, a Campylobacter jejuni FeoB mutant is outcompeted during colonisation in rabbit ileal loops, in the chick caecum and in the intestinal tract of piglets (Naikare et al., 2006). Notably, a S. Typhimurium strain lacking both FeoB and TonB is further attenuated in the mouse intestine, indicating that both ferrous and ferric iron may be available in the mouse colon (Tsolis et al., 1996). However, a single TonB mutant showed similar colonisation levels compared with the wild-type strain (Tsolis et al., 1996), suggesting that siderophore-mediated iron uptake is only required when the more standard mechanisms of iron uptake are somehow not possible or when freely available iron is absent. In contrast, other studies reported a disadvantage in intestinal colonisation when siderophore-mediated iron uptake was affected. For instance, a S. Typhimurium mutant in the ferrioxamine receptor FoxA was less able to colonise rabbit ileal loops and showed reduced virulence in a mouse typhoid model compared with the wild-type strain, indicating that S. Typhimurium can utilise siderophores in favour of their virulence at the intestinal–epithelial interface (Kingsley et al., 1999).
Other siderophores like aerobactin have been shown to play a role during colonisation of the mouse caecum by a pathogenic E. coli strain (Torres et al., 2012). In addition, E. coli mutants that were affected in the production or uptake of catechol-type siderophores showed impaired colonisation of the mouse intestine compared with the wild-type strain (Pi et al., 2012). Although all of these studies suggest that siderophores are secreted in the intestinal lumen, as far as we are aware, the study of Pi et al. (2012) is the only report that has also directly confirmed the presence of enterobactin in the mouse gut lumen (in the mucus). In future studies, it will be important to provide more information about the types and amounts of siderophores present in the faeces, but it might be challenging to isolate and identify them from such a complex matrix. Taken together, the results discussed herein suggest that the production and utilisation of siderophores is a special-purpose iron uptake system in the gut lumen, and they show that these molecules are required for full bacterial virulence. The importance of siderophore-mediated iron acquisition appears to be even more important in the inflamed gut, where the iron-laden enterobactin is sequestered by the antimicrobial protein lipocalin-2. Nonpathogenic Enterobacteriaceae, such as certain E. coli strains, that solely rely on enterobactin for siderophore-mediated iron acquisition are susceptible to lipocalin-2, while pathogens like S. Typhimurium circumvent lipocalin-2-based defence via the production of alternative stealth siderophores like salmochelin, which is not recognised by lipocalin-2. Thus, salmochelin-dependent iron acquisition provides those species with a competitive advantage in the inflamed gut (Raffatellu et al., 2009) (see also section ). Overall, the aforementioned studies suggest that enteric pathogens constantly have to face the stress of limited iron availability in the normal gut and even more in the inflamed gut, and demonstrate that high-affinity iron uptake systems are required for full virulence.
Iron cross-feeding with heterologous siderophores
An interesting phenomenon is the cross-feeding of heterologous siderophores, that is certain bacterial species can compete for and use each other's siderophores, and sometimes, they are even able to utilise a type of siderophore that cannot be produced by themselves. This is exemplified by Salmonella strains that can take up ferrioxamines (via the receptor FoxA) as mentioned above. They, however, cannot synthesise ferrioxamines themselves, but may be available from related species and other members of normal gut microbiota (Champomier-Verges et al., 1996; Kingsley et al., 1999). Examples of other genera that can utilise heterologous siderophores are Campylobacter and Pseudomonas (Baig et al., 1986; Champomier-Verges et al., 1996; Llamas et al., 2006). A study with microbial isolates from marine sediments indicated that the growth of many bacteria depends on siderophore production by other bacteria within the same community (D'Onofrio et al., 2010). Although there is not yet direct evidence, it may be anticipated that cross-feeding with siderophore–iron plays a significant role in the colonic environment with its microbial community of > 1000 different species (Fig. 3).
Low-affinity siderophores
A distinct type of iron-binding siderophores, that is α-keto-acids and α-hydroxyacids (a reduced form of α-keto-acids), may be important players in the cross-feeding of siderophore–iron. α-Keto-acids and α-hydroxyacids are primary metabolites of both human cells and bacteria, but are also known to bind iron (Drechsel et al., 1993). The ferric form of these siderophores can stimulate the growth of bacteria that reside in an iron-limited environment. To our knowledge, the association constant of these siderophores for iron has not been determined yet, but based on a study by Kingsley et al. (1996), it has been suggested by Reissbrodt et al. (1997) that these iron-binding acids have a lower affinity for iron compared with conventional siderophores. This is in agreement with the notion that conventional siderophores are synthesised for the purpose of iron scavenging, while α-keto-acids are products of general metabolism (Reissbrodt et al., 1997). Notably, it has been shown that the excretion of α-keto-acids by S. Typhimurium was increased upon iron limitation (Reissbrodt et al., 1997). Although low-affinity siderophores may not be very useful for pathogens invading the host with its high-affinity iron-withholding proteins, they could significantly contribute to iron sequestration in the gut lumen where iron most probably can be scavenged more easily. Importantly, certain bacteria, among which are Salmonella spp., can benefit from the α-keto-acids type of siderophores using them as iron sources (Kingsley et al., 1996) (Fig. 3). Consistent with these findings, we found two hydroxyacids with (tentative) siderophoric activity in an in vitro model of the human proximal colon containing a faecal microbiota (Kortman et al., 2014). 2-Hydroxyisovaleric acid was only detected in low-iron conditions and not in moderate to high-iron conditions, and 2-hydroxyisocaproic acid was detected in higher amounts in low-iron conditions compared with the moderate to high-iron conditions (Kortman et al., 2014). These results suggest that the reduced iron availability might be a signal to increase the production of hydroxyacids for at least a subset of the gut microbiota.
Summary of the quest for iron in the colonic lumen
To wrap up the preceding sections on iron speciation and availability in the colon lumen, we here outline the main points. First of all, the total luminal iron content is mainly dependent on dietary iron intake, but is generally considered high. However, the majority of this iron appears not to be readily available for the gut microbiota because much of the iron is bound to food or host-derived compounds (ligands) that, together with the environmental conditions in the gastrointestinal tract, make the iron difficult to access. This is supported by the evidence that siderophore production by enteropathogens takes place in the (inflamed) colon, a process that is only required when readily available iron is low. These and other aspects that point at a gut bacterial quest for iron in the colon lumen are visualised in Fig. 3. Finally, oral iron administration will change the iron speciation balance and it is likely that part of this extra iron becomes readily available for the gut microbiota (see sections and ).
Effect of host iron intake on gut physiology and immunology
To better understand the interplay between nutritional iron, the intestinal microbiota and the host, we review the basic aspect of the effects of luminal iron and host iron status on gut physiology and gut immunology in the following paragraphs.
Colonic iron absorption
In humans, iron is mainly absorbed in the duodenum, but to a small extent also in the colon. Ferrous iron infused into the colon is much better absorbed than ferric iron, which is in line with findings in the duodenum where iron needs to be in the ferrous state for uptake by enterocytes (Ohkawara et al., 1963). It can be envisaged that the effect of iron speciation on iron uptake is larger in the colon compared with the duodenum as the iron within the food matrix has travelled a longer route to the colon with many changes in environmental conditions, allowing the formation of many different iron complexes that possibly affect host iron uptake (see sections and ). Colonic iron absorption may contribute to the iron needs of the body, but it may also be part of a defence mechanism as iron exclusion from the colonic lumen can contribute to nutritional immunity and restraining the gut microbial community, especially the growth and virulence of enteric pathogens (Fig. 3).
Interestingly, the microbiota itself also appears to play a role in host iron absorption. It is known that prebiotics can change the microbiota composition and metabolism as shown in humans, which in turn can influence iron absorption, as shown in pigs (Tako et al., 2008; Petry et al., 2012). A change in bacterial metabolism may, for example, involve an increase in lactate or short chain fatty acids (SCFAs), which can lower the pH and promote solubility and reduction of iron to the ferrous state (Salovaara et al., 2003) (Fig. 3). SCFAs can also stimulate the proliferation of epithelial cells, hereby enhancing the absorptive surface, whereas prebiotics or their fermentation products may increase the expression of iron regulatory genes such as divalent metal transporter 1 (DMT1) in both the duodenum and the colon (Yeung et al., 2005; Tako et al., 2008). It has been shown that iron absorption genes in the colon of mice were highly expressed in iron-deficient mice compared with controls (Takeuchi et al., 2005). This study also showed that the reductase Dcytb (highly expressed in the duodenum) was lowly expressed in mouse colon. Dcytb mediates conversion of ferric iron to ferrous iron, which is crucial for the uptake by enterocytes through the iron importer DMT1. Together, this may suggest that it is not necessary for colonocytes to express this enzyme because the microbiota possesses reductase activity that already contributes to the conversion of ferric iron to ferrous iron (Takeuchi et al., 2005). A role for the gut microbiota in iron absorption is also supported by the finding that germ-free raised rats showed decreased iron uptake compared with conventionally raised rats (Reddy et al., 1972). Notably, also oral iron administration can modify the gut microbiota (see section ) and this implies that the uptake of this administered iron might actually be influenced by the iron-induced changes in microbiota composition and metabolism.
Effect of gut luminal iron on the host epithelial barrier function
Besides the effects of iron on the gut microbiota, which may cause a shift towards a more pathogenic profile and an increase in virulence of enteric pathogens, iron may also directly exert unfavourable effects to the gut epithelium most likely via the promotion of redox stress. Importantly, a decrease in epithelial integrity has been reported in vitro with Caco-2 cells exposed to iron (Ferruzza et al., 2003; Natoli et al., 2009). In contrast, an earlier study did not find a decrease in epithelial integrity when cells were incubated with iron, despite an increase in lipid peroxidation through iron (Courtois et al., 2000). Also, in our hands, we were not able to reproduce the decrease in epithelial integrity of Caco-2 cells observed by Ferruza et al. and Natoli et al. (G.A.M. Kortman, unpublished). However, a combined in vivo–ex vivo study with calf duodenum showed increased permeability after feeding the calves with a high-iron diet, even though duodenal oxidative damage, as measured by malonyldialdehyde, was not different between diets (Hansen et al., 2009). Furthermore, Nchito et al. (2006) showed that African schoolchildren had increased small intestinal permeability after being orally supplemented with iron, as indicated by an increased lactulose: mannitol ratio. This increased permeability may allow easier translocation of pathogenic bacteria across the gut wall. It has also been shown that oral iron tablets, due to iron deposition, can cause pathological changes in the mucosa of the upper gastrointestinal tract (Kaye et al., 2008). Notably, the studies mentioned above were either focused on the small intestine, or their results were based on in vitro data. It is therefore less clear what the effects of iron on the colonic epithelium are in vivo. In the colonic lumen, oxygen is hardly available, but close to the epithelium and in the mucous layers, there is an oxygen tension, resulting from diffusion from epithelial cells (Van den Abbeele et al., 2011). This oxygen may contribute to iron-generated radical formation. Indeed, it has been shown that oral iron supplementation can increase lipid peroxidation in the colon of rats, which may have affected epithelial integrity, and which may contribute to the development of colorectal carcinoma via the induction of DNA damage (Lund et al., 2001). These authors also reported that oral iron supplementation increased the free radical production in the faeces of human healthy volunteers (Lund et al., 1999). Also, epidemiological and animal studies may point to a role of luminal iron in colorectal carcinogenesis (Seril et al., 2002; Chua et al., 2010). As elaborated on in section of this review, nutritional iron may be related to the bacterial production of SCFAs, which lower the pH of the intestinal environment. Remarkably, a lower pH is associated with a reduction in the conversion of bile acids to secondary bile acids, which are thought to exert harmful effects to the colonic wall (Macdonald et al., 1978; Boleij & Tjalsma, 2012). This conversion may also be influenced by iron availability as bacteria use iron-dependent oxidoreductases for this process (Kang et al., 2008). To finish this section, also the bacteria may suffer from iron-mediated oxidation stress in the colon lumen. However, oxygen tension is very low in the outer mucous layer and the lumen where the majority of the microorganisms reside, thus impeding the formation of oxidative stress (Van den Abbeele et al., 2011).
Effect of host iron status on gut immunology and defence
Iron plays an important role in host immunity, and host iron status is therefore likely to influence host immunity ( Fig. 6). First of all, as discussed above, iron withholding is an important innate defence mechanism against microbial invaders. Iron is also required by phagocytic cells to generate the oxidative burst with ROS and reactive nitrogen species to kill the invaders (Cairo et al., 2006).
Both iron deficiency and iron overload are associated with impaired immune responses (Weiss, 2005). Patients with iron overload (haemochromatosis) can experience oxidative damage to cells and organs, but they also have a higher risk of infection because of the increased availability of iron to pathogenic bacteria (Bullen et al., 2005; Cassat & Skaar, 2013). Another reason for the increased risk of infection is the direct effect of iron on the immune system as it has been shown that hfe knockout mice (haemochromatosis mice) showed an attenuated intestinal inflammatory response, probably due to a reduced production of TNF-α and IL-6 by macrophages when they were stimulated with lipopolysaccharide or S. Typhimurium (Wang et al., 2008; Cherayil, 2010). The production of these pro-inflammatory molecules could be enhanced by pre-incubation of the isolated macrophages with hepcidin, which blocks the iron exporter ferroportin, suggesting that the impaired response is mediated by low hepcidin levels in haemochromatosis mice. It was also reviewed by Wang and Cherayil that alterations in intracellular and extracellular iron concentrations can influence the innate immune response by influencing host gene expression (Wang & Cherayil, 2009). Iron-loaded macrophages may fail to kill intracellular pathogens due to a reduced formation of nitric oxide, because iron inhibits interferon-γ (IFN-γ)-mediated pathways (Weiss, 2005). In summary, iron levels need to be in good balance to maintain optimal immunity. Too much iron can provide an easy accessible source of iron for pathogenic bacteria and can reduce the immune response to infection, whereas iron deficiency can strengthen nutritional immunity against pathogenic invaders, but which can also reduce the effectiveness of immune cells (Weiss, 2005).
As host iron status can influence inflammatory pathways, also the intestinal innate mucosal defences are likely to be affected. One interesting aspect is the specific array of antimicrobial defences in the intestine. Enterocytes and Paneth cells secrete antimicrobial peptides (AMPs) such as defensins, cathelicidins and other AMPs that confront the gut microbiota to another stress factor (Muniz et al., 2012). Although little is known about the mechanism of regulation of their expression, AMPs show basal expression levels and they are increasingly expressed in response to inflammatory stimuli. The main site of expression is the small intestine, but to a less extent, AMPs are also expressed in the colon, where they play a role in restraining the gut microbiota (Muniz et al., 2012). As inflammation upregulates AMP expression, iron could provisionally influence expression levels of AMPs. Future research is needed to further elucidate AMP expression pathways and the putative effects of iron on colonic AMP pathways. One molecule with antimicrobial properties that plays a role in the gastrointestinal tract is indoleamine 2,3-dioxygenase 1 (IDO1), a molecule that starves tryptophan from microorganisms (Muller et al., 2009). IDO1 is expressed in high amounts by macrophages and dendritic cells, and its expression can be upregulated by IFNs (Cherayil, 2009). Iron overload could therefore reduce IDO1 expression in the gut, as the expression of IFNs is reduced in iron-loaded macrophages (Weiss, 2005). Another important molecule with antimicrobial activity concerns lipocalin-2, as discussed in section . We found that both mice on an iron-deficient diet and mice on a high-iron supplemented diet showed a decrease in faecal lipocalin-2 levels during intestinal inflammation, which may have resulted in an impaired host defence against siderophilic pathogens in these mice (G.A.M. Kortman, unpublished). The latter observation suggests that extreme (low and high) iron conditions result in an impaired intestinal host defence, but more research is needed to confirm this hypothesis.
Effect of host iron status on gut microbiota composition
As we will discuss in section , dietary iron can alter the gut microbiota composition. In addition, evidence accumulates that also host iron metabolism or iron status, possibly via effects on host immunity or altered luminal iron uptake, can influence the gut microbiota composition. Vice versa, the gut microbiota may influence host iron uptake, and thereby the host iron status. The former was exemplified by Buhnik-Rosenblau et al. who showed that deletions of genes involved in iron homeostasis altered the gut microbiota of the mutant mice. Interestingly, a hfe deletion did not alter faecal iron content or the content of other common minerals, but a Irp2 (iron regulatory protein 2) deletion caused an increase in faecal iron levels and other minerals and electrolytes, due to an altered uptake by intestinal epithelial cells. Although they showed that the gut microbiome of the mutant mice was not significantly different from the wild type, the hfe mutant mice showed a significantly different microbiome compared with the Irp2 mutant mice, possibly due to the greater genetic diversity between the mutant groups than between the single mutants and the wild type (Buhnik-Rosenblau et al., 2012). In a study with pregnant women, increased faecal Enterobacteriaceae and E. coli numbers, in combination with lower Bifidobacteriaceae numbers, correlated with an increase in serum ferritin and in transferrin saturation levels. Although dietary intake was similar among the women of that study, an effect of dietary iron cannot completely be excluded as its intake was not assessed in this study (Santacruz et al., 2010). In another study, it has been shown that Indian women with iron deficiency anaemia had lower levels of Lactobacillaceae in their faeces, despite a similar dietary iron intake compared with nonanaemic individuals (Balamurugan et al., 2010). One limitation of the latter study is that faecal iron levels were not determined and also a limited selection of Lactobacillaceae and other bacterial species were assessed. Besides potential differences in faecal iron due to differential duodenal uptake, another possible explanation for the lower abundance of Lactobacillaceae in the anaemic women is the potential role of colonic iron absorption (see section ). Lactobacillaceae contribute to gut fermentation in a positive way, and a decrease in their abundance could therefore be the reason for reduced colonic iron uptake, or it could even exert a more distant effect on duodenal absorption (Balamurugan et al., 2010). Of note, modulation of the gut microbiota by prebiotics has been associated with increased iron uptake in rats and pigs and in the latter species also with an increase in colonic Lactobacillaceae (Tako et al., 2008; Freitas Kde et al., 2012). This supports a role of the microbiota in iron absorption; however, as far as we are aware, in humans, this effect has not been confirmed (yet) (Petry et al., 2012).
Effect of nutritional iron on the gut microbiota composition
The number of studies investigating the effect of diets on the mammalian gut microbiota has increased greatly during the past years. However, studies investigating the effect of nutritional iron are relatively limited. So far, in vivo (humans and animal models) and in vitro studies have reported various outcomes regarding the influence of iron administration or iron deficiency on the composition of the gut microbiota; however, some findings can be marked as consistent, as summarised in the following paragraphs and in Table 1 and Supplementary Data.
Most studied bacterial groups in relation to dietary iron content in the past few decades
Bacterial group | Direction | Number of studies | Study 1 | Study 2 | Study 3 | Study 4 | Study 5 | Study 6 | Study 7 |
Enterobacteriaceae | Up | 2 | Zimmermann et al. (2010) African schoolchildren | Jaeggi et al. (2014) African infants | |||||
Down | 2 | Dostal et al. (2012b) Rats | Dostal et al. (2012a) in vitro fermentation | ||||||
No change | 6 | Mevissen-Verhage et al. (1985) Dutch infants | Krebs et al. (2013) U.S. infants | Werner et al. (2011) Mice | Ettreiki et al. (2012) Mice and rats | Dostal et al. (2014a) Rats | Kortman et al. (2014) In vitro fermentation | ||
Coliform bacteria/E. coli | Up | 5 | Mevissen-Verhage et al. (1985) Dutch infants | Balmer et al. (1989) UK infants (4 days) | Jaeggi et al. (2014) African infants | Benoni et al. (1993) Rats (2 weeks) | Lee et al. (2008) Piglets | ||
Down | 0 | ||||||||
No change | 5 | Balmer et al. (1989) UK infants (14 days) | Balmer et al. (1991) UK infants | Benoni et al. (1993) Rats (4 weeks) | Tompkins et al. (2001) Mice | Kortman et al. (2014) In vitro fermentation | |||
Lactobacillaceae | Up | 1 | Benoni et al. (1993) Rats (2 weeks) | ||||||
Down | 7 | Zimmerman et al. (2010) African schoolchildren | Krebs et al. (2013) U.S. infants | Tompkins et al. (2001) Mice | Werner et al. (2011) Mice | Dostal et al. (2012b) Rats | Dostal et al. (2012a) in vitro fermentation | Kortman et al. (2014) In vitro fermentation | |
No change | 7 | Mevissen-Verhage et al. (1985) Dutch infants | Balmer et al. (1989) UK infants (4 and 14 days) | Balmer et al. (1991) UK infants | Jaeggi et al. (2014) African infants | Benoni et al. (1993) Rats (4 weeks) | Lee et al. (2008) Piglets | Dostal et al. (2014a) Rats | |
Bifidobacteriaceae | Up | 0 | |||||||
Down | 6 | Mevissen-Verhage et al. (1985) Dutch infants | Krebs et al. (2013) U.S. infants | Jaeggi et al. (2014) African infants | Werner et al. (2011) Mice | Dostal et al. (2012a) in vitro fermentation | Kortman et al. (2014) In vitro fermentation | ||
No change | 4 | Balmer et al. (1989) UK infants (4 and 14 days) | Balmer et al. (1991) UK infants | Zimmermann et al. (2010) African schoolchildren | Lee et al. (2008) Piglets | ||||
Bacteroidetes/Bacteroides | Up | 4 | Mevissen-Verhage et al. (1985) Dutch infants | Krebs et al. (2013) U.S. infants | Werner et al. (2011) Mice | Dostal et al. (2012b) Rats | |||
Down | 3 | Balmer et al. (1991) UK infants | Benoni et al. (1993) Rats (4 weeks) | Dostal et al. (2012a) in vitro fermentation | |||||
No change | 5 | Balmer et al. (1989) UK infants (4 + 14 days) | Jaeggi et al. (2014) African infants | Ettreiki et al. (2012) Mice and rats | Dostal et al. (2014a) Rats | Kortman et al. (2014) In vitro fermentation | |||
Clostridia/Clostridium | Up | 3 | Balmer et al. (1991) UK infants | Jaeggi et al. (2014) African infants | Benoni et al. (1993) Rats (2 weeks) | ||||
Down | 1 | Werner et al. (2011) Mice | |||||||
No change | 5 | Mevissen-Verhage et al. (1985) Dutch infants | Balmer et al. (1989) UK infants (4 and 14 days) | Krebs et al. (2013) US infants | Lee et al. (2008) Piglets | Kortman et al. (2014) In vitro fermentation |
Bacterial group | Direction | Number of studies | Study 1 | Study 2 | Study 3 | Study 4 | Study 5 | Study 6 | Study 7 |
Enterobacteriaceae | Up | 2 | Zimmermann et al. (2010) African schoolchildren | Jaeggi et al. (2014) African infants | |||||
Down | 2 | Dostal et al. (2012b) Rats | Dostal et al. (2012a) in vitro fermentation | ||||||
No change | 6 | Mevissen-Verhage et al. (1985) Dutch infants | Krebs et al. (2013) U.S. infants | Werner et al. (2011) Mice | Ettreiki et al. (2012) Mice and rats | Dostal et al. (2014a) Rats | Kortman et al. (2014) In vitro fermentation | ||
Coliform bacteria/E. coli | Up | 5 | Mevissen-Verhage et al. (1985) Dutch infants | Balmer et al. (1989) UK infants (4 days) | Jaeggi et al. (2014) African infants | Benoni et al. (1993) Rats (2 weeks) | Lee et al. (2008) Piglets | ||
Down | 0 | ||||||||
No change | 5 | Balmer et al. (1989) UK infants (14 days) | Balmer et al. (1991) UK infants | Benoni et al. (1993) Rats (4 weeks) | Tompkins et al. (2001) Mice | Kortman et al. (2014) In vitro fermentation | |||
Lactobacillaceae | Up | 1 | Benoni et al. (1993) Rats (2 weeks) | ||||||
Down | 7 | Zimmerman et al. (2010) African schoolchildren | Krebs et al. (2013) U.S. infants | Tompkins et al. (2001) Mice | Werner et al. (2011) Mice | Dostal et al. (2012b) Rats | Dostal et al. (2012a) in vitro fermentation | Kortman et al. (2014) In vitro fermentation | |
No change | 7 | Mevissen-Verhage et al. (1985) Dutch infants | Balmer et al. (1989) UK infants (4 and 14 days) | Balmer et al. (1991) UK infants | Jaeggi et al. (2014) African infants | Benoni et al. (1993) Rats (4 weeks) | Lee et al. (2008) Piglets | Dostal et al. (2014a) Rats | |
Bifidobacteriaceae | Up | 0 | |||||||
Down | 6 | Mevissen-Verhage et al. (1985) Dutch infants | Krebs et al. (2013) U.S. infants | Jaeggi et al. (2014) African infants | Werner et al. (2011) Mice | Dostal et al. (2012a) in vitro fermentation | Kortman et al. (2014) In vitro fermentation | ||
No change | 4 | Balmer et al. (1989) UK infants (4 and 14 days) | Balmer et al. (1991) UK infants | Zimmermann et al. (2010) African schoolchildren | Lee et al. (2008) Piglets | ||||
Bacteroidetes/Bacteroides | Up | 4 | Mevissen-Verhage et al. (1985) Dutch infants | Krebs et al. (2013) U.S. infants | Werner et al. (2011) Mice | Dostal et al. (2012b) Rats | |||
Down | 3 | Balmer et al. (1991) UK infants | Benoni et al. (1993) Rats (4 weeks) | Dostal et al. (2012a) in vitro fermentation | |||||
No change | 5 | Balmer et al. (1989) UK infants (4 + 14 days) | Jaeggi et al. (2014) African infants | Ettreiki et al. (2012) Mice and rats | Dostal et al. (2014a) Rats | Kortman et al. (2014) In vitro fermentation | |||
Clostridia/Clostridium | Up | 3 | Balmer et al. (1991) UK infants | Jaeggi et al. (2014) African infants | Benoni et al. (1993) Rats (2 weeks) | ||||
Down | 1 | Werner et al. (2011) Mice | |||||||
No change | 5 | Mevissen-Verhage et al. (1985) Dutch infants | Balmer et al. (1989) UK infants (4 and 14 days) | Krebs et al. (2013) US infants | Lee et al. (2008) Piglets | Kortman et al. (2014) In vitro fermentation |
Most studied bacterial groups in relation to dietary iron content in the past few decades
Bacterial group | Direction | Number of studies | Study 1 | Study 2 | Study 3 | Study 4 | Study 5 | Study 6 | Study 7 |
Enterobacteriaceae | Up | 2 | Zimmermann et al. (2010) African schoolchildren | Jaeggi et al. (2014) African infants | |||||
Down | 2 | Dostal et al. (2012b) Rats | Dostal et al. (2012a) in vitro fermentation | ||||||
No change | 6 | Mevissen-Verhage et al. (1985) Dutch infants | Krebs et al. (2013) U.S. infants | Werner et al. (2011) Mice | Ettreiki et al. (2012) Mice and rats | Dostal et al. (2014a) Rats | Kortman et al. (2014) In vitro fermentation | ||
Coliform bacteria/E. coli | Up | 5 | Mevissen-Verhage et al. (1985) Dutch infants | Balmer et al. (1989) UK infants (4 days) | Jaeggi et al. (2014) African infants | Benoni et al. (1993) Rats (2 weeks) | Lee et al. (2008) Piglets | ||
Down | 0 | ||||||||
No change | 5 | Balmer et al. (1989) UK infants (14 days) | Balmer et al. (1991) UK infants | Benoni et al. (1993) Rats (4 weeks) | Tompkins et al. (2001) Mice | Kortman et al. (2014) In vitro fermentation | |||
Lactobacillaceae | Up | 1 | Benoni et al. (1993) Rats (2 weeks) | ||||||
Down | 7 | Zimmerman et al. (2010) African schoolchildren | Krebs et al. (2013) U.S. infants | Tompkins et al. (2001) Mice | Werner et al. (2011) Mice | Dostal et al. (2012b) Rats | Dostal et al. (2012a) in vitro fermentation | Kortman et al. (2014) In vitro fermentation | |
No change | 7 | Mevissen-Verhage et al. (1985) Dutch infants | Balmer et al. (1989) UK infants (4 and 14 days) | Balmer et al. (1991) UK infants | Jaeggi et al. (2014) African infants | Benoni et al. (1993) Rats (4 weeks) | Lee et al. (2008) Piglets | Dostal et al. (2014a) Rats | |
Bifidobacteriaceae | Up | 0 | |||||||
Down | 6 | Mevissen-Verhage et al. (1985) Dutch infants | Krebs et al. (2013) U.S. infants | Jaeggi et al. (2014) African infants | Werner et al. (2011) Mice | Dostal et al. (2012a) in vitro fermentation | Kortman et al. (2014) In vitro fermentation | ||
No change | 4 | Balmer et al. (1989) UK infants (4 and 14 days) | Balmer et al. (1991) UK infants | Zimmermann et al. (2010) African schoolchildren | Lee et al. (2008) Piglets | ||||
Bacteroidetes/Bacteroides | Up | 4 | Mevissen-Verhage et al. (1985) Dutch infants | Krebs et al. (2013) U.S. infants | Werner et al. (2011) Mice | Dostal et al. (2012b) Rats | |||
Down | 3 | Balmer et al. (1991) UK infants | Benoni et al. (1993) Rats (4 weeks) | Dostal et al. (2012a) in vitro fermentation | |||||
No change | 5 | Balmer et al. (1989) UK infants (4 + 14 days) | Jaeggi et al. (2014) African infants | Ettreiki et al. (2012) Mice and rats | Dostal et al. (2014a) Rats | Kortman et al. (2014) In vitro fermentation | |||
Clostridia/Clostridium | Up | 3 | Balmer et al. (1991) UK infants | Jaeggi et al. (2014) African infants | Benoni et al. (1993) Rats (2 weeks) | ||||
Down | 1 | Werner et al. (2011) Mice | |||||||
No change | 5 | Mevissen-Verhage et al. (1985) Dutch infants | Balmer et al. (1989) UK infants (4 and 14 days) | Krebs et al. (2013) US infants | Lee et al. (2008) Piglets | Kortman et al. (2014) In vitro fermentation |
Bacterial group | Direction | Number of studies | Study 1 | Study 2 | Study 3 | Study 4 | Study 5 | Study 6 | Study 7 |
Enterobacteriaceae | Up | 2 | Zimmermann et al. (2010) African schoolchildren | Jaeggi et al. (2014) African infants | |||||
Down | 2 | Dostal et al. (2012b) Rats | Dostal et al. (2012a) in vitro fermentation | ||||||
No change | 6 | Mevissen-Verhage et al. (1985) Dutch infants | Krebs et al. (2013) U.S. infants | Werner et al. (2011) Mice | Ettreiki et al. (2012) Mice and rats | Dostal et al. (2014a) Rats | Kortman et al. (2014) In vitro fermentation | ||
Coliform bacteria/E. coli | Up | 5 | Mevissen-Verhage et al. (1985) Dutch infants | Balmer et al. (1989) UK infants (4 days) | Jaeggi et al. (2014) African infants | Benoni et al. (1993) Rats (2 weeks) | Lee et al. (2008) Piglets | ||
Down | 0 | ||||||||
No change | 5 | Balmer et al. (1989) UK infants (14 days) | Balmer et al. (1991) UK infants | Benoni et al. (1993) Rats (4 weeks) | Tompkins et al. (2001) Mice | Kortman et al. (2014) In vitro fermentation | |||
Lactobacillaceae | Up | 1 | Benoni et al. (1993) Rats (2 weeks) | ||||||
Down | 7 | Zimmerman et al. (2010) African schoolchildren | Krebs et al. (2013) U.S. infants | Tompkins et al. (2001) Mice | Werner et al. (2011) Mice | Dostal et al. (2012b) Rats | Dostal et al. (2012a) in vitro fermentation | Kortman et al. (2014) In vitro fermentation | |
No change | 7 | Mevissen-Verhage et al. (1985) Dutch infants | Balmer et al. (1989) UK infants (4 and 14 days) | Balmer et al. (1991) UK infants | Jaeggi et al. (2014) African infants | Benoni et al. (1993) Rats (4 weeks) | Lee et al. (2008) Piglets | Dostal et al. (2014a) Rats | |
Bifidobacteriaceae | Up | 0 | |||||||
Down | 6 | Mevissen-Verhage et al. (1985) Dutch infants | Krebs et al. (2013) U.S. infants | Jaeggi et al. (2014) African infants | Werner et al. (2011) Mice | Dostal et al. (2012a) in vitro fermentation | Kortman et al. (2014) In vitro fermentation | ||
No change | 4 | Balmer et al. (1989) UK infants (4 and 14 days) | Balmer et al. (1991) UK infants | Zimmermann et al. (2010) African schoolchildren | Lee et al. (2008) Piglets | ||||
Bacteroidetes/Bacteroides | Up | 4 | Mevissen-Verhage et al. (1985) Dutch infants | Krebs et al. (2013) U.S. infants | Werner et al. (2011) Mice | Dostal et al. (2012b) Rats | |||
Down | 3 | Balmer et al. (1991) UK infants | Benoni et al. (1993) Rats (4 weeks) | Dostal et al. (2012a) in vitro fermentation | |||||
No change | 5 | Balmer et al. (1989) UK infants (4 + 14 days) | Jaeggi et al. (2014) African infants | Ettreiki et al. (2012) Mice and rats | Dostal et al. (2014a) Rats | Kortman et al. (2014) In vitro fermentation | |||
Clostridia/Clostridium | Up | 3 | Balmer et al. (1991) UK infants | Jaeggi et al. (2014) African infants | Benoni et al. (1993) Rats (2 weeks) | ||||
Down | 1 | Werner et al. (2011) Mice | |||||||
No change | 5 | Mevissen-Verhage et al. (1985) Dutch infants | Balmer et al. (1989) UK infants (4 and 14 days) | Krebs et al. (2013) US infants | Lee et al. (2008) Piglets | Kortman et al. (2014) In vitro fermentation |
Human studies
It was recently shown that iron fortification resulted in a shift towards a potentially more pathogenic gut microbiota profile (i.e. increased relative abundance of potential pathogenic Enterobacteriaceae and a decrease in beneficial Lactobacillaceae) among schoolchildren in Ivory Coast (Zimmermann et al., 2010). Notably, although not statistically significant, numbers of Salmonella spp. tended to be higher in the iron-fortification group. The increase in the abundance of faecal Enterobacteriaceae correlated with the increase in faecal calprotectin, which is a marker of gut inflammation. Very recently, similar effects were found in a study among Kenyan infants. Iron fortification increased the relative abundance of Enterobacteriaceae, among which were pathogenic E. coli species, and decreased the relative abundance of Bifidobacteriaceae, which also included a higher ratio of Enterobacteriaceae to Lactobacillaceae. Also, in this study, faecal calprotectin was elevated in infants receiving iron fortificant compared with the control group (Jaeggi et al., 2014). Earlier studies showed by using culture techniques that an iron-fortified cow's milk preparation did not result in differential Enterobacteriaceae (other than E. coli) and Lactobacillaceae counts in neonatal (Dutch infants, first 3 months of life) gut microbiota, but isolation frequencies of Bacteroides spp. and E. coli were higher and isolation frequencies of Bifidobacteriaceae were lower under these conditions (Mevissen-Verhage et al., 1985). Other bottle-fed UK infants who received milk complemented with lactoferrin or lactoferrin plus iron also showed increased numbers of E. coli in the iron group, but no effect on Bifidobacteriaceae, Lactobacillaceae, Bacteroides and Clostridia was found (Balmer et al., 1989).
In a later study with iron-fortified formulas (without lactoferrin fortification), the same investigators found an increase in Clostridia and a decrease in Bacteroides, with no effect on E. coli, coliforms, Bifidobacteriaceae and Lactobacillaceae counts in faeces of UK infants (Balmer & Wharton, 1991). This paragraph is finished with the interesting findings from a study with US infants in which an iron-fortified diet was compared to a different meat diet that contained much less iron. Although the different types of diet may have influenced or contributed to differences in the gut microbiota composition, the iron-fortified diet was associated with a decrease in Bifidobacteriaceae and Lactobacillaceae and an increase in Bacteroidetes. Remarkably, when zinc was added to the iron-fortified diet, the microbiome responded similar to the meat diet, which contained similar amounts of zinc compared with the iron- and zinc-fortified diet (Krebs et al., 2013). Notably, zinc has been associated with reduced virulence of enteropathogens in the gut via the induction of bacterial envelope stress (Crane et al., 2011; Mellies et al., 2012).
Animal studies
A study with weanling pigs receiving an iron-supplemented diet again reported higher counts of coliform bacteria and found no effect on the populations of total anaerobic bacteria, Bifidobacteriaceae, Lactobacillaceae and Clostridia (Lee et al., 2008). In two independent mice studies, it was found that healthy mice receiving an iron-deficient diet had increased Lactobacillaceae numbers compared with the control group (Tompkins et al., 2001; Werner et al., 2011). Further, Tompkins et al. found higher numbers of total anaerobes and Enterococcus spp. in mice fed on the iron-deficient diet, while Werner et al. found increased numbers of Bifidobacteriaceae, Clostridium and unclassified Prevotellaceae and decreased numbers of Bacteroides. Enterobacteriaceae counts were apparently not affected (not reported), but the parent taxon Proteobacteria appeared to be more abundant in the iron group (Werner et al., 2011).
In rats, depending on iron dose and time point, several effects were found. There was an increase in Clostridium difficile enterotoxin (only after 24 h), and at 2 weeks, an increase in E. coli, Lactobacillaceae and Clostridium spp. was found, while Lactobacillaceae abundance was not different at 4 weeks and Clostridium spp. were again more abundant at 4 weeks (high-iron dose only). The latter increase was accompanied by a decrease in enterococci and Bacteroides spp. (Benoni et al., 1993). A recent study with young rats showed that an iron-deficient diet increased both Enterobacteriaceae and Lactobacillaceae numbers, while Bacteroides spp. and Roseburia spp./Eubacterium rectale numbers decreased. Subsequent iron repletion did not restore Roseburia spp./E. rectale numbers, but Lactobacillaceae and Enterobacteriaceae populations got back to their baseline levels (Dostal et al., 2012b). In a very recent study of Dostal et al. (2014a), various iron doses caused rather disperse effects on the abundance of the most dominant bacterial taxa in rats with a humanised gut microbiota. In another recent study with rats and mice in which only Bacteroidetes, Enterobacteriaceae and Firmicutes were assessed, no effect of ferric iron on these tested gut microorganisms was found in noncolitis animals. These findings could indicate that ferric iron has less effect on the gut microbiota, but two important limitations of this study were the limited selection of tested taxa and the lack of testing the effects of the ferrous iron control on those taxa (Ettreiki et al., 2012).
In vitro studies
The in vivo studies described above illustrate that the effects of oral iron supplementation or an iron-deficient diet, leading to high and low amounts of colonic luminal iron, respectively, can be various and contradictive. Host factors such as iron status, intestinal immune function, dietary habits and also environmental factors such as hygiene play probably an important role and result in a large variation in the effects of iron on the gut microbiota composition. The effect of such host factors can be better controlled in in vitro gut fermentation models, allowing to study the effect of sole iron on the gut microbiota. Obviously, the lack of host immunity factors is also a drawback of in vitro models. Nevertheless, they are valuable tools for monitoring microbial metabolism during the passage of food. A recent in vitro study by Dostal et al. reported an increase in Lactobacillaceae, Enterobacteriaceae and Roseburia spp./E. rectale under low-iron conditions, which was consistent with the outcome of their study in young rats (Dostal et al., 2012a, b). Notably, when S. Typhimurium was added to this fermentation model, it grew unexpectedly less well under high-iron conditions compared with normal-iron conditions (Dostal et al., 2012a). A possible explanation for this is the absence of host-mediated inflammation on which S. Typhimurium can thrive (see section ). In contrast to the rat study, Bacteroides spp. increased under low-iron conditions, except for extremely low-iron conditions where a decrease was found. Further, in a study where silage making was simulated, it was found that extreme iron depletion by 2,2-dipyridyl had no effect on growth and performance of Lactobacillaceae, but repressed the growth of Enterobacteriaceae effectively (Bruyneel et al., 1990). Recently, we found, using an in vitro model that simulates the luminal conditions of the healthy adult proximal colon, that prominent changes were a relative decrease in Lactobacillaceae and Bifidobacteriaceae and an increase in Roseburia and Prevotellaceae in iron-rich conditions (Kortman et al., 2014).
Summary of the effects of nutritional iron on the gut microbiota composition
From the sections above, it becomes clear that oral iron intake can influence the gut microbiota composition, but we note that the reported effects are markedly different from study to study. We also note that infants and young children belong to the population that most often requires oral iron therapy and that altering the gut microbiota composition may have a relatively large impact on the developing microbiota of infants that do not yet have developed a stable core microbiota (Yatsunenko et al., 2012). However, the potential long-term effects of such iron therapy-induced changes are currently not known. The distinct outcomes of the studies described above can originate from many factors. In in vivo studies, differential results may first of all very well be caused by the host, as the gut microbiota of mice, rats and humans are distinct and are likely to respond differently to iron intervention. The site of sampling of the gut microbiota also varied, as some animals were sampled in their gut (e.g. caecum) after sacrifice, while most studies used samples from excreted stools. Also, the iron source (formulation), dose of iron and length of iron intervention were not the same for all studies (Supplementary Data). Next, there were studies comparing iron-deficient diets with diets containing normal amounts of iron on the one hand and studies comparing normal-iron diets with iron-supplemented/iron-fortified diets on the other hand. Obviously, in vitro studies may also result in different outcomes, compared with in vivo studies, but it has some advantages like the easy testing of various iron sources and concentrations in a highly controlled setting. It should also be mentioned that analysis techniques have changed tremendously during the last decades. Hence, we here compared older studies that used culture techniques, studies that used qPCR (consequently, the various studies did not assess the effect of iron on exactly the same set of taxa) and recent studies that investigated the entire population, that is the most abundant genera, using 16S rRNA gene pyrosequencing. Importantly, the studies differed in age of the human subjects and animals and they resided in dispersed geographic locations. Hence, the intrinsic gut microbiota might be very different at the start of the study and the environmental influence will be diverse. The geographic location might be of particular importance as the prevalence of iron deficiency and infections is generally higher, and hygiene standards are often lower in developing countries compared with industrialised countries. This is probably the reason why adverse effects of oral iron administration are more prevalent in developing countries. Importantly, two recent studies showed that both African children and infants receiving iron had higher levels of potentially pathogenic Enterobacteriaceae and/or higher levels of pathogenic E. coli, which was paralleled lower levels of Lactobacillaceae and/or Bifidobacteriaceae (Zimmermann et al., 2010; Jaeggi et al., 2014). Notwithstanding the difference between studies and their outcomes, this also appears to be the most consistent and robust outcome of the other studies (comparing iron-sufficient or high-iron diets to iron-sufficient or low-iron diets, respectively) as mentioned above and as depicted in Table 1. Escherichia coli was mostly increased and was never reported to decrease; lactobacilli were reported to increase only once and shows basically a net decrease, while bifidobacteria mostly decreased and never increased. In our view, the most relevant outcomes are from the two studies among African children/infants (Zimmermann et al., 2010; Jaeggi et al., 2014), which are overall supported by studies among Western infants as well as animal or in vitro studies. It should also be noted that from a clinical point of view, it is most relevant to study the effects of oral iron administration among children in developing countries, but that those studies are not easy to set up and control. In contrast, studies among subjects in industrialised countries and especially studies with animal and in vitro models can be controlled much better, but are inferior with respect to clinical relevance. It is therefore a future challenge to design the experimental setup of the latter studies in such a way that they better resemble the clinically relevant environmental setting.
It is tempting to find explanations for the hitherto consensus outcome of iron on the gut microbiota composition as described above. The fact that Lactobacillaceae do not require iron (see section ) could be an explanation for their decrease within a microbial community in response to iron, as they might be outcompeted by bacterial species that thrive on the extra iron, for example enteropathogens. We note that in older studies, Enterobacteriaceae as a family were often not assessed, but an increase in coliforms and/or E. coli may consequently favour the growth and colonisation of closely related (pathogenic) Enterobacteriaceae, a phenomenon known as the like-will-to-like concept postulated by Stecher et al. (2010). The common decrease in Bifidobacteriaceae through iron is more difficult to explain as they are known to be dependent on iron as their growth is generally impaired in response to iron limitation, created by the addition of the iron chelator dipyridyl (Bezkorovainy et al., 1996; Cronin et al., 2012). This growth impairment in response to dipyridyl indicates the absence of a high-affinity siderophore-mediated iron uptake system in most Bifidobacterium species, but it also indicates that they require iron for their growth. The common decrease in Bifidobacteriaceae in response to luminal iron could therefore be due to an altered balance in competition with other microorganisms or an effect in response to a changed environment such as the presence of stressful metabolites or oxidative stress.
The effect of nutritional iron on bacterial metabolism in the gut
Next to microbiota composition, nutritional iron will affect metabolic pathways within the gut microbiota. In the following paragraphs, we review the current knowledge on the bacterial fatty acid synthesis and the subsequent effect on virulence of pathogenic bacteria.
Effect of iron on the production of health-promoting SCFAs
Bacterial metabolism is important for our gut health because indigestible food components (mainly complex carbohydrates) are predominantly metabolised to the main SCFAs acetate, propionate and butyrate. These SCFAs are the principal metabolites in the colon, very beneficial for gut health and an energy source for enterocytes and more distant tissues (Macfarlane & Macfarlane, 2011, 2012). The connection between iron and SCFA production was first described in two studies of Dostal et al. (2012a, b) (see also Fig. 4). Specifically, the in vivo levels of butyrate and propionate were lower during luminal iron deficiency in rats and were restored by iron repletion. Although, it cannot be excluded that intestinal SCFA uptake was altered by the dietary interventions, these results suggested that iron supplementation could have a beneficial effect on gut health by increasing SCFA production. In contrast, however, in vitro SCFA production seemed not much stimulated by high-iron conditions, and butyrate and propionate production was most clearly impaired during extremely low-iron conditions. This decreased production was accompanied by a decrease in the SCFA-producing species Roseburia spp./E. rectale and Clostridium Cluster IV members (Dostal et al., 2012a). Our in vitro fermentation studies showed that supplementary iron increased propionate levels slightly, but that total SCFA levels were not significantly changed (Kortman et al., 2014). A third (and very recent) study of Dostal et al. showed reduced caecal levels of acetate, propionate and butyrate in rats on an iron-deficient diet compared with rats on iron-supplemented diets. They additionally showed that propionate and butyrate levels were higher in rats on an iron-supplemented diet compared with rats on a control diet (and which were not first depleted from iron) (Dostal et al., 2014a). It can thus be hypothesised that an iron-deficient diet (in combination with iron deficiency) may be unbeneficial for gut health because luminal SCFA levels can decrease under these conditions. Conversely, iron supplementation may increase health-promoting luminal SCFA levels. Notably, enteric pathogens might benefit from a decrease in luminal SCFA levels, as SCFAs may influence their virulence (described in section ). A decrease in butyrate may also decrease the intestinal expression of the AMP cathelicidin, and hereby weaken the host defence (Raqib et al., 2006; Sekirov & Finlay, 2009).
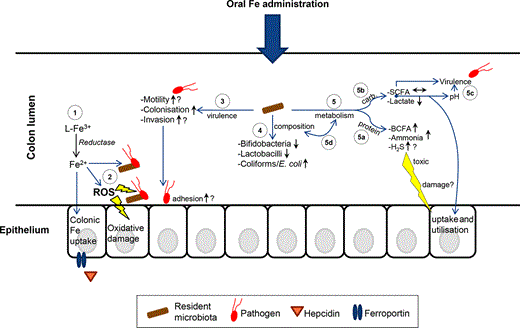
Potential effects of oral iron administration on the microbiota in the noninflamed colon. Oral iron administration: (1) Primarily increases the amount of iron in the colon, which may consist of both ferric and ferrous forms, available for uptake by the microbiota and the host (depicted on the left); (2) Can induce the formation of ROS close to the epithelium, which may elicit damage to the epithelium and stress to the local microbiota; (3) May enhance the virulence of enteric pathogens, favouring colonisation and possibly the ability to adhere to and invade the epithelium; (4) Will alter the gut microbiota composition, which mostly involves a decrease in Bifidobacteriaceae and Lactobacillaceae and an increase in coliforms/E. coli; (5) Likely influences gut microbial metabolism (directly and/or via an altered microbial composition), which likely increases protein fermentation, resulting in the formation of toxic products (5a) and which may also affect beneficial carbohydrate (carb.) metabolism (5b). It is known that iron limitation may lower SCFA production and increase lactate production, which in turn can influence virulence of enteric pathogens either directly or via a change in luminal pH (5c). Notably, a changed microbial metabolism may influence the gut microbiota composition (5d).
A decrease in SCFA production under (extremely) low-iron conditions can, besides changes in microbiota composition, possibly be explained by inhibition of iron-dependent enzymes involved in the pathways of butyrate and acetate synthesis. Approximately one-third of acetate production can be assigned to the reductive acetyl-CoA pathway (a.k.a. Wood-Ljungdahl pathway) (Miller & Wolin, 1996). This pathway contains several enzymes that depend on iron as a cofactor, and acetate production may therefore decrease when iron availability is limited (Ragsdale & Pierce, 2008; Dostal et al., 2012a). Similarly, butyrate production also involves iron-dependent enzymes and may thus be lowered during iron limitation, which could also involve a reduction in acetate to butyrate conversion by certain species (De Vuyst & Leroy, 2011). More (in vivo) research is needed to elucidate the effects of iron on the SCFA production by the gut microbiota, the metabolic pathways involved and the possible effects on gut health. One limitation may, however, be the rapid absorption of SCFAs and other bacterial metabolites by the host (Montagne et al., 2003). Caecal, colonic or faecal SCFA levels do therefore not necessarily reflect the actual production by the gut microbiota, which also differs along the intestinal tract (Mortensen & Clausen, 1996). This makes complementary in vitro studies very useful. Interestingly, African children had much higher faecal SCFA levels compared with European children, thus microbial production appeared to exceed absorption rates in these African children. This difference was likely due to the plant-based diets of the African children, which may result in an enrichment of beneficial SCFA-producing bacteria (De Filippo et al., 2010) (see also section ), but it should also be realised that the poorer health status of the African children may have compromised their capacity to absorb SCFA, which also would result in higher faecal SCFA levels.
SCFAs and bacterial virulence
One of the health effects described to SCFAs relies in the lowering of the luminal pH, which is known to inhibit the growth of (pathogenic) E. coli (Duncan et al., 2009), but also direct effects of SCFAs may cause growth inhibition of E. coli and Salmonella spp. (Topping & Clifton, 2001; Ricke, 2003). Notably, SCFAs and/or lowering of the pH may contribute to an increased solubility of luminal iron and other minerals (Salovaara et al., 2003; Freitas Kde et al., 2012). This in turn may enhance host mineral absorption, but also bacterial mineral uptake, from which both the host and bacteria could benefit. Another beneficial effect of SCFAs from the host's perspective can be an increased host resistance against pathogenic E. coli infection through acetate production of, for example, Bifidobacteriaceae and decreased virulence of pathogens (Fukuda et al., 2011). In addition, enteric pathogens have to deal with the stress of butyrate and propionate as they have been reported to downregulate invasion genes (HilA, HilD, invF, SipC) of Salmonella pathogenicity island 1 (SPI-1) (Van Immerseel et al., 2006). Notably, HilA, invF and SipC expression is also regulated by oxygen, pH and the iron metabolism regulator Fur (ferric uptake regulator protein), resulting in upregulation of HilA under iron sufficiency (Teixido et al., 2011; Troxell et al., 2011). Low oxygen is also a signal for upregulation, but an acidic pH is associated with a downregulation (Bajaj et al., 1996; Teixido et al., 2011). In contrast to the downregulation of invasion genes by SCFAs, also upregulation of a virulence factor, that is the iha gene (involved in adhesion), in pathogenic E. coli has been reported. This SCFA-induced regulation appeared to be independent of iron (Herold et al., 2009). It has also been shown that SCFAs can stimulate S. Typhimurium adhesion and invasion, via the induction of the acid tolerance system in response to the stress of a lower pH; however, the lowering of virulence by SCFA appears to prevail (Ricke, 2003; Van Immerseel et al., 2006) (see also Fig. 4).
Effect of iron on the production of potentially toxic branched chain fatty acids (BCFAs)
In contrast to carbohydrate fermentation, protein fermentation by gut bacteria can result in toxic or potentially toxic metabolites such as ammonia, H2S, BCFAs (e.g. isobutyrate and isovalerate), indolic and phenolic compounds (Macfarlane & Macfarlane, 2012).
Dostal et al. (2012a) showed that the production of BCFAs was decreased under low-iron conditions and increased under high-iron condition in vitro, indicating decreased and increased unbeneficial protein fermentation, respectively. This is in line with our in vitro findings that iron increased the production of BCFAs, and also toxic ammonia production, by an adult faecal microbiota (Kortman et al., 2014). Notably, BCFAs and ammonia are considered to be the indicators of protein fermentation (Hoyles & Wallace, 2010) and these in vitro studies therefore indicate that iron stimulates protein fermentation, which may result in a more putrefactive and potentially toxic or carcinogenic environment (Macfarlane & Macfarlane, 2012; Nyangale et al., 2012) (Fig. 4). In contrast, lactate (mostly derived from carbohydrate) levels have been found to decrease in response to iron in in vitro fermentation studies (Dostal et al., 2012a; Kortman et al., 2014). Notwithstanding the evidence for the toxicity of products originated from protein fermentation shown in multiple in vitro studies, we need to note that evidence for the in vivo toxicity is limited and a recent trial did not support a role of protein fermentation in human gut toxicity (Windey et al., 2012a, b). On the other hand, protein fermentation is often associated with the growth of pathogenic bacteria (Rist et al., 2013). Future in vivo research on the effect of iron on protein fermentation and toxicity is therefore warranted. Finally, it is important to realise that the effects of microbial metabolites are not limited to the gut as they are taken up and can have effects on distant sites and on systemic host metabolism (Mortensen & Clausen, 1996; Zheng et al., 2011; Nicholson et al., 2012). We do not yet know how this affects human health and disease, but it implicates that the iron-induced changes of gut microbial activity may also have systemic effects.
How pathogens can thrive on the altered nutritional conditions during intestinal inflammation
Life in the inflamed intestine
It is currently well known that intestinal inflammation can cause dysbiosis of the gut microbiota and that this often results in an overgrowth of enteric pathogens (Stecher & Hardt, 2008; Winter et al., 2013a, b) (Fig. 5). Pathogens can overcome the intestinal barrier and eventually cause intestinal inflammation when they get the opportunity to breach the colonisation resistance of beneficial resident strains (Kaiser & Hardt, 2011; Lawley & Walker, 2013). This may, for example, occur after antibiotic treatment that disrupts this resident microbiota (Stecher & Hardt, 2008). Paradoxically, also host defence systems can contribute to dysbiosis at the site of infection. The secretion of AMPs and the production of reactive oxygen and nitrogen intermediates will also affect the nonpathogenic resident microbiota. Their susceptibility to radical species may even be enhanced by host-mediated sequestration of zinc and manganese that are required for members of the SOD family (that can neutralise radicals), a process part of the nutritional immunity (Kehl-Fie et al., 2011; Diaz-Ochoa et al., 2014). Once inflammation is established, enteric pathogens can exploit this inflammation and outgrow the resident microbiota (Lupp et al., 2007; Stecher et al., 2007; Barman et al., 2008; Lawley et al., 2008; Winter et al., 2010). First, some general mechanisms adopted by pathogens to achieve this will be discussed in the next paragraphs followed by paragraphs that describe the potential role of iron in such processes.
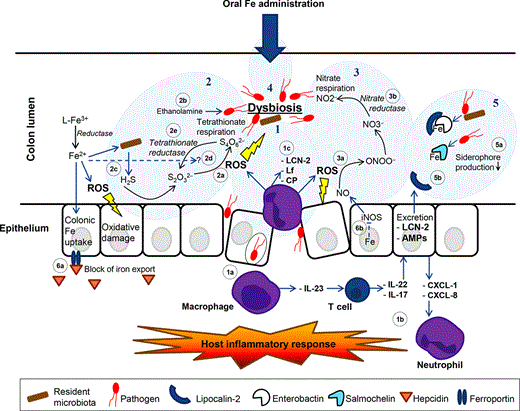
Pathogen exploitation of intestinal inflammation leading to dysbiosis, and the potential effects of oral iron administration. The figure shows processes 1–5 contributing to dysbiosis and process 6 that might be connected: (1) The inflammatory response, triggered by intestinal pathogens, activates macrophages (1a), which in turn stimulates T cells to secrete IL-22 and IL-17. This triggers secretion of chemokines by the epithelium, which attract neutrophils to the site of inflammation (1b). Importantly, the infiltrated neutrophils release ROS into the intestinal lumen (potentially strengthened by iron) as an innate defence mechanism, together with antimicrobial proteins (AMPs) such as lipocalin-2 (LCN-2), lactoferrin (Lf) and calprotectin (CP) (1c). This process and also the secretion of other AMPs by the epithelium cause dysbiosis because pathogens like S. Typhimurium confer an advantage in this inflamed environment; (2) The ROS generation by the neutrophils may be enhanced by luminal iron and which in turn favours the formation of tetrathionate (2a) on which S. Typhimurium can respire, and supports the utilisation of host-derived ethanolamine (2b). The formation of tetrathionate may potentially also be increased by iron-stimulated microbial H2S production (2c). Furthermore, extra iron may directly interfere with alternative respiration by pathogens (2d) and favour the ubiquitous expression of tetrathionate reductase, which contains an iron–sulphur cluster (2e); (3) Similarly, induction of ROS possibly favours formation of the alternative respiration molecule nitrate (3a) and also the nitrate reductase is dependent on an iron–sulphur cluster (3b); (4) Extra iron (coming from the upper GI tract) is likely to also enhance the dysbiosis process directly via its effects on gut microbiota composition; (5) The production of alternative stealth siderophores such as salmochelin normally also promotes dysbiosis, but extra iron is likely to inhibit both siderophore and stealth siderophore production (5a). Lipocalin-2 (LCN-2)-based defence is likely not effective in the absence of siderophores (5b); (6) Inflammation will increase the blood hepcidin levels, which in turn binds to the cellular iron exporter ferroportin at the basolateral side of the epithelial cell. This may cause an increase in intracellular epithelial iron, providing an iron source for invading pathogens (6a). This iron potentially influences inducible nitric oxide synthase (iNOS) production by the epithelium, as has been shown for macrophages (Weiss, 2005) (6b).
Evasion of nutritional immunity
One example of a pathogen that benefits from an inflamed gut is S. Typhimurium. A first step of S. Typhimurium infection is the invasion of nonphagocytic intestinal cells by injecting effector proteins with the type three secretion system encoded by the Salmonella pathogenicity island (SPI)-1 (T3SS-1) (Moest & Meresse, 2013). Secondly, other effector proteins secreted by a second T3SS encoded on SPI-2 contribute to S. Typhimurium replication in macrophages (Figueira & Holden, 2012). Both T3SS are important for the initiation of the inflammatory diarrhoea (Tsolis et al., 1999; Hapfelmeier et al., 2005); however, the amplification of the host response requires the activation of the resident T cells (mainly Th17 cells) to produce cytokines like IL-17 and IL-22, mediated by the macrophage cytokine IL-23 (Godinez et al., 2008, 2009). In turn, IL-17 and IL-22 stimulate intestinal epithelial cells to secrete neutrophil chemoattractants (e.g. CXCL-1, CXCL-8) and antimicrobial proteins including lipocalin-2 (Raffatellu et al., 2009; Behnsen et al., 2014). Moreover, the neutrophils recruited to the gut also secrete large amounts of antimicrobial proteins, including lactoferrin, calprotectin and lipocalin-2 (Blaschitz & Raffatellu, 2010) (Fig. 5). The goal of this host response is to limit micronutrient availability to pathogenic bacteria; however, also nonpathogenic resident strains have to face this armour of host defence. Pathogenic strains that express a lactoferrin receptor or can ‘steal’ iron from lactoferrin by the use of siderophores have a growth advantage over strains unable to do this (Andrews et al., 2003). Moreover, as lipocalin-2 sequesters the iron-laden siderophore enterobactin, bacterial pathogens that evade this response by secreting stealth siderophores such as salmochelin have a competitive advantage for colonising the inflamed gut (Raffatellu et al., 2009; Bachman et al., 2011) (Fig. 5). Furthermore, S. Typhimurium expresses a high-affinity zinc transporter (ZnuABC) with which it can evade the sequestering of zinc by host calprotectin (Liu et al., 2012). In addition to the evasion of nutritional immunity, other mechanisms that allow S. Typhimurium to thrive in the inflamed gut have been discovered and are highlighted below.
Utilisation of alternative respiration molecules by pathogens
In 2010, Winter et al. discovered that S. Typhimurium can thrive on tetrathionate, a molecule formed in the mouse gut during inflammation (Winter et al., 2010). The formation of tetrathionate starts at the site of the gut microbiota, but involves an important host factor: protein fermentation by the gut microbiota produces H2S, which is converted to thiosulphate by the intestinal mucosa. It was postulated that thiosulphate can be converted to tetrathionate via the reaction of thiosulphate with ROS, the latter being formed by the host in the inflammatory process. The newly formed tetrathionate can then be used as an electron acceptor by S. Typhimurium via the action of tetrathionate reductase (Fig. 5). In addition to S. Typhimurium, several other pathogenic members of the phylum Proteobacteria possess this rather unique virulence trait, whereas most members of the gut microbiota are not likely to respire on tetrathionate (Winter & Baumler, 2011). Therefore, tetrathionate respiration in the inflamed intestine appears to provide a luminal growth advantage for these Proteobacteria.
One year later, work in the same laboratory showed that tetrathionate respiration by S. Typhimurium supported this pathogen's utilisation of luminal host-derived ethanolamine (Thiennimitr et al., 2011). Ethanolamine is a substrate that is probably not ready fermentable by competing obligate anaerobic bacteria, thus providing a growth advantage for S. Typhimurium (Winter et al., 2013a) (Fig. 5). Next, it was discovered that E. coli can respire on nitrate, a feature likely to be present in other members of the Enterobacteriaceae as well (Winter et al., 2013b). Like tetrathionate, luminal nitrate levels also increase during intestinal inflammation (Fig. 5). In summary, the release of reactive nitrogen and oxygen species leads to the formation of nitrate, S-oxides and N-oxides on which certain pathogens can respire by the action of nitrate reductase, DMSO reductase and TMAO reductase, respectively; this is in contrast to the many obligate anaerobe members of the gut microbiome that lack these oxidoreductases (Winter et al., 2013b). Taken together, the alternative electron acceptors formed through the oxidative burst of inflammation can stimulate the growth and colonisation of enteric pathogens such as S. Typhimurium, which in turn can exaggerate inflammation. Freely available luminal iron may play a significant role in these processes, which is hypothesised below.
Motility of S. Typhimurium during inflammation
Another mechanism contributing to the exploitation of intestinal inflammation can be found in the motility and chemotaxis. Stecher et al. showed that S. Typhimurium defective in flagellar assembly and the ability to chemotax were only impaired in the inflamed intestine and not in the healthy intestine of mice. It was proposed that fully motile and chemotactic S. Typhimurium has better access to specific energy-rich carbohydrates that are released from mucins during inflammation (Stecher et al., 2008). We envisage that this may provisionally also apply for the access to certain iron sources in the inflamed colon, but this is subject of further investigations. Furthermore, it has been shown that S. Typhimurium uses chemotaxis to seek for niches in which tetrathionate and nitrate are present. The sensing of favourable energy-containing sources and motility are thus important for the capability to thrive in the inflamed gut (Rivera-Chavez et al., 2013).
The potential effect of iron on exploitation of inflammation by enteric pathogens
Iron administration programmes often take place in infection-endemic regions, implicating an increased chance of iron ending up in an already inflamed gut. The mechanisms of pathogens to thrive in an inflamed gut described above inspired us to hypothesise about the possible effects of an increase in luminal iron on these pathogenic mechanisms. To the best of our knowledge, this has not yet been investigated experimentally; therefore, we here can only speculate on the potential effects of iron on exploitation of inflammation by enteric pathogens. These speculations add to the potential effects of iron on the gut microbiota as already discussed above, and which are summarised in Fig. 4.
With regard to nutritional immunity, it can be envisaged that an excess of luminal iron decreases the need for the production of siderophores. Consequently, pathogens that can produce a stealth siderophore do not have an advantage over nonproducers if iron is available, because siderophore production will be repressed (Fig. 5). However, these pathogens are likely to benefit from the extra iron directly and enhance the expression of virulence proteins. Nevertheless, the resident microbiota would at least not be outcompeted via the stealth siderophore-mediated system. It should be noted, however, that intestinal inflammation would still suppress the growth of Bacteroidetes and Firmicutes, while enhancing the colonisation of Proteobacteria. Thus, iron administration is unlikely to increase the colonisation with beneficial microorganisms in this environment. An unfortunate paradox is that both iron withdrawal mechanisms and iron overload may enhance the colonisation of pathogens during inflammation; nevertheless, the best way to limit pathogens’ growth is most likely by iron limitation because this best prevents access to the essential iron. One should also realise that a decrease or absence of siderophores would make lipocalin-2-based defence useless as it only prevents bacterial iron uptake via siderophores. It would therefore make sense that the host only expresses lipocalin-2 when siderophores are present and/or readily available iron is low. It is not yet known whether siderophores or luminal iron concentration can influence lipocalin-2 expression, but we know that this antimicrobial protein is only weakly expressed under normal conditions (Nielsen et al., 1996; Cowland & Borregaard, 1997; Raffatellu et al., 2009).
Another argument for the benefit of iron limitation in the inflamed gut is that iron excess could possibly enhance the alternative respiration pathway via the following mechanisms because: (1) increased luminal iron is associated with increased intestinal permeability and lipid peroxidation and the respiratory burst during inflammation may be enhanced by the redox properties of iron. In this way, iron may increase the production of both tetrathionate ions and nitrate, thus promoting tetrathionate and nitrate respiration by enteric pathogens (Fig. 5); (2) as described (see section ), extra iron can increase microbial protein fermentation, which in turns leads to increased levels of H2S. As H2S is a precursor of tetrathionate, this may further support growth of enteric pathogens during inflammation (Fig. 5); (3) crucial for the alternative respiration is the expression of the oxidoreductases, which require iron–sulphur clusters (Winter & Baumler, 2011); if iron acquisition by the pathogen becomes easier through iron excess, the expression of the iron-containing oxidoreductases might be unhampered. It should be noted that it is not known whether the expression of these oxidoreductases is normally limited due to difficult access to iron (Fig. 5); and (4) finally, the reduction in ferric iron is considered an early form of microbial respiration, by which bacteria can generate energy (Pierre et al., 2002). We therefore envisage that iron itself may function as an electron donor (Fe2+) or acceptor (Fe3+) for certain members of the gut microbiota, but also for pathogens that might use it for alternative respiration during inflammation (Fig. 5). This is in line with the view that reduction of ferric iron is considered an early form of microbial respiration and by which bacteria can generate energy (Pierre et al., 2002). We want to emphasise that these hypothetical mechanisms and their potential contribution to exploitation of inflammation need to be examined experimentally in both in vitro and in vivo models.
Iron and IBD
Compelling evidence that the provision of extra iron to an inflamed gut is contraindicated comes from patients with IBD. These patients often get anaemia of the chronic disease (anaemia of inflammation), because iron uptake and iron homeostasis are unbalanced due to chronically increased systemic hepcidin levels (Cherayil, 2010). These patients therefore require iron supplementation therapy, but caution has to be taken as oral iron has been shown to worsen the symptoms of IBD, while intravenous iron does not (Zhu et al., 2010). In rats and mice where colitis was induced, it was shown that oral iron exaggerated the inflammation (Reifen et al., 2000; Seril et al., 2002; Carrier et al., 2006). Moreover, mutant mice (TNFΔARE/WT) that develop distal ileitis remained healthy on an iron-deficient diet, also in combination with intravenous iron administration (Werner et al., 2011). These studies strongly suggested that oral iron can worsen gut inflammation, which might be (partly) caused by iron-induced radical formation, but also might be a consequence of the increased growth of pathogenic microorganisms that thrive on iron and inflammation, and hereby might contribute to the pathogenesis of IBD. It is known that the gut microbiota of patients with IBD differs from healthy controls, often showing that enteropathogenic strains are increased in patients with IBD (Boleij & Tjalsma, 2012). This difference might be enlarged by iron, as Werner et al. (2011) showed that ferrous sulphate caused marked alterations of the mouse caecal microbiota. Among other differences, mice with ileitis on a diet supplemented with ferrous sulphate showed a reduction in beneficial Bifidobacteriaceae and Lactobacillaceae and an increase in Bacteroidaceae, but a similar abundance of Proteobacteria, when compared to mice with ileitis on a low-iron diet without ferrous sulphate (Werner et al., 2011). Remarkably, Ettreiki et al. showed that juvenile treatment with ferric iron could prevent 2,4,6-trinitrobenzenesulfonic acid (TNBS)-induced colitis in mice and rats, whereas treatment with ferrous iron did not. Ferric iron could also prevent gut microbial dysbiosis, as it prevented the increase in Enterobacteriaceae during colitis (Ettreiki et al., 2012). Results on the effects of ferrous iron on the microbiota were unfortunately not reported.
Also in patients with IBD, administration of iron intravenously is preferred over oral iron administration. A recent systematic review concluded that intravenous iron is more efficient in restoring iron status, with less discontinuation of intervention due to adverse effects, compared with oral iron (Avni et al., 2013). Another recent systematic review highlighted the gastrointestinal adverse effects due to oral iron administration, among which were nausea, diarrhoea and abdominal pain (Lee et al., 2012). It is therefore concluded that intravenous iron therapy is preferred for patients with IBD, which is in line with the conclusion of Zhu et al. (2010), a few years earlier. We, however, note that also intravenous iron therapy may not be without risk as this may induce iron overload (of reticulo-endothelial stores in patients with inflammation) (Rostoker et al., 2012). Finally, a recent study concluded that iron fortification is well tolerated in patients with quiescent IBD (absence of inflammation), but is contra-indicated in patients with relapses (Tolkien et al., 2013). A similar recent study confirmed the detrimental effect of oral iron administration in patients with forms of mildly active IBD (Powell et al., 2013). To our knowledge, no one has investigated the effects of oral iron on the human gut microbiome during IBD yet, but it might very well be that alterations of the gut microbiota during oral iron therapy contribute to the exaggeration of intestinal inflammation. If not directly influenced by iron, certain bacterial species are likely to thrive on the inflammation, which is associated with elevated lipocalin-2 and calprotectin levels, also in IBD (Diaz-Ochoa et al., 2014) (see section ). Furthermore, at the site of intestinal inflammation, luminal iron may exaggerate this inflammation by catalysing the formation of toxic oxygen radicals. This scenario could promote alternative microbial respiration, but could also lead to cellular apoptosis, tissue damage and ER stress (Weiss, 2011). It goes without saying that the adverse effects of oral iron in patients with IBD are likely to apply for patients with acute forms of gastroenteritis as well.
Iron challenges of enteric invasive pathogens during the infection route
This section will specifically discuss the aspects of iron in relation to virulence traits of invasive enteric pathogens that can also cause inflammatory diarrhoea, such as Salmonella, Shigella and Campylobacter (Navaneethan & Giannella, 2008), from the colonic lumen to epithelial invasion, mainly using S. Typhimurium as a model organism. As mentioned in section , iron availability is an important signal for the expression of virulence genes by bacterial pathogens. Although readily available iron is not likely to be present in large amounts in the colon lumen, it is probably still high compared with readily available iron after entering the host tissues, with its excellent iron withdrawal mechanisms. Herein lies the iron challenge: for full virulence, invasive enteric bacteria need mechanisms to deal with the changes in iron concentrations while they simultaneously have to be able to enter the host. To establish host invasion, epithelial adhesion is a first key step and requires different virulence mechanisms compared with the actual invasion, which is the next step to an infection. Moreover, evasion of the host defences is also indispensable to the success of the pathogen.
The very first step that leads to infection may be the expression of flagella by the pathogen. Flagella enable microorganisms to swim in liquids and swarm over surfaces and have also been implicated in the adhesion to epithelial cells (Moens & Vanderleyden, 1996). Motility is thus an important virulence factor and may be essential in the colon lumen to reach the epithelium and ultimately to invade the host. The expression of flagellum protein appears to be regulated by iron in S. Typhimurium and C. jejuni (Tang et al., 2004, 2005) (Butcher & Stintzi, 2013). Results from our laboratory have suggested that iron can increase the expression of flagellum protein in S. Typhimurium in vitro (G.A.M. Kortman, unpublished). More research is needed, but the increased iron availability may thus increase the motility of pathogens in the relative iron-rich environment of the lumen in a noninflamed environment (Fig. 4), which would make sense in an evolutionary view from the pathogen's perspective. Furthermore, it also fits with the finding that flagella synthesis is usually downregulated inside host cells, an environment with extremely low amounts of freely available iron (Ibarra & Steele-Mortimer, 2009).
Once the motile (and invasive) pathogen has reached the epithelium, it should adhere to the cells to be able to invade the epithelium in a second step. We found that adhesion of several enteric pathogens to intestinal epithelial cells was markedly increased by iron in vitro (Kortman et al., 2012). A recent study confirmed this effect, but also showed that iron-induced adhesion was minimised when S. Typhimurium was in an environment with bacterial metabolites, originating from an in vitro fermentation model (Dostal et al., 2014b). Other factors such as iron availability in the different media (cell culture medium vs. fermentation medium) or the deteriorating effect of fermentation medium on cultured intestinal epithelial cells may, however, have contributed to those findings. In vitro data thus show that iron can increase bacterial adhesion (see also Fig. 4), while in vivo studies point at an increased growth and colonisation of potentially pathogenic Enterobactericeae in vivo (Zimmermann et al., 2010; Jaeggi et al., 2014). There is, however, no direct evidence from in vivo studies that adhesion of enteric pathogens to the colon epithelium is increased by iron; thus, further investigations on this issue, and on the effect of iron on molecular bacterial adhesion mechanisms, are necessary.
For invasive pathogens, the next step for the establishment of an infection is the invasion of the intestinal epithelium. In Salmonella and Shigella, the largely studied type 3 secretion system (T3SS-1 and T3SS-2) is important in this step, as well as in the initial adhesion to the epithelium. Misselwitz et al. (2011) showed that T3SS-1 mediates irreversible docking of S. Typhimurium to HeLa cells. Notably, T3SS-1 is considered an essential virulence factor in causing host infections (Que et al., 2013). The expression of T3SS-1, which includes invasion genes, is regulated by the action of multiple genes and regulators. This topic will not be discussed in great detail here, but can be found elsewhere in literature (Teixido et al., 2011; Moest & Meresse, 2013). Importantly, one of the regulators of T3SS is Fur (described in section ) and activation of Fur under iron sufficiency, together with a low oxygen tension, ultimately leads to increased T3SS-1 expression (Ellermeier & Slauch, 2008; Teixido et al., 2011; Troxell et al., 2011). Vice versa, iron limitation will lead to decreased T3SS-1 expression. Notably, iron has shown to upregulate the expression of the invH gene in S. Typhimurium, which encodes an invasion protein (Bjarnason et al., 2003).
When we translate these findings to the gut, it makes sense that the T3SS-1 will be switched on when pathogens transit in the lumen of the noninflamed gut, where iron availability is probably limited, but relatively high compared with the environment inside the host. This scenario supports the pathogen's expression of this important virulence mechanism for invading the host. After invasion, the availability of intracellular iron to pathogens is very limited, which, together with increased oxygen tension, may directly form a signal for the pathogen to switch off the T3SS-1 (Teixido et al., 2011). Instead, the invaded pathogen may increase the expression of different virulence factors, such as the expression of high-affinity iron uptake systems to acquire iron in the iron-limited environment of the host cell and to switch on the T3SS-2 system to promote intracellular survival (Zaharik et al., 2002; Ibarra & Steele-Mortimer, 2009). Interestingly, increased iron status of enterocytes promoted the invasion of Salmonella enteritidis into differentiated Caco-2 cells. Thus, the increased iron status of enterocytes may also further support the invasion process (Foster et al., 2001). The iron content of enterocytes might increase during inflammation because of an increase in systemic hepcidin levels, which in turn prevents iron export from cells, presumably causing the trap of iron inside enterocytes (Fig. 5). It can be envisaged that also oral supplementation can increase enterocyte iron status, although the relevance for the colon has to be investigated further. Notably, in vitro experiments show that moderate (extracellular) iron levels can increase invasion of S. Typhimurium into cultured intestinal epithelial cells, while high-iron levels can conversely decrease invasion (Kortman et al., 2012; Dostal et al., 2014b). Finally, once the pathogen has breached the colonic epithelium, it will highly trigger the host immune response. Survival of the pathogen may partly depend on the host iron status as this can affect the local immune response and the ability to quickly clear the infection (see section ).
To conclude this section, it is clear that iron availability can have a large impact on the infection cycle (Saini et al., 2010) of a pathogen and that increased luminal iron, but also increased intracellular iron levels of enterocytes may exaggerate the virulence of enteric pathogens. More research is, however, needed to investigate these aspects in more detail.
Clinical relevance of nutritional iron stress
Iron deficiency in the tropics and oral iron administration programmes
As outlined above, iron is a highly abundant metal on earth and is vital for virtually all organisms (Cairo et al., 2006). Despite its abundance, iron deficiency is the most prevalent nutrition disorder worldwide, and especially infants and young children in the tropics are in a vulnerable age for developing iron deficiency anaemia. These children require oral iron therapy to prevent serious health consequences such as developmental impairment (WHO & UNICEF, 2004; De-Regil et al., 2011). It has been shown that iron deficiency can be effectively controlled by both iron supplementation and fortification programmes (Zimmermann & Hurrell, 2007). Also, iron-containing micronutrient powders (MNPs), which are added to food after cooking (in-home fortification), can be effective in reducing iron deficiency rates (Adu-Afarwuah et al., 2008). Recent trials have, however, questioned the safety of untargeted iron supplementation and fortification in developing countries (Oppenheimer, 2001; Sazawal et al., 2006; WHO & UNICEF, 2006; Soofi et al., 2013; Zlotkin et al., 2013). In, 2007 the WHO advised against universal iron supplementation and fortification in malaria endemic regions with low standards of health care, considering the dramatic results of the Pemba trial of Sazawal et al. (2006; WHO, 2007). Reported increases in infections during oral iron administration might be at least partly ascribed to bacteria that could originate from the gut and that thrive on extra iron.
Oral iron administration and morbidity
Importantly, as discussed in section , it has recently been shown in two studies that oral iron administration can shift the gut microbiota towards a more pathogenic profile (Zimmermann et al., 2010; Jaeggi et al., 2014). Although morbidity rates were not significantly higher, the increase in enteric pathogens was associated with increased gut inflammation (Zimmermann et al., 2010). Notably, a systematic review of oral iron supplementation trials reported a slight increase in the risk of developing diarrhoea upon oral iron administration (Gera & Sachdev, 2002). Two recent trials with the administration of iron-containing MNPs to young children have also raised concerns with regard to diarrhoea. In Pakistan, a significant increase in severe and bloody diarrhoea and respiratory disease was reported, and in Ghana, there was an increased number of hospitalisations that was possibly partly due to diarrhoea (Soofi et al., 2013; Zlotkin et al., 2013). It should be noted that these MNPs contained multiple micronutrients and vitamins next to iron.
It thus appears that oral iron increases the risk of getting diarrhoea in children. This is an important adverse effect, as diarrhoea contributed for 11% to the mortality of children < 5 years old in the WHO African region in 2010 (WHO, 2013). Although iron-induced diarrhoea so far has not directly been linked to the stimulation of enteric pathogens, it is important to realise that diarrhoea is often a sign of gastrointestinal infection and that luminal iron might play a role in its aetiology (WHO & UNICEF, 2009). Therefore, a prior recommendation of both the WHO and the U.S. National Institutes of Health Working group (NHI TWG) is to investigate the impact of iron preparations on the gut microbiota (WHO & UNICEF, 2006).
Effects of nutritional iron on Salmonella infection and other intestinal pathogens
Salmonella Typhimurium is a common cause of diarrhoea and is a major cause of gastroenteritis and invasive disease in humans worldwide. Remarkably, in tropical Africa, this pathogen is often associated with severe invasive disease rather than with gastroenteritis and diarrhoea (Graham, 2010; Feasey et al., 2012). Furthermore, invasive disease caused by Salmonella often co-occurs with malaria infection as malaria appears to predispose children for systemic Salmonella infection, while both infections clearly have a link with host iron homeostasis (van Santen et al., 2013). As pointed out above, there is a concurrent high prevalence of iron deficiency among children in those countries. These children require oral iron therapy to prevent developmental impairment (WHO & UNICEF, 2004). Precariously, S. Typhimurium appears to be stimulated by increased iron availability in several but not all published studies, as extensively discussed above. Moreover, S. Typhimurium has shown to possess mechanisms to exploit inflammation and outcompete the residing microbiota as discussed in section (Winter et al., 2013a, b). The effect of luminal iron content and host iron status in these exploitation mechanisms are largely unknown and needs therefore more attention. So far, relatively little is known about a potential link of nutritional iron with intestinal infection caused by other enteric pathogens, but which may be important to investigate for its clinical implications. For example, Campylobacter requires iron for successful colonisation of the host intestine and it is known that iron can induce flagella biosynthesis (an important virulence trait) in C. jejuni (Feldmann et al., 2007; Miller et al., 2009). Moreover, certain pathogenic E. coli strains are known for their production and utilisation of stealth siderophores, suggesting an important role of iron in their virulence (Hantke et al., 2003; Feldmann et al., 2007). Importantly, a recent study showed that Kenyan infants receiving iron-containing micronutrient powder had increased faecal pathogenic E. coli numbers compared with infants receiving micronutrient powder without iron (Jaeggi et al., 2014).
Challenges in and approaches for safe oral iron administration without affecting the microbiota composition and metabolism
A big challenge of oral iron administration in infection-endemic regions is to increase the bioavailability of iron to the host, without enhancing the risk of (gut borne) bacterial infections. The particular challenges in a rural and tropical setting, which involve cereal-based monotonous diets and a high prevalence of inflammation (both inhibiting dietary iron absorption) and poor access to proper biomarkers to diagnose iron deficiency, are discussed in Supplementary Data. To overcome these challenges, several strategies to increase bioavailability and safety of iron formulas for universal use have been investigated. Moreover, there are several additional approaches that can be thought of based on theoretical considerations. All these approaches are discussed in Supplementary Data. To summarise, many approaches have already been tested with regard to bioavailability to the host, but to assess their safety, it would be required to study their effects on the gut microbiota. It remains difficult to predict in which form the originally administered iron will end up in the colon and to what extent it can be utilised by the microorganisms, which is especially important with regard to enteric pathogens.
The perfect iron preparation would show good bioavailability for the host, but not for (pathogenic) gut microorganisms, shows no adverse effects and is low cost for application in developing countries. When new iron preparations are tested in regions with high infection pressure, it is advisable to also assess the effects on the gut microbiota. It goes without saying that preparations can first be tested in vitro gut models and animal models that closely resemble the relevant in vivo conditions.
Conclusion and future perspectives
Iron deficiency anaemia is widespread among the world and should generally be corrected to avoid serious health consequences. Although in industrialised countries, oral iron therapy is commonly associated with gastrointestinal side effects, it is not known to increase the burden of infection in this setting. In contrast, in an African region with high infection pressure (e.g. malaria) and low hygiene standards, oral iron supplementation has been associated with increased morbidity and mortality of children. Furthermore, oral administration can increase the incidence of diarrhoea, which may partly be caused by the outgrowth of enteric pathogens. In this review we extensively discussed the multifaceted effects of nutritional iron, oral iron administration, host iron status, intestinal inflammation and nutritional stress on the gut microbiota (summarised in Fig. 6). From a gut microbial perspective, it becomes clear that life in the colon lumen is not always easy and that many stress factors play a role, especially for enteric pathogens that are usually very well restrained in the healthy colon (Fig. 1). Above all, it becomes clear that oral iron administration changes the gut microbiota profile on several fronts. Although the effects appear to be variable among studies, together they point at a shift towards a potentially pathogenic profile. The clinical relevance of these effects is not fully clear yet, but it is highly recommended that potentially pathogenic effects on the gut microbiota need to be avoided as much as possible. We therefore provide leads for the development of alternative iron preparations that provisionally have less impact on the gut microbiome. It goes without saying that more research on this matter will help us to increase the understanding of how the gut microbiome deals with iron and which way the iron can be administered best.
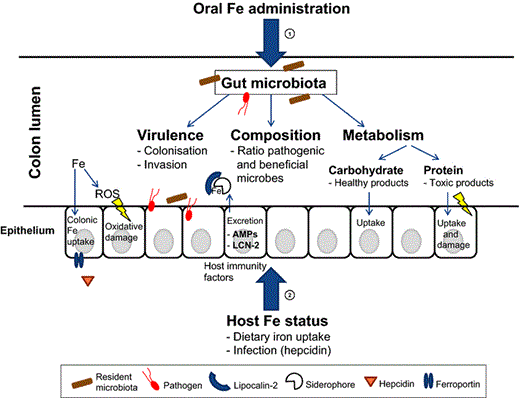
Schematic overview of the main gut microbial factors that are influenced by oral iron administration and host iron status. This figure summarises the main two iron-related components that influence the gut microbiota composition, virulence and metabolism. (1) Orally administered iron can have direct impact on the gut microbiota composition (and the ratio of pathogenic bacteria to beneficial bacteria) and metabolism and potentially on the virulence of enteric pathogens. (2) Host iron status is influenced by dietary iron uptake and infection; this in turn influences host immunity factors which can affect the gut microbiota. Intestinal inflammation (not depicted in this figure) will have a large impact on the gut microbiota (Fig. 5).
Acknowledgements
We thank our colleagues Annemarie Boleij, Rian Roelofs, Bas Dutilh, Anne-Claire Martines, Thijs Richters and Michelle Mulder and our collaborators Tanja Jaeggi and Michael Zimmermann for useful discussions. This work was in part supported by the Dutch Digestive Diseases Foundation (project WO 10-53), by the Public Health Service Grants AI083663 and AI105374 and by the Pacific Southwest Regional Center of Excellence for Biodefense and Emerging Infectious Disease Research funds (supported by award number U54AI065359 from the National Institute of Allergy and Infectious Diseases). The authors have no conflict of interest to declare.
References
Author notes
Editor: Wilbert Bitter