-
PDF
- Split View
-
Views
-
Cite
Cite
Clément Coclet, Cédric Garnier, Gaël Durrieu, Sébastien D'onofrio, Nicolas Layglon, Jean-François Briand, Benjamin Misson, Impacts of copper and lead exposure on prokaryotic communities from contaminated contrasted coastal seawaters: the influence of previous metal exposure, FEMS Microbiology Ecology, Volume 96, Issue 6, June 2020, fiaa048, https://doi.org/10.1093/femsec/fiaa048
- Share Icon Share
ABSTRACT
Our understanding of environmental factors controlling prokaryotic community is largely hampered by the large environmental variability across spatial scales (e.g. trace metal contamination, nutrient enrichment and physicochemical variations) and the broad diversity of bacterial pre-exposure to environmental factors. In this article, we investigated the specific influence of copper (Cu) and lead (Pb) on prokaryotic communities from the uncontaminated site, using mesocosm experiments. In addition, we studied how pre-exposure (i.e. life history) affects communities, with reference to previous metal exposure on the response of three prokaryotic communities to similar Cu exposition. This study showed a stronger influence of Cu contamination than Pb contamination on prokaryotic diversity and structure. We identified 12 and 34 bacterial families and genera, respectively, contributing to the significant differences observed in community structure between control and spiked conditions. Taken altogether, our results point toward a combination of direct negative responses to Cu contamination and indirect responses mediated by interaction with phytoplankton. These identified responses were largely conditioned by the previous exposure of community to contaminants.
INTRODUCTION
Marine coastal environments are especially vulnerable to anthropogenic pressures such as chemical contamination (Halpern et al. 2008; The Mermex Group et al. 2011). For example, copper (Cu) contamination in coastal environments is mainly caused by urban and industrial wastes (Levin et al. 2001; Oursel et al. 2013), or antifouling coatings close to harbors (Turner 2010). Lead (Pb) contamination is mainly associated with historical short-term events (Xu et al. 2014; Dang et al. 2015a,b) or historical fuel consumption for nautical traffic (Callender 2005). Cu contamination is mainly encountered in seawaters of harbor areas, while Pb contamination is found in sediments, probably due to past events (Dang et al. 2015b). In Toulon Bay, Cu and Pb concentrations can be enriched up to >100-fold above geochemical background levels depending on the compartment (e.g. sediment or water column) (Casas et al. 2008; Tessier et al. 2011; Jean et al. 2012; Cossa et al. 2014; Dang et al. 2015b; Twining et al. 2015; Coclet et al. 2018, 2019). Thus, the unique feature of Toulon Bay relates to a gradient of multiple trace metal elements (TMEs) with very low concentrations in open parts comparable to levels measured in open Mediterranean Sea (Morley et al. 1997). By comparison, most enclosed and anthropized areas exhibit very high TME concentrations (Dang et al. 2015a; Coclet et al. 2018, 2019). Therefore, Toulon Bay is a model ecosystem for the study of ecological consequences of TME contamination in the marine coastal environment.
In Toulon Bay where TME gradients are wider than classical physicochemical gradients, TME contamination has been proposed to influence directly the bacterioplankton diversity and structure (Coclet et al. 2019). Several field studies clearly suggested TMEs as structuring factors of abundance, diversity and composition of bacterioplanktonic communities by selecting metal-resistant taxa (Sheeba et al. 2017; Coclet et al. 2018, 2019; Goni-Urriza et al. 2018). A microcosm-based study revealed that artificial mixture of contaminants (nutrients, metals and herbicides) induced a strong inhibition of growth, production, respiration and significant modifications of the functional patterns of bacterioplankton community (Pringault et al. 2016). Furthermore, both industrial effluent discharge and the resuspension of metal-contaminated marine sediments have been shown to affect microbial diversity in a resuspension experiment (Zouch et al. 2018). TME contamination typically found in coastal environments has also been observed to affect phytoplanktonic communities (Mackey et al. 2012; Lafabrie et al. 2013; Coclet et al. 2018), which can have indirect consequences for bacterioplankton community (Goni-Urriza et al. 2018; Coclet et al. 2019).
Life history of a microbial community can be defined as its past exposure to a multitude of environmental changes, like top-down (e.g. grazing pressure, exposition to toxic substances) as well as bottom-up (e.g. phytoplanktonic production) control. The pre-exposure of communities to harmful contaminants like TME can affect the microbial ecology of the marine system and therefore is of major concern (Hughes-Martiny et al. 2006; Bissett et al. 2010; Bell et al. 2013; Sjöstedt et al. 2018; Ward et al. 2019). However, most studies exploring patterns in microbial community diversity and structure in response to environmental change have focused on direct effects, without consideration of life history. Previous TME exposure can lead to an increase of metal tolerance in microbial communities by the elimination of the most sensitive individuals and the replacement of sensitive species by tolerant ones (Gustavson et al. 1999). Serra et al. (2009) found that copper pulse exposure may be toxic to periphyton communities, while continuous copper exposure may lead to community adaptation, which is often related to changes in species composition. All of these studies are in agreement with the pollution-induced community tolerance (PICT) model (Blanck, Wangberg and Molander 1988; Blanck 2002). However, while numerous studies developed the PICT approach based on photosynthetic efficiency, extracellular enzyme activities or respiration activity (Carman, Fleeger and Pomarico 2000; Soldo et al. 2005; Serra, Corcoll and Guasch 2009; Tlili 2013), little is known about how metal exposure impacts diversity and composition of marine prokaryotic communities previously exposed to similar contamination.
Assuming that the life history of its planktonic communities in Toulon Bay would mainly reflect TME exposure history due to the strong and chronic Cu and Pb contamination gradients of the area (personal communication), in this study we aimed to answer two main questions: (i) to what extent do Cu and Pb contaminations affect prokaryotic communities from uncontaminated site at relevant concentrations; and (ii) does prokaryotic TME exposure history play a role in shaping prokaryotic diversity after a Cu exposure?
MATERIALS AND METHODS
Study area, seawater collection, processing and storage
The study was conducted in Toulon Bay, located in the northwestern part of the Mediterranean Sea (Fig. 1 and Supporting Information). Seawater used for the first mesocosm experiment was collected at 1-m depth from site 41p (low contaminated site) (Fig. 1) on 10 May 2016 and seawater used for the second mesocosm experiment was collected at 1-m depth from both sites LAZ (intermediate contaminated site) and 6ext (highly contaminated site) on 2 May 2017 (Fig. 1). The three sampling seawaters used for mesocosm experiments were geochemically contrasted. They were representative of the North-West to South-East decreasing gradient of trace metal contamination (mainly Cu and Pb) previously observed in Toulon Bay (Fig. 1). Minimal concentrations were recorded in the open-sea sampling site (41p), while maximal concentrations were recorded in the northern and enclosed part of the little bay (6ext) where the TME contamination is chronic (Coclet et al. 2019; personal communication). Details on sample's information are given in Table S1 (Supporting Information). Seawater was collected using an air-compressed Teflon pump (AstiPure PFD2, Saint-Gobain, France) connected with Teflon tubing. It was filtered on site through a 5–10 µm cellulose Polycap filter (Whatman) connected to the Teflon tubing after the pump in order to remove the largest grazers. Filtered seawater was collected in 50-L low-density polyethylene (LDPE) tanks (Nalgene®). Back to the lab, each mesocosm was filled with 20 L of filtered seawater. The remaining seawater was filtered through 0.2 µm Teflon Polycap filters (Whatman) to eliminate all organisms, and then stored at 4°C and subsequently used to refill mesocosms during the experiment. All the sampling material, bottles, tanks and filters were pre-cleaned following rigorous protocols, detailed in Supporting Information and in Coclet et al. (2018), in order to keep metal concentrations as close as possible to their initial levels.
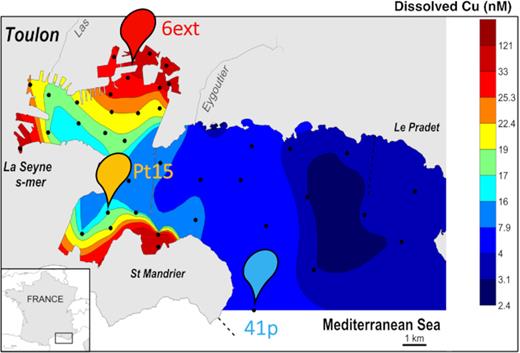
Map of Toulon Bay showing the location of the different sampling sites and the concentration of dissolved copper in surface seawaters measured in February 2014. Solid and dashed lines represent a seawall and treated sewage submarine outlets, respectively.
Mesocosm setup and experimental design
For both mesocosm studies, twelve 20-L LDPE tanks (Nalgene®) were enclosed in a large box covered of plastics in order to avoid contamination in TMEs from ambient particles. Fresh air was continuously provided inside the box by a filtered-equipped fan in order to avoid humidity accumulation and to equilibrate temperature with the surrounding room. The tanks were filled with 5–10 µm filtered water, and thus included a natural marine microbial community mainly composed of free-living organisms. Seawater in each tank was continuously mixed with a Teflon magnetic stir bar used at low speed and oxygenized with 0.2-µm-filtered and humidified ambient air. The 12 tanks were submitted to a daily light/dark cycle of 14/10 h, using full solar spectrum aquariophilly neon tubes and a surface light irradiance of 50 µmol m2s−1 of white light. In the first year, the temperature of the room and of the mesocosms’ box were kept constant at 19 ± 1.6 and 23 ± 1.5°C, respectively. In the second year, the room temperature and the mesocosm interior were kept constant at 19 ± 2.2 and 22 ± 2.0°C, respectively. To maintain resource availability during the experiments, each tank had a continuous opened water circulation system, using a peristaltic pump (Minipuls Evolution®, Gilson, Paris, France), to renew 50% of the total water volume in a week by 0.2-µm-filtered seawater sampled at the same site.
The first mesocosm experiment was performed from 10 May to 17 June 2016 over a 6-week period. Twelve pre-cleaned 20-L LDPE (Nalgene®) tanks were filled with 5–10 µm filtered water from site 41p (Fig. 1). A 2-week acclimation period was carried out to establish relatively stable conditions in each tank. After the acclimation period, seawater from the 12 tanks was pooled, homogenized and then tanks were refilled. Following the acclimation period, seawaters in tanks from the first mesocosm study were assigned to four treatments: (i) site control (41p CTRL, i.e. no TME added to 41p seawater), (ii) Cu addition (150 nM) (41p Cu), (iii) Pb addition (7 nM) (41p Pb) and (iv) Cu and Pb addition (150 + 7 nM, respectively) (41p Cu+Pb) (Figure S1, Supporting Information). The Cu treatments were spiked with a stock solution of Cu nitrate (10−3 M) and the Pb treatments were spiked with a stock solution of Pb nitrate (10−3 M). According to the initial Cu and Pb concentrations measured in the 41p seawater used to fill the tanks, final concentrations of 150 and 7 nM for Cu and Pb, respectively, were targeted. The water supplies were simultaneously spiked with similar concentrations of Cu, Pb or both depending on the treatment to maintain metal concentrations in tanks.
The second mesocosm was performed from 2 May to 12 June 2017 over a 6-week period. Six 20-L LDPE (Nalgene®) tanks were filled with 5–10 µm filtered water from each sampling site (LAZ and 6ext sites) (Fig. 1). The treatments in the second mesocosm experiment were (i) site control (LAZ CTRL, i.e. no TME added to LAZ seawater), (ii) Cu addition to LAZ seawater (150 nM) (LAZ Cu), (iii) site control (6ext CTRL, i.e. no TME added to 6ext seawater) and (iv) Cu addition to 6ext seawater (150 nM) (6ext Cu) (Figure S2, Supporting Information). The Cu treatments were spiked with a stock solution of Cu nitrate (10−3 M). According to the initial Cu concentrations measured in LAZ and 6ext seawaters used to fill the tanks, final concentration of 150 nM for Cu was targeted. The water supplies were simultaneously spiked with Cu depending on the treatment to maintain metal concentrations in tanks.
Experimental time plan and sampling
After the acclimation period, seawater was sampled from the 12 tanks using a peristaltic pump connected with Teflon tubing at different times over the course of the first mesocosm experiment (0, 3, 7, 10, 14, 17, 21 and 25 days) after the Cu/Pb spikes, and the second mesocosm experiment (0, 3, 7, 10, 14, 17, 21, 25 and 28 days) after the Cu spikes. At each sampling time, 333 mL of seawater was sampled in each replicate of a given experimental condition and pooled in 1-L FEP bottles (Nalgene®) to obtain composite samples for each condition. It was then immediately resampled for future trace metals, dissolved organic carbon (DOC) and total nitrogen (TN) quantifications as previously described (Omanović et al. 2006; Cindrić et al. 2015), and fully explained in Supporting Information. Additionally, seawater (10 mL) for flow cytometry analysis was directly collected in 15-mL centrifuge tubes (Falcon) from each of 12 tanks, fixed with 0.25% (final concentration) glutaraldehyde and stored at −80°C until analysis. Finally, at 0, 7, 14, 21 and 25 days, 1 L of seawater from each of 12 tanks was collected in 1-L HDPE bottles and filtered through 0.2-µm polycarbonate membranes (Millipore). Filters were stored at −80°C until DNA extraction.
Seawater physicochemical measurements and analyses
Temperature (°C), pH, dissolved oxygen concentration (mg L−1) and saturation (%) were directly monitored daily into tanks, over the course of both mesocosm experiments, using LDO10105 (Hach) multiparameter probe. Differential pulse anodic stripping voltammetry was used to measure Cd-, Cu-, Pb- and Zn-dissolved concentrations. Details about instruments, analysis conditions and data treatment are provided in the Supporting Information and elsewhere (Oursel et al. 2013; Cindrić et al. 2015). DOC and TN concentrations were determined using a TOC-VCSH analyzer (Shimadzu) (further details in Dang et al. 2014; Coclet et al. 2018, 2019).
Flow cytometry analysis
Autotrophic prokaryotes (Synechococcus-like), photosynthetic picoeukaryotes and photosynthetic nanoeukaryotes populations were characterized and enumerated using a BD Accuri C6 (BD Biosciences) flow cytometer, as previously described (Coclet et al. 2018, 2019). Heterotrophic prokaryotes were enumerated after staining with SYBR Green as previously described (Cabrol, Quéméneur and Misson 2017).
DNA extraction, PCR amplification and sequencing of 16S rRNA gene amplicons
DNA was extracted from the polycarbonate filters by a combination of enzymatic cell lysis (Ghiglione, Conan and Pujo-Pay 2009) and AllPrep DNA/RNA Mini Kit (QIAGEN) according to the manufacturer's instructions. Prokaryotic community structure was assessed by targeting the V4–V5 region of the 16S rRNA gene (Parada, Needham and Fuhrman 2016) and using Illumina Miseq 2× 250 pb paired-end sequencing (Genoscreen, France). The protocol for the DNA extraction and the library preparation is fully described in Coclet et al. (2019).
Bioinformatic analysis
Sequences were demultiplexed and assigned to corresponding samples using CASAVA (Illumina). Bioinformatic analysis was performed on sequences from two sequencing MiSeq runs in the same sequencing company used for our two mesocosm experiments. The analysis of the raw sequences was done by following the standard pipeline of DADA2 (Callahan et al. 2016) in RStudio (R Core Team 2017) with the following parameters: maxN = 0, maxEE = c(2,2), truncQ = 2. Briefly, the package includes the following steps: filtering, dereplication, sample inference, chimera identification and merging of paired-end reads. DADA2 infers exact amplicon sequence variants (ASVs) from sequencing data, instead of building operational taxonomic units (OTUs) from sequence similarity. The taxonomy assignments were done with the SILVA v.128 database (Pruesse et al. 2007; Quast et al. 2013) and the ‘assignTaxonomy’ function in DADA2. For some ASVs, in order to obtain a finer taxonomical resolution, DADA2 package implements a method to make species-level assignments based on exact matching between ASVs and sequenced reference strains in the SILVA v.128 database, using ‘addSpecies’ function. Sequences classified as mitochondria or chloroplast were removed from the ASV table. The datasets from the samples were normalized by random subsampling to include an equal number of reads (n = 2078 reads). Samples with lower number of sequences than the number of reads chosen to the normalization were discarded (Table S1, Supporting Information).
Statistical analysis
All plots and statistical analyses were performed with RStudio (R Core Team 2017). Alpha diversity and error estimates were performed using QIIME script core_diversity_analyses.py (Caporaso et al. 2010), including Chao1, equitability, observed richness, Shannon and Simpson's diversity. Two-way analysis of variance (ANOVA) was applied to investigate the influences of Cu and Pb addition, experimental time and their interaction on α-diversity metrics and the abundance heterotrophic prokaryotes’ community. Non-metric multidimensional scaling (NMDS) based on Bray–Curtis distance was applied to evaluate the overall differences in prokaryotic community structure, using the vegan package in R (Oksanen et al. 2019). Global and pairwise PERMANOVA tests, using vegan and RVAideMemoire packages, respectively, were applied to test significant differences between treatments and sampling time. To find representative phylotypes associated with the treatments, similarity percentage (SIMPER) analyses were first applied to screen OTUs primarily responsible for the overall dissimilarity (i.e. contributing to >1% of total dissimilarity) in the prokaryotic communities between treatments at each time point using vegan package.
RESULTS
Trace metal elements and additional physicochemical parameters
Initial seawater samples (Ti) used for the first (i.e. 41p) and the second (i.e. LAZ and 6ext) experiments were geochemically contrasted and presented variations in trace metal concentrations as expected (Tables S2 and S3, Supporting Information). Total Cu, Pb and Zn concentrations for 41p were 2.44, 0.13 and 5.34 nM, respectively, while the concentrations were 2.5-, 3- and 3.6-fold higher than in LAZ, respectively, representing 15, 0.79 and 40 nM. For initial 6ext seawater, total Cu, Pb and Zn concentrations were 15-, 18- and 26-fold higher than in 41p, respectively, representing 36, 2.32 and 139 nM.
At the beginning of both mesocosm experiments (T0), trace metal concentrations measured in control conditions were close to the ones encountered in initial seawater samples (Ti) from 41p, LAZ and 6ext. Thus, this confirms that no adsorption or contamination in metals occurred in our experiments. In both mesocosms, metal concentrations added were close to the targeted concentrations (150 nM of Cu and 7 nM of Pb) (Tables S2 and S3, Supporting Information). Finally, both Cu and Pb concentrations at the beginning (T0) were not different to those measured at the end (TF) of each experiment, confirming no metal loss in treatments where metals were added (Tables S2 and S3, Supporting Information).
The DOC and TN concentrations were not significantly different between the three initial seawaters (Tables S2 and S3, Supporting Information). For both mesocosm experiments, water temperature, pH, DO and DOC did not exhibit significant differences among conditions, but all of these parameters experienced temporal significant changes during the entire course of experiments (Kruskal–Wallis, P < 0.05). Globally, for both mesocosm experiments, pH and water temperature increased with time, while DO saturation and DOC concentration decreased with time (Tables S2 and S3, Supporting Information).
Ultraphytoplanktonic and heterotrophic prokaryotes’ abundances
During the first mesocosm experiment, total ultraphytoplanktonic abundances were determined by flow cytometry. Abundances were comparable between sampled seawater (Ti) from 41p and both conditions 41p CTRL and 41p Pb (1.1 ± 0.1 × 104 cells mL−1 on average), with low temporal variability during the entire course of experiment (Figure S3A, Supporting Information). Total ultraphytoplanktonic abundances in conditions 41p Cu and 41p Cu+Pb started to decrease 3 days after metal addition, leading to significantly lower abundances than in conditions 41p CTRL and 41p Pb until the end of the experiment (ANOVA, P < 0.001). At the beginning of the experiment (T0), heterotrophic prokaryotes’ abundance measured in all conditions (11 ± 0.3 × 104 cells mL−1 on average) was significantly lower than in sampled seawater (Ti) from the 41p (2.5 × 105 cells mL−1) (ANOVA, P < 0.001) (Figure S3B, Supporting Information). From 3 days after metal addition to the end of the experiment, heterotrophic prokaryotic abundance was significantly higher in conditions 41p Cu and 41p Cu+Pb than in conditions 41p CTRL and 41p Pb (ANOVA, P < 0.001).
During the second mesocosm experiment, total ultraphytoplanktonic abundance in LAZ (1.9 × 104 cells mL−1) was significantly higher than in 6ext seawater (7.9 × 103 cells mL−1) (ANOVA, P < 0.001). After the acclimation period (T0), total ultraphytoplanktonic abundance followed a similar pattern in all conditions, decreasing 3 days after metal addition (Figure S4A, Supporting Information). From 3 days after metal addition to the end of the experiment, total ultraphytoplanktonic abundance was significantly lower in Cu-treated conditions than in LAZ and 6ext control conditions (ANOVA, P < 0.001). Heterotrophic prokaryotic abundance was not significantly different between LAZ (5.8 × 105 cells mL−1) and 6ext (5.0 × 105 cells mL−1) initial seawaters (Figure S4B, Supporting Information). At the beginning of the experiment (T0), this abundance was similar in all conditions (41 ± 1.0 × 104 cells mL−1), while from 7 days after metal addition to the day 17, it was higher in Cu-treated conditions than in control conditions (ANOVA, P < 0.001). From 21 days after metal addition to the end of the experiment, heterotrophic prokaryotic abundance was similar in 6ext CTRL and 6ext Cu. Heterotrophic prokaryotic abundance from LAZ Cu was higher than in LAZ CTRL.
Prokaryotic alpha-diversity
For the first mesocosm experiment, all the α-diversity indices except equitability varied significantly over time (Table 1A; Figure S5, Supporting Information). Globally, prokaryotic α-diversity indices significantly decreased over time in all conditions (ANOVA, P < 0.05). After the metal addition (T0), the Chao1 index was significantly lower in Cu-treated conditions (i.e. 41p Cu and 41p Cu+Pb) relative to both initial seawaters from 41p and condition 41p CTRL (ANOVA, P < 0.001). Simpson's diversity was significantly higher in metal-treated conditions (i.e. 41p Cu, 41p Pb and 41p Cu+Pb) relatively to condition 41p CTRL (ANOVA, P < 0.001). No significant difference was observed in equitability, observed richness and Shannon indices between conditions.
Two-way ANOVA for alpha diversity indices of seawater across different conditions and sampling times for the first (A) and the second (B) mesocosm experiment. P, P-value. Probabilities are marked as follows: ***P< 0.001; **P< 0.01; *P < 0.05.
A . | Chao1 . | Equitability . | Observed OTUs . | Shannon . | Simpson . | |||||
---|---|---|---|---|---|---|---|---|---|---|
. | F . | P . | F . | P . | F . | P . | F . | P . | F . | P . |
Time | 8.6 | < 0.001 | 1.4 | 0.261 | 8.1 | < 0.001 | 5.7 | 0.001 | 6.9 | < 0.001 |
Condition | 3.7 | 0.024 | 1.4 | 0.270 | 2.5 | 0.087 | 0.6 | 0.610 | 3.4 | 0.034 |
Time × Condition | 3.5 | 0.005 | 1.0 | 0.478 | 2.9 | 0.014 | 1.8 | 0.102 | 3.6 | 0.004 |
B | Chao1 | Equitability | Observed OTUs | Shannon | Simpson | |||||
F | P | F | P | F | P | F | P | F | P | |
Time | 8.3 | < 0.001 | 54 | < 0.001 | 14 | < 0.001 | 62 | < 0.001 | 30 | < 0.001 |
Condition | 11 | < 0.001 | 0.7 | 0.606 | 12 | < 0.001 | 3.3 | 0.020 | 1.7 | 0.163 |
Time × Condition | 2.3 | 0.025 | 2.0 | 0.048 | 2.4 | 0.020 | 2.8 | 0.006 | 2.3 | 0.023 |
A . | Chao1 . | Equitability . | Observed OTUs . | Shannon . | Simpson . | |||||
---|---|---|---|---|---|---|---|---|---|---|
. | F . | P . | F . | P . | F . | P . | F . | P . | F . | P . |
Time | 8.6 | < 0.001 | 1.4 | 0.261 | 8.1 | < 0.001 | 5.7 | 0.001 | 6.9 | < 0.001 |
Condition | 3.7 | 0.024 | 1.4 | 0.270 | 2.5 | 0.087 | 0.6 | 0.610 | 3.4 | 0.034 |
Time × Condition | 3.5 | 0.005 | 1.0 | 0.478 | 2.9 | 0.014 | 1.8 | 0.102 | 3.6 | 0.004 |
B | Chao1 | Equitability | Observed OTUs | Shannon | Simpson | |||||
F | P | F | P | F | P | F | P | F | P | |
Time | 8.3 | < 0.001 | 54 | < 0.001 | 14 | < 0.001 | 62 | < 0.001 | 30 | < 0.001 |
Condition | 11 | < 0.001 | 0.7 | 0.606 | 12 | < 0.001 | 3.3 | 0.020 | 1.7 | 0.163 |
Time × Condition | 2.3 | 0.025 | 2.0 | 0.048 | 2.4 | 0.020 | 2.8 | 0.006 | 2.3 | 0.023 |
Two-way ANOVA for alpha diversity indices of seawater across different conditions and sampling times for the first (A) and the second (B) mesocosm experiment. P, P-value. Probabilities are marked as follows: ***P< 0.001; **P< 0.01; *P < 0.05.
A . | Chao1 . | Equitability . | Observed OTUs . | Shannon . | Simpson . | |||||
---|---|---|---|---|---|---|---|---|---|---|
. | F . | P . | F . | P . | F . | P . | F . | P . | F . | P . |
Time | 8.6 | < 0.001 | 1.4 | 0.261 | 8.1 | < 0.001 | 5.7 | 0.001 | 6.9 | < 0.001 |
Condition | 3.7 | 0.024 | 1.4 | 0.270 | 2.5 | 0.087 | 0.6 | 0.610 | 3.4 | 0.034 |
Time × Condition | 3.5 | 0.005 | 1.0 | 0.478 | 2.9 | 0.014 | 1.8 | 0.102 | 3.6 | 0.004 |
B | Chao1 | Equitability | Observed OTUs | Shannon | Simpson | |||||
F | P | F | P | F | P | F | P | F | P | |
Time | 8.3 | < 0.001 | 54 | < 0.001 | 14 | < 0.001 | 62 | < 0.001 | 30 | < 0.001 |
Condition | 11 | < 0.001 | 0.7 | 0.606 | 12 | < 0.001 | 3.3 | 0.020 | 1.7 | 0.163 |
Time × Condition | 2.3 | 0.025 | 2.0 | 0.048 | 2.4 | 0.020 | 2.8 | 0.006 | 2.3 | 0.023 |
A . | Chao1 . | Equitability . | Observed OTUs . | Shannon . | Simpson . | |||||
---|---|---|---|---|---|---|---|---|---|---|
. | F . | P . | F . | P . | F . | P . | F . | P . | F . | P . |
Time | 8.6 | < 0.001 | 1.4 | 0.261 | 8.1 | < 0.001 | 5.7 | 0.001 | 6.9 | < 0.001 |
Condition | 3.7 | 0.024 | 1.4 | 0.270 | 2.5 | 0.087 | 0.6 | 0.610 | 3.4 | 0.034 |
Time × Condition | 3.5 | 0.005 | 1.0 | 0.478 | 2.9 | 0.014 | 1.8 | 0.102 | 3.6 | 0.004 |
B | Chao1 | Equitability | Observed OTUs | Shannon | Simpson | |||||
F | P | F | P | F | P | F | P | F | P | |
Time | 8.3 | < 0.001 | 54 | < 0.001 | 14 | < 0.001 | 62 | < 0.001 | 30 | < 0.001 |
Condition | 11 | < 0.001 | 0.7 | 0.606 | 12 | < 0.001 | 3.3 | 0.020 | 1.7 | 0.163 |
Time × Condition | 2.3 | 0.025 | 2.0 | 0.048 | 2.4 | 0.020 | 2.8 | 0.006 | 2.3 | 0.023 |
For the second mesocosm experiment, all the α-diversity indices except equitability varied significantly over time (Table 1B; Figure S6, Supporting Information). All prokaryotic α-diversity indices significantly decreased over time in all conditions, especially after the 7th day of the experiment (ANOVA, P < 0.05). Average Chao1, observed OTUs and Shannon indices varied across conditions, and they were lower in condition LAZ Cu relative to LAZ CTRL (ANOVA, P < 0.05). No significant difference was observed in all α-diversity indices between conditions 6ext CTRL and 6ext Cu. Finally, all α-diversity indices measured in the second mesocosm experiment were lower compared to those of the first mesocosm experiment.
Prokaryotic community is strongly shaped by Cu in contrast to Pb
In the first mesocosm, the most abundant dominant phyla/classes (>1% of all sequences across all samples) were Alphaproteobacteria, Gammaproteobacteria, Flavobacteriia and Cyanobacteria, representing 96% of the overall relative abundance. Archaea represented <1% of the prokaryotic community of all samples and thus were grouped with all the ‘other’ rare taxa (<1% of all sequences across all samples) in subsequent analysis.
Among the abundant families, 10 representative families, determined by SIMPER analysis, significantly differed treatments over time (Table S5, Supporting Information). Their relative abundances are shown in Fig. 2. Rhodobacteraceae, Family I (Cyanobacteria), Alteromonadaceae and SAR11 Surface 1 clade decreased in Cu-treated conditions compared to 41p CTRL or 41p Pb. These families contributed to 20 ± 1.3, 16 ± 4.6, 6.7 ± 2.5 and 3.0 ± 0.76% of the overall dissimilarity between these two conditions, respectively. Conversely, the relative abundance of Hyphomonadaceae, Flavobacteriaceae and Halieaceae increased in Cu-treated conditions compared to 41p CTRL or 41p Pb and contributed to 34 ± 8.1, 17 ± 4.4 and 4.8 ± 1.6%, respectively, of the overall dissimilarity between these two groups of conditions.
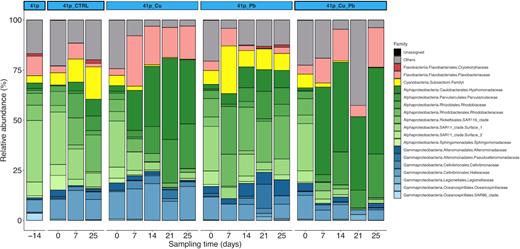
Composition of heterotrophic microbial community at the family level in 41p seawater and in the four conditions over the entire course of the first mesocosm experiment. Each bar represents the relative abundance of family in the samples. Families that on average comprised <1% of the libraries are grouped as ‘Others.’
Moreover, 19 genera showed overall significant differences between conditions at the end of the experiment (TF) (Table S6, Supporting Information). Among these genera, only four were responsible for the structural change in Pb-treated condition. The relative abundance of Alteromonas, Glaciecola and unknown SAR11 Surface 2 clade significantly increased, while Erythrobacter nearly disappeared when only Pb was added. Synechococcus, Litorimicrobium, and to a lesser extent, CHAB XI 27 decreased in 41p Cu compared to 41p CTRL. Conversely, Parvularcula, NS5 marine group and Hyphomonas increased in 41p Cu compared to 41p CTRL (Fig. 3).
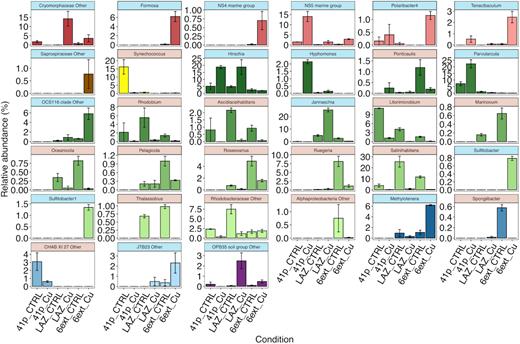
Relative abundance of genera found at week 5 (TF) in both control and Cu-treated conditions from all mesocosm experiments showing significant differences in abundance between conditions (SIMPER). Error bars represent the standard deviation (n = 3). Specific contribution (%) of particular genera to total dissimilarity between prokaryotic communities of two conditions using SIMPER is detailed in Table S8 (Supporting Information). Panel colors represent OTUs, which increased (blue) or decreased (red) after the Cu addition.
Samples from the first mesocosm study were mainly clustered by conditions (PERMANOVA, R2 = 0.41; P < 0.001) and to a lesser extent by sampling times (PERMANOVA, R2 = 0.34; P < 0.001) or interaction (PERMANOVA, R2 = 0.17; P < 0.001) (Fig. 4; Table S4A, Supporting Information). At the beginning of the experiment (T0), prokaryotic community structures were similar between all conditions, and significantly different from the initial seawater at 41p (Table S4B, Supporting Information). After the 7th day, Cu-treated conditions (41p Cu and 41p Cu+Pb) showed significantly different prokaryotic community structures from the other conditions (41p, 41p CTRL and 41p Pb) at each sampling time, especially at the end of the experiment (TF). Finally, there was no significant difference in communities between 41p CTRL and 41p Pb, as well as between 41p Cu and 41p Cu+Pb at each sampling time (Table S4, Supporting Information).
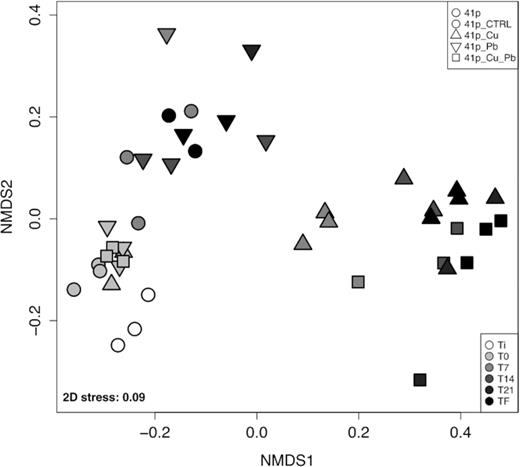
Non-metric dimensional scaling (nMDS) ordination based on Bray–Curtis dissimilarity for 16S rRNA gene libraries between the different conditions at different sampling times during the first mesocosm experiment. Each symbol corresponds to a distinct condition, time and replicate. Empty symbols represent initial seawater samples.
Influence of TME exposure history on copper effects
Prokaryotic community composition and structure
Concerning prokaryotic communities sampled from three geochemically contrasted sites, a low contaminated site (41p), an intermediately contaminated site (LAZ) and a highly contaminated site (6ext)), the most abundant phyla/classes (>1% of all sequences across all samples) were Alphaproteobacteria, Gammaproteobacteria, Flavobacteriia and Cyanobacteria, representing 94% of the overall relative abundance. At the family taxonomic rank, Rhodobacteraceae (25 ± 1.5%), Flavobacteriaceae (16 ± 1.1%), Hyphomonadaceae (11 ± 1.3%), SAR11 Surface 1 clade (6.3 ± 0.89%) and Halieaceae (6.0 ± 0.59%) included the majority of the total sequences (Fig. 5). As for the first mesocosm experiment, Archaea represented <1% of the prokaryotic community of all samples, and they were considered in the group ‘other’ corresponding to the rare taxa in further analyses.
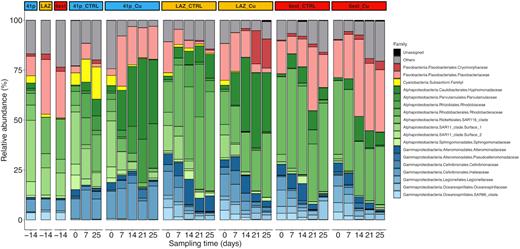
Composition of heterotrophic microbial community at the family level in initial seawaters and in the six conditions over the entire course of both control and Cu-treated conditions from all mesocosm experiments. Each bar represents the relative abundance of family in the studied samples. Families that on average comprised <1% of the libraries are grouped as ‘Others.’
NMDS plot and PERMANOVA tests showed significant clusters corresponding to both sampling times and conditions (Fig. 6; Table S7A, Supporting Information). At all taxonomic rank, Cu addition (PERMANOVA, R2 = 0.58; P < 0.001) showed a greater influence on community variations than time (PERMANOVA, R2 = 0.14; P < 0.001) or interaction between time and experimental condition (PERMANOVA, R2 = 0.20; P < 0.001) (Table S7A, Supporting Information). Prokaryotic community structures were significantly similar between initial seawaters from LAZ and 6ext and significantly different to the initial seawater from 41p (Table S7B, Supporting Information). At the beginning of the experiment (T0), similarly to what was observed for 41p in the first experiment, the prokaryotic community structures in each control conditions were not significantly dissimilar from related Cu-treated ones (LAZ CTRL vs LAZ Cu, and 6ext CTRL vs 6ext Cu). After the 7th day, and until the end of the experiment, significant differences were clearly observed between Cu-treated and non-Cu-treated communities for 41p and LAZ. Conversely, pairwise comparisons showed no significant difference between 6ext CTRL and 6ext Cu, whatever the sampling time.
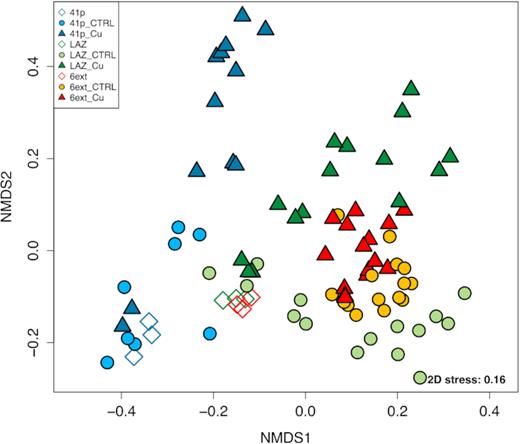
nMDS ordination based on Bray–Curtis dissimilarity for 16S rRNA gene libraries between the different conditions of both control and Cu-treated conditions from all mesocosm experiments. Each symbol corresponds to a distinct condition, time and replicate. Empty symbols represent initial seawater samples.
Representative taxa responsible for structural changes between control and Cu-treated conditions
SIMPER analyses revealed that 12 representative families significantly differed not only initial seawaters, but also control and Cu-treated conditions throughout experiment duration (Fig. 5; Table S8, Supporting Information). SAR11 Surface 1 and Surface 2 clades, Hyphomonadaceae and Family I (Cyanobacteria) were higher in 41p than in LAZ and 6ext, while Flavobacteriaceae and Rhodobacteraceae were more abundant in LAZ and 6ext than in 41p (Table S8, Supporting Information). Finally, major differences between LAZ CTRL and LAZ Cu were explained by the variation of relative abundances of Hyphomonadaceae, Rhodobiaceae, Cryomorphaceae and Alteromonadaceae.
At a lower taxonomic rank, among the 176 genera recorded, 34 significantly differed control and Cu-treated conditions at the end of the experiment (TF) (Fig. 3; Table S9, Supporting Information). In addition, the genera Salinihabitans and Rhodobium discriminating 41p CTRL from 41p Cu decreased in LAZ Cu compared to LAZ CTRL, while Jannaschia, unknown Cryomorphaceae and Hirschia increased in LAZ Cu compared to LAZ CTRL. Ruegeria decreased in 6ext Cu compared to 6ext CTRL, while Formosa, OCS116 clade and Methylotenera increased in 6ext Cu compared to 6ext CTRL. Finally, representative genera responsible for structural changes between control conditions and Cu-treated conditions for 41p and LAZ were mainly abundant genera, while for 6ext, representative genera were mainly rare genera (<1% of all sequences across all samples).
DISCUSSION
Copper exposure induced changes in prokaryotic community
In both mesocosm experiments, temporal variations in prokaryotic community structure were observed regardless of metal additions. However, metal addition led to stronger changes of community structure than the experimental setup or the experiment time. Copper exposure killed >99% of the ultraphytoplanktonic community. The selective pressure of metals on phytoplankton and more precisely on ultraphytoplankton is now well documented (Echeveste, Agustí and Tovar-Sánchez 2012; Lafabrie et al. 2013; Coclet et al. 2018). In parallel to the phytoplankton mortality, we observed an increase in heterotrophic prokaryotes’ abundance and a decrease in their α-diversity if they originated from the lower and intermediately contaminated sites. This abundance increase thus seemed to relate to the development of a limited number of opportunistic r-strategists that efficiently use the organic resources released by the phytoplanktonic decay (Odum 1969; Pianka 1970). This behavior has already been observed with the highly reactive marine microbial community (Buchan et al. 2014; El-Swais et al. 2015). Taken altogether, these observations highlight the strong interdependence between prokaryotic and phytoplanktonic communities, which could partly explain Cu responses of microbial communities through indirect trophic relationships.
When considering the widely reported bacterial resistance to metals (Nies 1999; Baker-Austin et al. 2006), the response of the prokaryotic community to Cu addition might also be due to the persistence or proliferation of resistant taxa, which can be higher than the extinction rate of sensitive taxa (Wang et al. 2015; Qian et al. 2017).
Significant differences in community structure between the control and the Cu-added conditions were found at all sampling time. Particularly, Hirschia (Hyphomonadaceae), NS4 and NS5 marine groups, Formosa and Tenacibaculum (Flavobacteriaceae) increased in Cu-treated conditions for both mesocosm experiments, indicating that they could be more resistant or take advantage from copper addition and phytoplankton decay (Pinhassi et al. 2004). Hyphomonadaceae were known to be abundant in plastic-attached biofilm communities (Zettler, Mincer and Amaral-zettler 2013; Bryant et al. 2016; De Tender et al. 2017; Oberbeckmann et al. 2018), especially in disturbed environments (Briand et al. 2017; personal communication). However, the results in this paper demonstrate for the first time the significant presence of this group in the prokaryotic community of metal contaminated seawater. Flavobacteriacea was also a major component of the microbial community in strongly disturbed and metal-contaminated environments (Allen et al. 2013; Wang et al. 2015; Zouch et al. 2018; Coclet et al. 2019). Finally, the previous observation that Tenacibaculum and Formosa genera are positively selected in Cu-amended 6ext water as abundant and representative taxa from the most contaminated site (6ext) during a one-month-long survey is in agreement with the idea of a similar selective pressure in situ as that observed in our previous field study experiment (Coclet et al. 2019). Conversely, members of Rhodobacteraceae, Family I (Cyanobacteria, mainly Synechococcus), Alteromonadaceae and SAR11 Surface 1 clade families have been proposed to be sensitive in situ to high trace metal levels (Cassier-Chauvat and Chauvat 2014; Coclet et al. 2018, 2019; Corcoll et al. 2019) or characteristic of marine zones away from anthropogenic influence (Gilbert et al. 2009, 2012; Fuhrman, Cram and Needham 2015).
Thus, our study reveals the sensitivity of a part of the prokaryotic community to Cu contamination representative from a harbor, by a combination of direct (i.e. toxicity) or indirect (i.e. related to phytoplankton decay) processes, as suggested recently in a field study (Coclet et al. 2019).
Limited effects of Pb contamination on prokaryotic community
In our study, the effect of Pb exposure on prokaryotic communities was far less pronounced than the effect of Cu exposure. Pb addition using nitrate salts appeared to have limited impact on prokaryotic diversity and structure, with no detectable effect on ultraphytoplankton. Moreover, it did not prove to have a synergistic interaction with Cu. The weak toxicity of Pb on prokaryotic communities could be explained by its resistance and detoxification mechanisms, which are very efficient in microbial organisms (Trevors 1989; Nies 1999; Nayar, Goh and Chou 2004; Naik and Dubey 2013). Additionally, the dichotomy between Cu and Pb effects could be due to the difference in their concentrations added in our mesocosms as well as their bioavailability. Indeed, to mimic the contamination levels observed in Toulon Bay, Pb concentrations were 20-fold lower than Cu concentrations, thus reducing the dose at which the cells were exposed. No data are available in the literature concerning tolerance threshold of marine bacterial communities against Pb contamination. Pb-dissolved concentration was constant throughout the experiment, indicating no Pb adsorption on mesocosm materials or on microbial cells. Thus, the surface-reactive behavior of Pb might not have induced a reduced bioavailability by reducing its dissolved concentration. Therefore, our study does not allow us to explain precisely this difference of toxicity between Cu and Pb, but the non-essential nature of Pb and potential different ways to enter the cells than Cu represent hypotheses that should be studied in future research (Han et al. 2014).
The relative abundance of Glaciecola taxon significantly increased, while Erythrobacter became undetectable when only Pb was added. Glaciecola has been described to be an opportunistic member of prokaryotic communities, its abundance increasing rapidly following an increase in DOC concentration (Sipler et al. 2017; von Scheibner, Sommer and Jürgens 2017). Since DOC concentrations in our experiment were similar in all conditions, the ability of Glaciecola to take advantage compared to other taxa in a Pb-contaminated seawater remains unexplained. The genus Erythrobacter was found to be important taxa in surface-attached communities (Zettler, Mincer and Amaral-zettler 2013; Dussud et al. 2018; Oberbeckmann et al. 2018), and notably in contaminated ecosystems (Briand et al. 2017; Pollet et al. 2018; Catão et al.2019; personal communication). Thus, the strong decrease of Erythrobacter in the water after Pb a ddition could be explained by the ability of this genus to form biofilms in the mesocosms. Indeed, biofilms are one of the most widely distributed and successful modes of life in water environment (Stoodley et al. 2002; Flemming et al. 2016) and a strategy that microorganisms might use to protect them from toxic metals (Harrison, Ceri and Turner 2007).
TME exposure history determines Cu effects on prokaryotic communities
Trace metal contamination gradients in Toulon Bay have been already described by punctual mappings (Jean et al. 2012; Dang et al. 2015b; Coclet et al. 2018) or during short- (Coclet et al. 2019) and long-term surveys (personal communication). These studies demonstrated that TME contamination gradients persist at a pluri-annual scale in Toulon Bay. This led us to assume that prokaryotic communities sampled along this contamination gradient could present different metal exposure histories. Cu addition in both low (41p) and in intermediately (LAZ) contaminated seawaters induced important changes in prokaryotic community structure, while Cu addition in seawater originating from a chronically contaminated site (6ext) only weakly impacted the prokaryotic community structure. Based on the PICT theory (Gustavson et al. 1999; Blanck 2002), this observation validates our assumption and demonstrates the adaptive potential of prokaryotic communities to withstand elevated Cu concentrations. This adaptation must have developed rapidly since the water residence time in Toulon Bay has been estimated to be a few days (Dufresne, Duffa and Rey 2014), even if this estimation is probably not representative of what happens in very enclosed docks such as the one where we sampled contaminated water (6ext). This adaptive potential could be the result of the presence of a higher proportion of tolerant species in the most contaminated seawater, representative of a strong and chronic metal-exposure history of these communities (Carman, Fleeger and Pomarico 2000). This hypothesis is in agreement with the very small variation of structure after Cu addition in the community sampled from the most contaminated site. Indeed, for this community, we mainly observed changes in the relative abundance of rare taxa.
We hypothesize that mechanisms of toxicity and resistance to Cu co-occur in prokaryotic communities from low and intermediately contaminated sites. The observed dissimilarity in prokaryotic structures between these communities after Cu exposure could be the result of differences in initial community composition in 41p and LAZ seawaters. To the best of our knowledge, our study provides the first evidence that TME exposure history may play a critical role in marine prokaryotic community response to recurrent metal contamination.
CONCLUSION
From our mesocosm experiences, we can conclude that harbor-relevant Cu exposure affects abundance, diversity and structure of prokaryotic communities from both low and intermediate TME-contaminated seawaters. The effect of Cu addition is visible through both the disappearance of sensitive taxa and the selection of adapted ones. We also demonstrated that TME exposure history has a strong influence on the response of prokaryotic communities to newer-current contamination, as prokaryotic communities from contaminated site were less impacted by Cu addition. Conversely, we showed limited impacts of Pb exposure compared to Cu contamination, probably due to the low toxic potential against prokaryotes and the efficiency of its resistance and detoxification mechanisms. Finally, our observations suggest both direct (toxic) and indirect (trophic) effects of TME contaminations, highlighting the need for studies of the complexity of both biotic and abiotic interactions.
FUNDING
This study was supported by IMPRECI-M2 research program funded by "Initiative Structurante Ecosphère Continentale et Côtière" (EC2CO). CC, Ph.D., was funded by the and supported by Toulon-Provence-Métrople (TPM).
ACKNOWLEDGEMENTS
The authors wish to thank the French Navy and the LASEM for diving and sampling assistance, C. Martino for building assistance of mesocosm structure (Toulon University, France) for sampling and sample treatment assistance. The authors wish also to thank the Dr Pedro Lebre to correct language and grammar.
Conflicts of interest
None declared.