-
PDF
- Split View
-
Views
-
Cite
Cite
Karen M Houghton, Carlo R Carere, Matthew B Stott, Ian R McDonald, Thermophilic methanotrophs: in hot pursuit, FEMS Microbiology Ecology, Volume 95, Issue 9, September 2019, fiz125, https://doi.org/10.1093/femsec/fiz125
- Share Icon Share
ABSTRACT
Methane is a potent greenhouse gas responsible for 20–30% of global climate change effects. The global methane budget is ∼500–600 Tg y−1, with the majority of methane produced via microbial processes, including anthropogenic-mediated sources such as ruminant animals, rice fields, sewage treatment facilities and landfills. It is estimated that microbially mediated methane oxidation (methanotrophy) consumes >50% of global methane flux each year. Methanotrophy research has primarily focused on mesophilic methanotrophic representatives and cooler environments such as freshwater, wetlands or marine habitats from which they are sourced. Nevertheless, geothermal emissions of geological methane, produced from magma and lithosphere degassing micro-seepages, mud volcanoes and other geological sources, contribute an estimated 33–75 Tg y−1 to the global methane budget. The aim of this review is to summarise current literature pertaining to the activity of thermophilic and thermotolerant methanotrophs, both proteobacterial (Methylocaldum, Methylococcus, Methylothermus) and verrucomicrobial (Methylacidiphilum). We assert, on the basis of recently reported molecular and geochemical data, that geothermal ecosystems host hitherto unidentified species capable of methane oxidation at higher temperatures.
INTRODUCTION
Methane (CH4) is a potent greenhouse gas, with atmospheric emissions a consequence of many anthropogenic and natural processes, ultimately contributing substantially to global warming. Responsible for up to 47% of global temperature response to greenhouse gases over a 10-year period, methane is 25 times more effective at absorbing infrared radiation than carbon dioxide (IPCC 2007, 2013). In the latest assessments, methane contributes to ∼16% (CO2 equivalents) of anthropogenic greenhouse gas emissions (IPCC 2014) and deep reductions in atmospheric methane emissions are needed to limit temperature increases to <1.5°C (IPCC 2018).
More than half the methane produced each year, particularly from non-anthropogenic sources, is consumed by methanotrophic bacteria or archaea that act as a biofilter prior to its reaching the atmosphere (Reeburgh 2003). The major sink for methane that does reach the atmosphere is photochemical oxidation with hydroxyl radicals in the troposphere (Nazaries et al. 2013), which removes ∼88% of atmospheric methane, while ∼7% diffuses into the stratosphere and ∼5% is taken up by soils and oxidised by methanotrophs.
Although microbes are important mediators of methane oxidation, a limited diversity of methanotrophic microorganisms and their associated metabolic pathways have been described. Indeed, the majority of research on methanotrophs and methanotrophic activity has focused on cool or ambient temperature environments with few publications linked to methanotrophy within high-temperature ecosystems (reviewed in Trotsenko et al. 2009; Semrau, DiSpirito and Yoon 2010). In the time following these publications, several high-temperature methanotroph studies have been undertaken, including the cultivation and description of at least seven thermophilic methanotrophs (Table S1, Supporting Information), and multiple molecular or geochemical studies associated with methane-rich ecosystems (Table 1). At face value, the limited number of thermophilic methanotroph studies seems to be a substantial oversight given that geological sources, including geothermal and hydrothermal fields, emit up to 75 Tg methane per year (Table S2, Supporting Information). Methane of geological origin is the fifth most abundant source of atmospheric methane, exceeded only by emissions from wetlands (177–284 Tg y−1), fossil fuels (85–105 Tg y−1), ruminants (87–94 Tg y−1) and landfills (67–90 Tg y−1) (Etiope et al. 2008; IPCC 2013). Proportionally, methane can be one of the more abundant gases in geothermal soil gas emissions, as well as in hydrothermal systems with hot springs or geysers. For example, soil gas emissions from the Awakeri geothermal field in the Bay of Plenty, New Zealand, contain upwards of 27% (v/v) methane, while emissions from some springs within the Reporoa geothermal field, also in New Zealand, contain up to 19.3% (v/v) methane (Giggenbach 1995). However, emissions in these environments can be highly variable; methane levels measured at multiple soil sites (>200) within a single geothermal field in Italy were found to vary between 0.008 and 43 kg CH4 d−1 (Cardellini et al. 2003). Considering the abundance of methane emitted from geothermal systems, it is likely that thermophilic methanotrophic microorganisms resident in geothermal ecosystems play a significant role in mitigating release of methane to the atmosphere. Despite this, a lack of described thermophilic methanotrophs or descriptions of thermophilic methanotroph ecology in the literature suggests there may be environmental or physiological determinants that restrict the in situ growth or activity of these microorganisms.
Environment . | Temperaturea . | Detection methods . | Maximum methane oxidation rate . | Methanotrophic genera identified . | Reference/accession . |
---|---|---|---|---|---|
Hydrothermal sediment, Guaymas Basin | 5–85°C | Methane oxidation (anaerobic) | 25 pmol L−1 d−1 | ND | Kallmeyer and Boetius 2004 |
Hydrothermal vent, East Pacific Rise | 50–100°C | pmoA PCR | ND | Methylothermus | Nercessian et al. 2005 |
Compost, Germany | 25–70°C | Methane oxidation, cultivation | 98.4 µmol g−1 d−1 | Methylocaldum | Jäckel, Thummes and Kämpfer 2005 |
Compost, Belgium | 10–66°C | 16S rRNA gene qPCR with type I and type II specific primers | ND | Type I methanotrophs (Gammaproteobacteria) | Halet, Boon and Verstraete 2006 |
Hydrothermal sediment, Mid Atlantic Ridge | 44–91°C | Metagenomics (16S rRNA gene) (EMP) | ND | Crenothrix, uncultured Methylococcaceae | Brazelton et al. 2010, ERP016395 |
Geothermal gas vents, Greece | 32–39°C | Methane oxidation | 11.4 nmol g−1 d−1 | ND | D'Alessandro et al. 2011 |
Hydrothermal sediment, Guaymas Basin | 4–70, 50°C | Methane oxidation (anaerobic), 16S rRNA gene and mcrA PCR | 1.2 µmol g−1 d−1 | ANME-1, ANME-2c | Holler, Widdel and Knittel 2011 |
Geothermal soil, New Zealand | 37–65°C | Methane oxidation, DNA-SIP | 7.0 µmol g−1 d−1 | Methylacidiphilum | Sharp, Stott and Dunfield 2012 |
Hot springs, Kamchatka, Russia | 47–65°C | pmoA and 16S rRNA gene qPCR | ND | Methylobacter, Methylomonas, Methylothermus | Kizilova et al. 2012 |
Hydrothermal vent, Taiwan | 49.5°C | Metagenomics (MG-RAST) | ND | Methylomarinum, Methylomicrobium | Meyer et al. 2008, MGP19574 |
Geothermal soil, Italy | 33–83, 37–80°C | pmoA PCR, methane oxidation | 2.4 µmol g−1 d−1 | Methylacidiphilum, Methylocaldum, Methylococcus, Methylocystis | Gagliano et al. 2014 |
Geothermal soil, New Zealand | 35–82, 37–65°C | pmoA and 16S rRNA gene PCR, methane oxidation, DNA-SIP | 20.4 µmol g−1 d−1 | Methylacidimicrobium, Methylacidiphilum | Sharp et al. 2014b |
Geothermal soil, Canada | 22–45°C | Methane oxidation, DNA-SIP | 99 µmol g−1 d−1 | Methylocaldum, Methylocapsa | Sharp et al. 2014a |
Hot springs, Kuril Islands, Russia | 44–99, 40–75°C | pmoA and 16S rRNA gene qPCR, methane oxidation | 104 µmol L−1 d−1 | Methylobacter, Methylococcus, Methylothermus | Kizilova et al. 2014 |
Hydrothermal vents, Norwegian Sea | <90°C | Metagenomics, metatranscriptomics, metaproteomics | ND | Methylobacter | Urich et al. 2014 |
Hot spring, Yellowstone National Park (YNP), USA | 86°C | nifH qRT-PCR (nitrogen fixation) | ND | Methylacidiphilum | Hamilton et al. 2014 |
Geothermal lake, YNP, USA | 62–66°C | Metagenomics (pmoA), 16S rRNA gene PCR | ND | Methylothermus | Inskeep et al. 2015 |
Imperial Geyser microbial mats, YNP, USA | 64–75°C | Metagenomics (16S rRNA gene) (EMP) | ND | Methylocaldum, Methylomonas | Thompson et al. 2017, ERP022167 |
Microbial mats, Rainbow Spring, YNP, USA | 42–90°C | Assembled metagenomes (IMG/M) | ND | Methylacidiphilum | Chen et al. 2019 |
Joseph's Coat hot spring, YNP, USA | ND | Assembled metagenomes (IMG/M) | ND | Methylacidiphilum | Chen et al. 2019 |
Microbial mat, Mushroom Spring, YNP, USA | 42–90°C | Assembled metagenomes (IMG/M) | ND | Methylothermus | Chen et al. 2019 |
Microbial mat, hot spring, YNP, USA | 42–90°C | Assembled metagenomes (IMG/M) | ND | Methylococcus, Methylocystis, Methylosinus | Chen et al. 2019 |
Hot spring sediment, YNP, USA | 42–90°C | Assembled metagenomes (IMG/M) | ND | Methylacidiphilum | Chen et al. 2019 |
Environment . | Temperaturea . | Detection methods . | Maximum methane oxidation rate . | Methanotrophic genera identified . | Reference/accession . |
---|---|---|---|---|---|
Hydrothermal sediment, Guaymas Basin | 5–85°C | Methane oxidation (anaerobic) | 25 pmol L−1 d−1 | ND | Kallmeyer and Boetius 2004 |
Hydrothermal vent, East Pacific Rise | 50–100°C | pmoA PCR | ND | Methylothermus | Nercessian et al. 2005 |
Compost, Germany | 25–70°C | Methane oxidation, cultivation | 98.4 µmol g−1 d−1 | Methylocaldum | Jäckel, Thummes and Kämpfer 2005 |
Compost, Belgium | 10–66°C | 16S rRNA gene qPCR with type I and type II specific primers | ND | Type I methanotrophs (Gammaproteobacteria) | Halet, Boon and Verstraete 2006 |
Hydrothermal sediment, Mid Atlantic Ridge | 44–91°C | Metagenomics (16S rRNA gene) (EMP) | ND | Crenothrix, uncultured Methylococcaceae | Brazelton et al. 2010, ERP016395 |
Geothermal gas vents, Greece | 32–39°C | Methane oxidation | 11.4 nmol g−1 d−1 | ND | D'Alessandro et al. 2011 |
Hydrothermal sediment, Guaymas Basin | 4–70, 50°C | Methane oxidation (anaerobic), 16S rRNA gene and mcrA PCR | 1.2 µmol g−1 d−1 | ANME-1, ANME-2c | Holler, Widdel and Knittel 2011 |
Geothermal soil, New Zealand | 37–65°C | Methane oxidation, DNA-SIP | 7.0 µmol g−1 d−1 | Methylacidiphilum | Sharp, Stott and Dunfield 2012 |
Hot springs, Kamchatka, Russia | 47–65°C | pmoA and 16S rRNA gene qPCR | ND | Methylobacter, Methylomonas, Methylothermus | Kizilova et al. 2012 |
Hydrothermal vent, Taiwan | 49.5°C | Metagenomics (MG-RAST) | ND | Methylomarinum, Methylomicrobium | Meyer et al. 2008, MGP19574 |
Geothermal soil, Italy | 33–83, 37–80°C | pmoA PCR, methane oxidation | 2.4 µmol g−1 d−1 | Methylacidiphilum, Methylocaldum, Methylococcus, Methylocystis | Gagliano et al. 2014 |
Geothermal soil, New Zealand | 35–82, 37–65°C | pmoA and 16S rRNA gene PCR, methane oxidation, DNA-SIP | 20.4 µmol g−1 d−1 | Methylacidimicrobium, Methylacidiphilum | Sharp et al. 2014b |
Geothermal soil, Canada | 22–45°C | Methane oxidation, DNA-SIP | 99 µmol g−1 d−1 | Methylocaldum, Methylocapsa | Sharp et al. 2014a |
Hot springs, Kuril Islands, Russia | 44–99, 40–75°C | pmoA and 16S rRNA gene qPCR, methane oxidation | 104 µmol L−1 d−1 | Methylobacter, Methylococcus, Methylothermus | Kizilova et al. 2014 |
Hydrothermal vents, Norwegian Sea | <90°C | Metagenomics, metatranscriptomics, metaproteomics | ND | Methylobacter | Urich et al. 2014 |
Hot spring, Yellowstone National Park (YNP), USA | 86°C | nifH qRT-PCR (nitrogen fixation) | ND | Methylacidiphilum | Hamilton et al. 2014 |
Geothermal lake, YNP, USA | 62–66°C | Metagenomics (pmoA), 16S rRNA gene PCR | ND | Methylothermus | Inskeep et al. 2015 |
Imperial Geyser microbial mats, YNP, USA | 64–75°C | Metagenomics (16S rRNA gene) (EMP) | ND | Methylocaldum, Methylomonas | Thompson et al. 2017, ERP022167 |
Microbial mats, Rainbow Spring, YNP, USA | 42–90°C | Assembled metagenomes (IMG/M) | ND | Methylacidiphilum | Chen et al. 2019 |
Joseph's Coat hot spring, YNP, USA | ND | Assembled metagenomes (IMG/M) | ND | Methylacidiphilum | Chen et al. 2019 |
Microbial mat, Mushroom Spring, YNP, USA | 42–90°C | Assembled metagenomes (IMG/M) | ND | Methylothermus | Chen et al. 2019 |
Microbial mat, hot spring, YNP, USA | 42–90°C | Assembled metagenomes (IMG/M) | ND | Methylococcus, Methylocystis, Methylosinus | Chen et al. 2019 |
Hot spring sediment, YNP, USA | 42–90°C | Assembled metagenomes (IMG/M) | ND | Methylacidiphilum | Chen et al. 2019 |
In situ temperature shown for molecular detection methods, and incubation temperatures for methane oxidation rates. ND, not determined; EMP, Earth Microbiome Project; MG-RAST, Metagenomic Rapid Annotations using Subsystems Technology; IMG/M, Integrated Microbial Genomes and Microbiomes.
Environment . | Temperaturea . | Detection methods . | Maximum methane oxidation rate . | Methanotrophic genera identified . | Reference/accession . |
---|---|---|---|---|---|
Hydrothermal sediment, Guaymas Basin | 5–85°C | Methane oxidation (anaerobic) | 25 pmol L−1 d−1 | ND | Kallmeyer and Boetius 2004 |
Hydrothermal vent, East Pacific Rise | 50–100°C | pmoA PCR | ND | Methylothermus | Nercessian et al. 2005 |
Compost, Germany | 25–70°C | Methane oxidation, cultivation | 98.4 µmol g−1 d−1 | Methylocaldum | Jäckel, Thummes and Kämpfer 2005 |
Compost, Belgium | 10–66°C | 16S rRNA gene qPCR with type I and type II specific primers | ND | Type I methanotrophs (Gammaproteobacteria) | Halet, Boon and Verstraete 2006 |
Hydrothermal sediment, Mid Atlantic Ridge | 44–91°C | Metagenomics (16S rRNA gene) (EMP) | ND | Crenothrix, uncultured Methylococcaceae | Brazelton et al. 2010, ERP016395 |
Geothermal gas vents, Greece | 32–39°C | Methane oxidation | 11.4 nmol g−1 d−1 | ND | D'Alessandro et al. 2011 |
Hydrothermal sediment, Guaymas Basin | 4–70, 50°C | Methane oxidation (anaerobic), 16S rRNA gene and mcrA PCR | 1.2 µmol g−1 d−1 | ANME-1, ANME-2c | Holler, Widdel and Knittel 2011 |
Geothermal soil, New Zealand | 37–65°C | Methane oxidation, DNA-SIP | 7.0 µmol g−1 d−1 | Methylacidiphilum | Sharp, Stott and Dunfield 2012 |
Hot springs, Kamchatka, Russia | 47–65°C | pmoA and 16S rRNA gene qPCR | ND | Methylobacter, Methylomonas, Methylothermus | Kizilova et al. 2012 |
Hydrothermal vent, Taiwan | 49.5°C | Metagenomics (MG-RAST) | ND | Methylomarinum, Methylomicrobium | Meyer et al. 2008, MGP19574 |
Geothermal soil, Italy | 33–83, 37–80°C | pmoA PCR, methane oxidation | 2.4 µmol g−1 d−1 | Methylacidiphilum, Methylocaldum, Methylococcus, Methylocystis | Gagliano et al. 2014 |
Geothermal soil, New Zealand | 35–82, 37–65°C | pmoA and 16S rRNA gene PCR, methane oxidation, DNA-SIP | 20.4 µmol g−1 d−1 | Methylacidimicrobium, Methylacidiphilum | Sharp et al. 2014b |
Geothermal soil, Canada | 22–45°C | Methane oxidation, DNA-SIP | 99 µmol g−1 d−1 | Methylocaldum, Methylocapsa | Sharp et al. 2014a |
Hot springs, Kuril Islands, Russia | 44–99, 40–75°C | pmoA and 16S rRNA gene qPCR, methane oxidation | 104 µmol L−1 d−1 | Methylobacter, Methylococcus, Methylothermus | Kizilova et al. 2014 |
Hydrothermal vents, Norwegian Sea | <90°C | Metagenomics, metatranscriptomics, metaproteomics | ND | Methylobacter | Urich et al. 2014 |
Hot spring, Yellowstone National Park (YNP), USA | 86°C | nifH qRT-PCR (nitrogen fixation) | ND | Methylacidiphilum | Hamilton et al. 2014 |
Geothermal lake, YNP, USA | 62–66°C | Metagenomics (pmoA), 16S rRNA gene PCR | ND | Methylothermus | Inskeep et al. 2015 |
Imperial Geyser microbial mats, YNP, USA | 64–75°C | Metagenomics (16S rRNA gene) (EMP) | ND | Methylocaldum, Methylomonas | Thompson et al. 2017, ERP022167 |
Microbial mats, Rainbow Spring, YNP, USA | 42–90°C | Assembled metagenomes (IMG/M) | ND | Methylacidiphilum | Chen et al. 2019 |
Joseph's Coat hot spring, YNP, USA | ND | Assembled metagenomes (IMG/M) | ND | Methylacidiphilum | Chen et al. 2019 |
Microbial mat, Mushroom Spring, YNP, USA | 42–90°C | Assembled metagenomes (IMG/M) | ND | Methylothermus | Chen et al. 2019 |
Microbial mat, hot spring, YNP, USA | 42–90°C | Assembled metagenomes (IMG/M) | ND | Methylococcus, Methylocystis, Methylosinus | Chen et al. 2019 |
Hot spring sediment, YNP, USA | 42–90°C | Assembled metagenomes (IMG/M) | ND | Methylacidiphilum | Chen et al. 2019 |
Environment . | Temperaturea . | Detection methods . | Maximum methane oxidation rate . | Methanotrophic genera identified . | Reference/accession . |
---|---|---|---|---|---|
Hydrothermal sediment, Guaymas Basin | 5–85°C | Methane oxidation (anaerobic) | 25 pmol L−1 d−1 | ND | Kallmeyer and Boetius 2004 |
Hydrothermal vent, East Pacific Rise | 50–100°C | pmoA PCR | ND | Methylothermus | Nercessian et al. 2005 |
Compost, Germany | 25–70°C | Methane oxidation, cultivation | 98.4 µmol g−1 d−1 | Methylocaldum | Jäckel, Thummes and Kämpfer 2005 |
Compost, Belgium | 10–66°C | 16S rRNA gene qPCR with type I and type II specific primers | ND | Type I methanotrophs (Gammaproteobacteria) | Halet, Boon and Verstraete 2006 |
Hydrothermal sediment, Mid Atlantic Ridge | 44–91°C | Metagenomics (16S rRNA gene) (EMP) | ND | Crenothrix, uncultured Methylococcaceae | Brazelton et al. 2010, ERP016395 |
Geothermal gas vents, Greece | 32–39°C | Methane oxidation | 11.4 nmol g−1 d−1 | ND | D'Alessandro et al. 2011 |
Hydrothermal sediment, Guaymas Basin | 4–70, 50°C | Methane oxidation (anaerobic), 16S rRNA gene and mcrA PCR | 1.2 µmol g−1 d−1 | ANME-1, ANME-2c | Holler, Widdel and Knittel 2011 |
Geothermal soil, New Zealand | 37–65°C | Methane oxidation, DNA-SIP | 7.0 µmol g−1 d−1 | Methylacidiphilum | Sharp, Stott and Dunfield 2012 |
Hot springs, Kamchatka, Russia | 47–65°C | pmoA and 16S rRNA gene qPCR | ND | Methylobacter, Methylomonas, Methylothermus | Kizilova et al. 2012 |
Hydrothermal vent, Taiwan | 49.5°C | Metagenomics (MG-RAST) | ND | Methylomarinum, Methylomicrobium | Meyer et al. 2008, MGP19574 |
Geothermal soil, Italy | 33–83, 37–80°C | pmoA PCR, methane oxidation | 2.4 µmol g−1 d−1 | Methylacidiphilum, Methylocaldum, Methylococcus, Methylocystis | Gagliano et al. 2014 |
Geothermal soil, New Zealand | 35–82, 37–65°C | pmoA and 16S rRNA gene PCR, methane oxidation, DNA-SIP | 20.4 µmol g−1 d−1 | Methylacidimicrobium, Methylacidiphilum | Sharp et al. 2014b |
Geothermal soil, Canada | 22–45°C | Methane oxidation, DNA-SIP | 99 µmol g−1 d−1 | Methylocaldum, Methylocapsa | Sharp et al. 2014a |
Hot springs, Kuril Islands, Russia | 44–99, 40–75°C | pmoA and 16S rRNA gene qPCR, methane oxidation | 104 µmol L−1 d−1 | Methylobacter, Methylococcus, Methylothermus | Kizilova et al. 2014 |
Hydrothermal vents, Norwegian Sea | <90°C | Metagenomics, metatranscriptomics, metaproteomics | ND | Methylobacter | Urich et al. 2014 |
Hot spring, Yellowstone National Park (YNP), USA | 86°C | nifH qRT-PCR (nitrogen fixation) | ND | Methylacidiphilum | Hamilton et al. 2014 |
Geothermal lake, YNP, USA | 62–66°C | Metagenomics (pmoA), 16S rRNA gene PCR | ND | Methylothermus | Inskeep et al. 2015 |
Imperial Geyser microbial mats, YNP, USA | 64–75°C | Metagenomics (16S rRNA gene) (EMP) | ND | Methylocaldum, Methylomonas | Thompson et al. 2017, ERP022167 |
Microbial mats, Rainbow Spring, YNP, USA | 42–90°C | Assembled metagenomes (IMG/M) | ND | Methylacidiphilum | Chen et al. 2019 |
Joseph's Coat hot spring, YNP, USA | ND | Assembled metagenomes (IMG/M) | ND | Methylacidiphilum | Chen et al. 2019 |
Microbial mat, Mushroom Spring, YNP, USA | 42–90°C | Assembled metagenomes (IMG/M) | ND | Methylothermus | Chen et al. 2019 |
Microbial mat, hot spring, YNP, USA | 42–90°C | Assembled metagenomes (IMG/M) | ND | Methylococcus, Methylocystis, Methylosinus | Chen et al. 2019 |
Hot spring sediment, YNP, USA | 42–90°C | Assembled metagenomes (IMG/M) | ND | Methylacidiphilum | Chen et al. 2019 |
In situ temperature shown for molecular detection methods, and incubation temperatures for methane oxidation rates. ND, not determined; EMP, Earth Microbiome Project; MG-RAST, Metagenomic Rapid Annotations using Subsystems Technology; IMG/M, Integrated Microbial Genomes and Microbiomes.
In this review, we summarise what is currently known of the diversity, ecological activity and metabolic adaptations of thermophilic and thermotolerant methanotrophs. We further explore some possible reasons explaining the lack of described high-temperature methanotroph isolates and finally argue that geothermal and hydrothermal environments are likely to support as-yet-undescribed methanotrophs that are active in the reduction of methane release from these ecosystems.
METHANE OXIDATION—AN OVERVIEW OF METHANOTROPHS
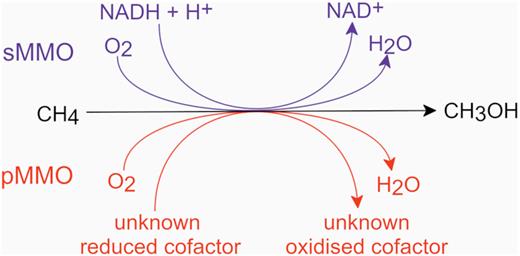
A simplified diagram of the methane oxidation step in aerobic methanotrophs. NADH, reduced nicotinamide adenine dinucleotide; NAD, oxidised nicotinamide adenine dinucleotide.
Methanol is then sequentially oxidised via formaldehyde and formate through to carbon dioxide (Fig. 2). For the majority of aerobic methanotrophs, cellular carbon can be assimilated from formaldehyde or formate (converted into methylene tetrahydrofolate) via the ribulose monophosphate or serine cycle pathways, respectively (Bowman 2006; Chistoserdova 2011). In contrast, anaerobic methanotrophy is the application of a reverse methanogenesis process coupled with a non-oxygen terminal electron acceptor such as nitrate or sulphate (Cui et al. 2015) to drive metabolism. Anaerobic methanotrophs assimilate carbon from either methane or carbon dioxide (Wegener et al. 2008; Kellermann et al. 2012).
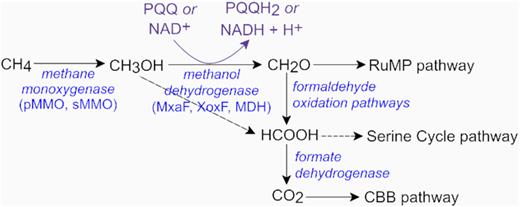
A simplified schematic of the major substrates and intermediates in the dissimilatory methanol oxidation pathways in aerobic methanotrophs. The XoxF enzyme from Methylacidiphilum fumariolicum oxidises methanol directly into formate (Keltjens et al. 2014). Formate can be converted into methylene tetrahydrofolate and assimilated via the serine pathway (Chistoserdova 2011). Most methanotrophs possess multiple formaldehyde oxidation systems and formate dehydrogenase enzymes (Chistoserdova 2011). NAD, oxidised nicotinamide adenine dinucleotide; NADH, reduced nicotinamide adenine dinucleotide; PQQ, pyrroloquinoline quinone; PQQH2, reduced pyrroloquinoline quinone; RuMP, ribulose monophosphate; CBB, Calvin–Benson–Bassham.
The known taxonomic diversity of methane oxidising microorganisms is limited to three phyla within the Bacteria and one phylum within the Archaea. Methanotrophic bacteria employing a molecular oxygen-catalysed methane oxidation pathway include Proteobacteria (classes Alphaproteobacteria and Gammaproteobacteria), Verrucomicrobia (genus Methylacidiphilum) and candidate phylum NC10 ‘Methylomirabilaeota’ (Table S1, Supporting Information). The proteobacterial methanotrophs have the greatest taxonomic representation of described strains, and are typically characterised by neutrophilic, moderately acidic or moderately alkaliphilic phenotypes displaying an archetypical pathway for methane oxidation yielding carbon dioxide, and carbon assimilation via ribulose monophosphate, serine cycle and/or Calvin–Benson–Bassham pathways.
In contrast, the verrucomicrobial strains are obligately acidophilic and assimilate carbon by the fixation of carbon dioxide via the Calvin–Benson–Bassham cycle (Khadem et al. 2011) (Fig. 1). Finally, Candidatus Methylomirabilis oxyfera, Candidatus Methylomirabilis sinica and Candidatus Methylomirabilis lanthanidiphila, the only cultured methanotrophs from candidate phylum NC10, are found in methane-replete, but anaerobic environments (Ettwig et al. 2010; He et al. 2016; Versantvoort et al. 2018). Despite these oxygen-free conditions, Ca. Methylomirabilis strains employ a novel variation of the typical aerobic methane oxidation pathway by coupling methane oxidation with nitrite reduction and then dismutation of nitric oxide to generate intracellular dinitrogen and oxygen gases. Oxygen is then shuttled into a standard aerobic methanotrophy pathway as a terminal respiratory electron acceptor and as an oxidant for MMO (Ettwig et al. 2010). Ca. Methylomirabilis strains have thus far been enriched from exclusively mesophilic environments ≤30°C (He et al. 2015, 2016; Versantvoort et al. 2018). Although almost all aerobic methanotrophs are obligately methylotrophic, a small number of strains including Methylomicrobium agile and Methylomonas aurantiaca (Bowman et al. 1993), and some Methylocella species (Semrau, DiSpirito and Vuilleumier 2011) are able to utilise mono-, di- and/or trimethylamine. More recently, mixotrophy amongst methanotrophs has been described, with some members of the family Beijerinckiaceae (Alphaproteobacteria) able to utilise multi-carbon substrates including acetate, pyruvate, succinate, malate or ethanol (Dedysh, Knief and Dunfield 2005; Dunfield et al. 2010; Belova et al. 2013), and Methylocella silvestris and Methylocella tundrae are capable of simultaneous methane and propane oxidation (Crombie and Murrell 2014; Kox et al. 2019). At least two species of Methylacidiphilum have been shown to respire molecular hydrogen (Carere et al. 2017; Mohammadi et al. 2017a), and molecular hydrogen has also been shown to be a source of reducing power for methane monooxygenases in Methylosinus trichosporium and Methylococcus capsulatus (Shah et al. 1995; Hanczár et al. 2002).
The anaerobic methane-oxidising strains (ANME) in the archaeal phylum Euryarchaeota (class Methanomicrobia) perform methane oxidation via reverse methanogenesis (i.e. a reversal of CO2 reduction) (Timmers et al. 2017). Although ANME often form consortia using extracellular electron transfer with sulphate-reducing bacteria such as Desulfosarcina, Desulfococcus and Desulfobulbus, representatives within all three clades (ANME-1, -2 and -3) have also been detected without bacterial partners (Cui et al. 2015; Skennerton et al. 2017). Anaerobic methane oxidisers and/or their associated consortia can use alternative terminal electron acceptors, including ferric iron or manganese (Beal, House and Orphan 2009; Cai et al. 2018), sulphate (Milucka et al. 2012) or nitrate (Haroon et al. 2013). To date, no ANME methanotrophs have been isolated (Cui et al. 2015). Although ANME consortia are more frequently detected in mesophilic or psychrophilic marine and freshwater environments (Cui et al. 2015), anaerobic methane oxidation has been observed in hydrothermal sediments at temperatures up to 90°C (Wankel et al. 2012). In addition to ANME consortia undertaking anaerobic oxidation of methane, there has been some recent conjecture over whether members of the Bathyarchaeota (formerly Miscellaneous Crenarchaeotal Group) are capable of this metabolic phenotype (Biddle et al. 2006; Evans et al. 2015; Vanwonterghem et al. 2016). The current consensus indicates that Bathyarchaeota strains are predominately heterotrophic, displaying carbohydrate and amino acid fermentative (Dombrowski et al. 2017; Maus et al. 2018) and/or alkanotrophic (propane, butane) (Evans et al. 2019) metabolisms and are unlikely to undertake dissimilatory anaerobic methanotrophy (Zhou et al. 2018; Evans et al. 2019). Nevertheless, a number of ecological observations in hydrothermal vent systems show Bathyarchaeota as the dominating archaeon in systems energetically favouring anaerobic oxidation of methane and leave open the possibility of future discoveries of bathyarchaeotal-driven methane oxidation. Methanotrophic archaea have recently been reviewed (McGlynn 2017; Timmers et al. 2017; He et al. 2018) and are not discussed further in this review.
THERMOPHILIC METHANOTROPHS
The majority of the described aerobic methanotrophs are mesophilic, growing optimally at temperatures between 10 and 35°C (Bowman et al. 1993), and at circumneutral pH. For the purposes of this review, we define ‘thermotolerant’ strains as displaying a maximum growth temperature (Tmax) between 42 and 50°C and ‘thermophilic’ strains as exhibiting an optimum growth temperature (Topt) >40°C. The first described thermotolerant methanotroph, M. capsulatus strain Texas, was isolated in 1966 from sewage sludge (Foster and Davis 1966), and displayed a Topt and Tmax of 37 and 50°C, respectively. A second thermotolerant strain, M. capsulatus Bath, was isolated from a geothermally heated Roman bath in Bath, UK, and subsequently became one of the most well-studied methanotrophs in terms of genetics, physiology and ecology (Stainthorpe et al. 1989; Zahn and Dispirito 1996; Ward et al. 2004). More than 30 years passed before the first truly thermophilic methanotroph was isolated from a hot spring in Hungary (Bodrossy et al. 1999). This methanotroph, Methylothermus strain HB, was reported to grow at temperatures up to 72°C with a Topt of 55°C (Bodrossy et al. 1999); however, the strain was unable to be maintained and is no longer extant. At the time of writing this review, only 21 thermotolerant or thermophilic methanotrophs have been described (Fig. 3), the majority of which were isolated from geothermal soils or hot springs. In comparison, >50 described mesophilic or psychrophilic strains have been reported (Table S1, Supporting Information).
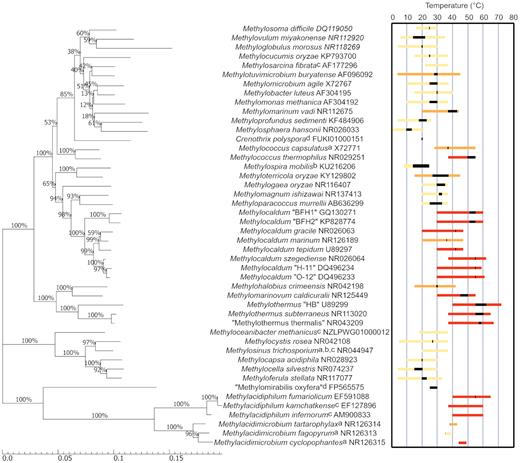
Molecular phylogenetic analysis of 16S rRNA gene sequences from aerobic methanotrophs. The reported growth temperature ranges and Topt values (black bar) for thermotolerant and thermophilic strains have been highlighted. Yellow bars indicate mesophilic strains (Tmax < 42°C), orange bars indicate thermotolerant strains (42°C < Tmax < 50°C) and red bars indicate thermophilic strains (Topt > 40°C). Dashed lines indicate where an upper or lower temperature limit is not known. a, no Tmin published; b, no Tmax published; c, no Topt published; d, only growth temperature published. The evolutionary history was inferred using the neighbour-joining method (Saitou and Nei 1987) and the evolutionary distances were computed using the maximum composite likelihood method (Tamura, Nei and Kumar 2004). The percentage of replicate trees in which the associated taxa clustered together in the bootstrap test (1000 replicates) is shown next to the branches. The scale bar represents nucleotide substitutions per site. Evolutionary analyses were conducted in MEGA7 (Kumar, Stecher and Tamura 2016).
Thermophilic methanotroph strains form discrete phylogenetic clusters separate from mesophilic methanotrophs, with two distinct groups clustered around the families Methylothermaceae and Methylacidiphilaceae and the genus Methylocaldum (Fig. 3). The family Methylothermaceae (Hirayama et al. 2014; Tindall 2019), within the Gammaproteobacteria, includes three thermophilic species, Methylothermus subterraneus (37–65°C) (Hirayama et al. 2011), ‘Methylothermus thermalis’ (37–67°C) (Tsubota et al. 2005) and Methylomarinovum caldicuralii (30–55°C) (Hirayama et al. 2014), and the thermotolerant species Methylohalobius crimeensis (15–42°C)(Heyer et al. 2005). All four strains use methane or methanol as their sole source of energy and the thermophilic strains were each enriched from hydrothermal sources, while in contrast M. crimeensis was isolated from a hypersaline lake. The genus Methylocaldum includes multiple thermophilic and one thermotolerant species (Fig. 3) that were isolated from both low-temperature (freshwater, soil) and heated environments (hot springs and marine hydrothermal systems). There are currently only two methanotrophic genera within the phylum Verrucomicrobia, Methylacidiphilum and Methylacidimicrobium. All three described strains of Methylacidiphilum were isolated from geothermal soil or hot springs and are thermophilic (growth at up to 65°C) (Op den Camp et al. 2009), and two of the four isolates of Methylacidimicrobium are thermotolerant or thermophilic (growth at up to 49°C) (van Teeseling et al. 2014; Sharp et al. 2014b). There are currently no cultivated methanotrophs with a growth optimum >60°C.
EVIDENCE FOR METHANOTROPHS AND METHANOTROPHIC ACTIVITY IN HIGH-TEMPERATURE ENVIRONMENTS
Molecular surveys
Genes encoding subunits of both pMMO and sMMO have been used as a molecular target for detecting methanotrophs in environmental surveys. The pmoA gene, encoding the large subunit of the particulate MMO, is most commonly used and displays good congruency with 16S rRNA gene-based phylogenetic trees (Fig. 4). Molecular surveys have detected the presence of pmoA in multiple high-temperature environments (Table 1). In general, few methanotroph taxa were identified in these studies with many of the pmoA and corresponding 16S rRNA gene sequences detected being most closely related to Methylacidiphilum, Methylocaldum and Methylothermus species. However, there are caveats to consider when interpreting ecological signals derived from pmoA amplifications. pMMO is evolutionarily related to ammonia monooxygenase and consequently the genes pmoA and amoA share significant homology, which can lead to co-amplification of amoA when applying standard pmoA primers (Dumont et al. 2014). Both bacterial (Lopez-Vazquez et al. 2014) and archaeal (De La Torre et al. 2008) ammonia oxidisers are capable of growth and nitrification at elevated temperatures, and may be present in geothermal areas (Abby et al. 2018). Additionally, substantial sequence variation exists within pmoA genes (Wang et al. 2016; Ghashghavi, Jetten and Lüke 2017) meaning that standard pmoA primers are unlikely to detect the more divergent pmoA genes, as highlighted in several studies (Gagliano et al. 2014; Kizilova et al. 2014; Sharp et al. 2014b). Further confounding environmental methanotroph detection, most strains encode multiple disparate copies of the gene (Op den Camp et al. 2009; Khadka et al. 2018), which may overestimate methanotroph diversity and abundance, while the alphaproteobacterial genera Methylocella, Methyloferula and one member of Methyloceanibacter express only sMMO and cannot be detected with pmoA primers (Vekeman et al. 2016). Metagenomic studies using shotgun sequencing, which avoids the known issues with primer bias, have now been undertaken, and assembled metagenomes containing pmoA have been constructed from several hot springs in YNP and a hydrothermal vent off the coast of Taiwan (Table 1).
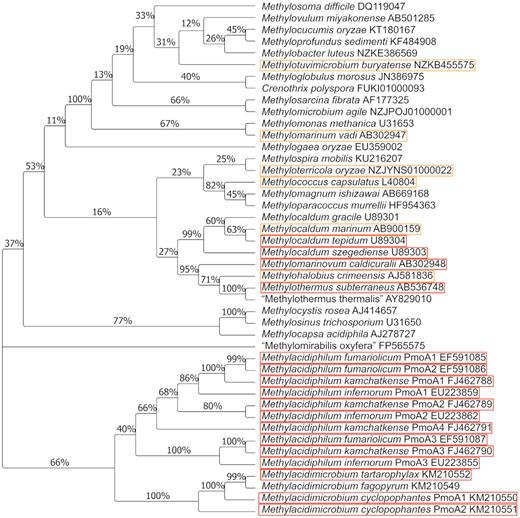
Phylogenetic relationships between described methanotrophs based on deduced PmoA sequences. Thermotolerant strains are highlighted in orange, and thermophilic isolates in red. The evolutionary history was inferred using the neighbour-joining method (Saitou and Nei 1987). The percentage of replicate trees in which the associated taxa clustered together in the bootstrap test (1000 replicates) is shown next to the branches. The evolutionary distances were calculated using the Poisson correction method (Zuckerkandl and Pauling 1965). Evolutionary analyses were conducted in MEGA7 (Kumar, Stecher and Tamura 2016).
The presence of sMMO in environmental surveys is detected using primers targeting the mmoX gene (McDonald, Kenna and Murrell 1995). However, the use of mmoX gene to probe for methanotrophy has limited utility when assessing community structure in high-temperature ecosystems as the majority of known methanotrophs contain pMMO but not sMMO. Aside from in mesophilic strains, the soluble form of MMO has thus far only been identified in thermotolerant strains (Methylotuvimicrobium buryatense, M. capsulatus and Methylocaldum marinum; Colby, Stirling and Dalton 1977; Kalyuzhnaya et al. 2001; Takeuchi et al. 2014) and is not encoded in any known thermophilic strain.
Non-MMO functional gene markers constituting components of the aerobic methanotrophic metabolic pathway, particularly methanol dehydrogenase, may be useful for detecting methanotrophs in situ. However, as these genes are not exclusive to this metabolic guild, this strategy is non-specific and will detect non-methanotrophs as well. For example, mxaF encodes the large subunit of methanol dehydrogenase and although it appears to be universally distributed in proteobacterial methanotrophs (Lau et al. 2013), including the thermophilic Methylocaldum (Islam et al. 2016), it is also found in Gram-negative methylotrophs (Holmes, Owens and Murrell 1995). Many methylotrophs and methanotrophs also possess the xoxF gene, an orthologue of mxaF (Chistoserdova 2011), encoding a polypeptide with ∼50% amino acid identity to MxaF (Schmidt et al. 2010). XoxF is reported to have methanol dehydrogenase activity dependent on lanthanide metals as a co-factor (Pol et al. 2013). This is the preferentially expressed methanol dehydrogenase in the thermotolerant M. buryatense (Chu and Lidstrom 2016) and the thermophilic Methylacidiphilum strains (Hou et al. 2008; Khadem et al. 2012b) when even low concentrations of lanthanide are present. There are at least 22 clades of the XoxF gene product (Chistoserdova 2016) and PCR primers have been designed to target the five major phylogenetic groups (Taubert et al. 2015). However, several methanotrophs and methylotrophs have been shown to contain genes from different XoxF clades (Chistoserdova 2016; Huang, Yu and Chistoserdova 2018), indicating that xoxF may not be suitable for quantifying methanotrophs in situ. No molecular surveys have been conducted in high-temperature environments using either mxaF or xoxF marker genes.
A few temporal studies have been performed in high-temperature environments that have reported evidence of methanotrophy. These include a 10-week study of compost, which reported an increase in the activity of gammaproteobacterial methanotrophs during thermophilic phases of compost maturation (45–70°C); unfortunately, no sequencing of the resulting DGGE bands was undertaken (Halet et al. 2006). A combined metatranscriptome and metaproteome approach to analyse sediments at a diffuse hydrothermal vent found expression of pmoA from a strain most similar to Methylobacter tundripaludum, as well as mcrA (encoding a subunit of methyl coenzyme M reductase, the enzyme catalysing the terminal step in methanogenesis) from Archaea within the order Methanomicrobiales (Urich et al. 2014). Finally, a study of the occurrence of ammonia oxidation within an 84°C hot spring in YNP classified 6% of transcripts to the nifH nitrogenase subunit belonging to a strain similar to that of the verrucomicrobial methanotroph M. fumariolicum (Hamilton et al. 2014).
It is important to note that methanotroph diversity in high-temperature environments may not be well represented by the studies shown in Table 1. Environmental studies based upon 16S rRNA or pmoA gene sequences to report methanotroph diversity in thermal environments are often constrained by the knowledge of characterised methanotroph taxa from predominately non-thermal (i.e. cooler) habitats. For example, the thermophilic and acidophilic Methylacidiphilum spp. were only isolated and characterised in 2007, prior to which methanotrophy within Verrucomicrobia was unknown (Dunfield et al. 2007; Pol et al. 2007; Islam et al. 2008). Although some uncultured Methylococcaceae were identified in one study (Brazelton et al. 2010), more diverse sequences from unknown methanotrophs would not be annotated as such.
Geochemical methods for detecting methanotroph activity
A key method for detecting methanotrophic activity, either in situ or in the laboratory, is to assess methane oxidation rates via gas chromatography. This has been demonstrated using various samples from geothermally heated soils in Canada (45°C) (Sharp et al. 2014a), Italy (50°C) (Gagliano et al. 2014), New Zealand (68°C) (Sharp et al. 2014b) and from hot springs in Russia (74°C) (Kizilova et al. 2014) (Table 1). In most studies, rates of methane oxidation were correlated to the presence of known methanotrophs using 16S rRNA or pmoA gene sequencing. The rates of methane oxidation in the majority of these high-temperature studies were in the range of µmol CH4 g−1 d−1, which is an order of magnitude greater than rates often described for peat bogs or forest soils (Reeburgh 2003; Zeng et al. 2019), or lake sediments (Bornemann et al. 2016). Methane oxidation rates in wetlands have been measured at up to a maximum of 17.3 µmol CH4 g−1 d−1 (Esson et al. 2016; Wang et al. 2018; Zhang et al. 2019), but these are still substantially less than values reported in some geothermal soils (99 µmol CH4 g−1 d−1; Sharp et al. 2014a), indicating the activity of methanotrophs in mitigating methane emissions from these environments. Hot springs have lower rates of methane oxidation (e.g. 104 nmol CH4 mL−1 d−1; Kizilova et al. 2014), due to the lower solubility of methane at high temperatures. In addition, anaerobic hydrothermal sediments from the Guaymas Basin were shown to oxidise methane at 70 and 85°C (Kallmeyer and Boetius 2004; Holler et al. 2011). In situ gas flux measurements (Urmann et al. 2005; Henneberger et al. 2013) have yet to be undertaken in high-temperature environments, but these could give more confidence to results seen with these types of ex situ laboratory experiments.
Stable isotope probing (SIP) and radioactive isotope probing can also be used to identify active microorganisms within thermal environments. SIP is conducted by providing a stable isotope substrate that is heavier than the naturally occurring isotope (e.g. 13C-methane), into a microcosm thought to contain methanotrophs. Labelled substrate is taken up and incorporated into cellular biomass, and can then be separated from the ‘light’ (or unlabelled) fractions via density gradient centrifugation (Dumont and Murrell 2005). DNA-SIP can be used in conjunction with sequencing of either 16S rRNA or functional genes in order to elucidate the genetic potential of an ecosystem, and has been used to identify active methanotrophs in, for example, peat bogs (Esson et al. 2016), soils (Radajewski et al. 2002), groundwater (Hutchens et al. 2004) and lake sediments (Dumont, Pommerenke and Casper 2013). A few methanotroph-targeted SIP studies have been conducted in high-temperature environments, and in these the activity of methanotrophs was confirmed in geothermal soils located in Canada (Sharp et al. 2014a) and New Zealand (Sharp, Stott and Dunfield 2012) (Table 1). Radioactive tracers (e.g. 14C-methane) have been used to measure assimilation of 14C into biomass and carbon dioxide production from water and sediments sampled from hot springs in Russia at 75°C (Kizilova et al. 2014), and anaerobic hydrothermal sediments from Guaymas Basin at 60°C (Kallmeyer and Boetius 2004) and 70°C (Holler et al. 2011). Using a combination of these molecular and biogeochemical techniques, methanotrophs or methane-oxidising activity has been detected in a variety of high-temperature ecosystems, but few thermophilic methanotrophs have been isolated (Fig. 3). Methanotrophs are difficult to obtain in pure culture (Bowman 2006) due to relatively slow growth rates (Jiang et al. 2010) and generally low affinity for methane (Bowman 2011). In addition, many methanotrophic strains are unable to grow on agar surfaces (Rahalkar, Bussmann and Schink 2007), and enrichment is often heavily contaminated with non-methanotrophic (and often methylotrophic) bacteria (Bowman 2011). Another complicating issue for liquid cultures at elevated temperatures is the poor methane and oxygen solubility and consequent reduction in gas–liquid mass transfer. Methane solubility decreases from 19.2 mg kg−1 in water at 30°C to 12.8 mg kg−1 in water at 60°C (Duan et al. 1992), and over the same temperature scale, oxygen solubility decreases by 37% (Ramsing and Gundersen 1994).
The temperature stability and activity of the enzymes involved in the complete oxidation of methane have been under-reported and bear some consideration with respect to thermophilic methanotrophy. All known aerobic methanotrophs use a homologous metabolic pathway for methane oxidation (Rxn 1). In order for metabolism to proceed, each of the enzymes in these sequential steps must be stable and active at high temperatures in thermotolerant or thermophilic methanotrophs for growth to occur. We address potential metabolic bottlenecks for thermophilic methanotrophy next.
The initial conversion of methane to methanol (Rxn 2) is catalysed by either a soluble or membrane-bound form of methane monooxygenase. In cultures of M. capsulatus, sMMO displayed no detectable loss of activity following heat shock (65°C) for 1 h (Bodrossy et al. 1995), but there are no reports on the thermal stability or activity of pMMO, as it is difficult to obtain a purified form of the enzyme (Deng, Ro and Rosenzweig 2018). However, as pMMO displays significant homology to ammonia monooxygenase (Holmes et al. 1995), it is plausible to infer temperature stability and activity via thermophilic ammonia oxidisers. Indeed, the ammonia-oxidising archaeon Ca. ‘Nitrosocaldus yellowstonii’ grows at temperatures up to 74°C, suggesting that the structurally similar pMMO may also be stable at higher temperatures. In addition, many methanotrophs encode multiple pMMO isozymes (Yimga et al. 2003; Tavormina et al. 2011), some of which have been shown to have different affinities for methane (Baani and Liesack 2008; Erikstad et al. 2012), or differential expression in response to ammonia (Dam et al. 2014; Mohammadi et al. 2017b) or oxygen levels (Khadem et al. 2012a). It is conceivable that this metabolic flexibility may enable methanotrophs to respond to fluctuations in other environmental conditions such as temperature given the structural differences between the enzymes (Yimga et al. 2003; Op den Camp et al. 2009).
Following the initial oxidation of methane, methanol is typically oxidised by methanol dehydrogenases containing the co-factor pyrroloquinoline quinone (Fig. 2) to formaldehyde (MxaF) or directly into formate (XoxF) (Keltjens et al. 2014). In the proteobacterial methanotrophs, formaldehyde is then catabolically oxidised via a variety of pathways to carbon dioxide (Chistoserdova 2011) or assimilated into biomass via either the ribulose monophosphate or serine cycle pathways (Chistoserdova and Lidstrom 2013). The conversion of methanol to formaldehyde, as catalysed by methanol dehydrogenase, has been thoroughly studied in methylotrophs and does not appear to be thermally limiting. Although there are no reports of thermophilic obligate methylotrophs, there are many facultative methanol-oxidising, aerobic bacteria capable of methylotrophic growth at elevated temperatures (e.g. Thermoflavifilum aggregans (35–63°C) (Anders et al. 2014), Thermorudis pharmacophila (53–76°C) (Houghton et al. 2015) and Vulcanithermus mediatlanticus (37–80°C) (Miroshnichenko et al. 2003)). This diversity of thermophilic methylotrophs suggests that some methanol dehydrogenases, and the subsequent enzymes within carbon dissimilation pathways, have evolved the capacity for thermophilic stability. Indeed, NAD-dependent methanol dehydrogenases, such as those found in Bacillus methanolicus, actually become more active at elevated temperatures (Whitaker et al. 2015).
THERMAL ADAPTATION IN METHANOTROPHIC METABOLISMS
Thermotolerant or thermophilic methanotrophs must, like all organisms living at elevated temperatures, have other thermal adaptations. Both thermophilic Methylocaldum szegediense and thermotolerant M. capsulatus display increased activity of cytochrome c peroxidase during growth at elevated temperatures. It is likely this adaption occurs in order to cope with the increased concentration of reactive oxygen species produced (Medvedkova et al. 2009). Similarly, formate dehydrogenase, hexulose phosphate synthase and hydroxypyruvate reductase from M. szegediense, which are all involved in carbon assimilation using either the serine cycle or ribulose monophosphate pathways, have been shown to have higher temperature optima than those from M. capsulatus or mesophilic Methylocystis echinoides (Medvedkova, Khmelenina and Trotsenko 2007).
Thermophilic bacteria and archaea have been shown to accumulate compatible solutes or thermolytes, such as polyamines, sucrose, α-glutamate, di-myo-inositol phosphate or hydroxyectoine, in response to both osmotic and heat stress (Oshima 2007; d'Avó et al. 2013; Esteves et al. 2014; Salvador et al. 2015). In a similar way, M. szegediense is thought to use sucrose as a possible thermoprotectant, accumulating the sugar in response to increased growth temperature (Medvedkova, Khmelenina and Trotsenko 2007).
Long-chain fatty acids are frequently found in the lipid membranes of thermophiles, as longer length chains typically have higher melting points (Pond and Langworthy 1987), which results in greater membrane stability at elevated temperatures. Conversely, the cold-adapted methanotroph Methylovulum psychrotolerans has recently been demonstrated to synthesise more short-chain and unsaturated fatty acids as growth temperatures decrease from 20 to 4°C (Bale et al. 2019). However, high-temperature methanotrophs do not always appear to follow this rule. For example, Methylacidiphilum kamchatkense produces higher quantities of ai15:0 and i14:0 than 18:0 fatty acids (Op den Camp et al. 2009), while Methylocaldum strains O-12 and H-11 synthesise more 16:0 and 16:1 than cy17:0 fatty acids (Eshinimaev et al. 2004). Many other thermophiles produce high proportions of branched fatty acids (Langworthy and Pond 1986; Koga 2012) despite branched fatty acids reportedly having lower melting points than their straight chain equivalents (Pond and Langworthy 1987), which suggests active regulation in response to temperature fluctuations may occur in all these strains (Weerkamp and Heinen 1972; Rilfors, Wieslander and Ståhl 1978).
Given the diversity of thermal adaptations already described within isolated methanotrophs, it is conceivable that other novel methane-oxidising bacteria may be found in high-temperature environments, possibly utilising other thermal adaptations not listed here.
THERMOPHILIC METHANOTROPHS AND BIOTECHNOLOGY
Methanotrophs have immense potential for environmental bioengineering. Excellent reviews of possible applications of methanotrophs in biotechnology were recently carried out by Strong and colleagues (Strong, Xie and Clarke 2015; Strong et al. 2016), including production of biopolymers such as poly-β-hydroxybutyrate (Shah, Hanna and Taylor 1996; Khmelenina et al. 2015), carotenoids (Ye et al. 2007) and biofuels (Silverman, Resnick and Mendez 2014). Since as early as 1999, M. capsulatus Bath, a thermotolerant methanotroph, has been used for the production of a single cell protein animal feed and other products on an industrial scale by a number of biotechnology companies (Aas et al. 2006; Øverland et al. 2010). Outside M. capsulatus, there are no reported applications of thermophilic methanotrophs being specifically used in biotechnology. This may be due to the lower methane and oxygen solubilities at elevated temperatures conferring no rate advantage over mesophilic methanotroph counterparts. Nevertheless, the application of thermophilic methanotrophs such as the acidophilic Methylacidiphilum spp. to methane bioconversion, while not displaying improved reaction or solubilities due to increased temperatures, may offer some advantages of more relaxed bioreactor operating conditions due to reduced contamination of methanotrophic cultures.
Other potential applications for thermophilic methanotrophs could be as biocovers or biofilters in factory farms and landfill sites that can remove up to 85% and 89–100% of produced CH4, respectively (Melse and Van Der Werf 2005; Scheutz et al. 2009). The non-specific nature of both methane monooxygenases also highlights a potential use for methanotrophs in bioremediation of non-methane contaminants. The sMMO, in particular, has a wide range of substrates that include alkanes, alkenes, aromatics and halogenated aliphatics that are oxidised but with no apparent energetic benefit to the bacterium (Colby, Stirling and Dalton 1977; Fox et al. 1990). Methanotrophs have also been appliedin situ to remove contaminants including pesticides from groundwater (Hedegaard et al. 2018) and chlorinated solvents such as trichloroethylene in soil (Sutfin and Ramey 1997; Hazen et al. 2009). It may also be possible to use methanotrophs to harvest or recover rare earth elements, essential for technology components including batteries and computers, which are currently extracted at high cost and low efficiency. The ability of methylotrophs to solubilise and transport lanthanides, for example, required for XoxF-type methanol dehydrogenase activity, has already been exploited to biorecycle electrical waste components (Martinez-Gomez, Vu and Skovran 2016) and could be used to obtain lanthanides from mining ores. While none of the current biotechnological applications currently utilise thermophilic methanotrophs, further applied research on the current strains and/or the discovery of novel thermophilic strains in the future could offer new options for converting industrial wastes and gases into useful, high-value products.
CONCLUSION
The diversity of known methanotrophs has expanded greatly over the last decade, but our knowledge of thermophilic methanotrophy is sparse compared to our understanding of the ecology and function of mesophilic representatives. The recent isolation and characterisation of several thermophilic bacterial species and the significant advances in understanding the verrucomicrobial thermophilic methanotrophs highlight the need for further focussed research in this area. Of particular note, recent publications on the role of mixotrophy in methanotrophic respiration strategies highlight the potential for other substrates to participate in the growth and/or persistence of methanotrophs at elevated temperatures. Geothermal and hydrothermal fields, which release large volumes of methane, are excellent targets for attempts to investigate and cultivate novel thermophilic methanotrophs. Many culture-independent studies investigating specific functional genes, metagenomic, metatranscriptomic and metaproteomic data, and incorporation of radiolabelled 13CH4 or 13CO2, have shown the potential of these high-temperature environments to host hitherto unidentified strains capable of methane oxidation at higher temperatures. This may also lead to insights into how methanotrophs mediate biogeochemical cycling of minor elements such as copper and lanthanides in these environments. Copper (required for methane monooxygenase activity) can be found in elevated concentrations in geothermal surface waters compared to freshwater, particularly in low-pH systems (Kaasalainen and Stefánsson 2012), while rare earth elements have been observed in high concentrations in magmatic and metamorphic rocks that are often found in geothermal areas (Tyler 2004).
In conclusion, we note that the field of thermophilic methanotrophy is relatively immature, particularly with respect to the currently fast-moving field of anaerobic methane oxidation, and will benefit from further focused studies of geothermal and heated environments, and via the mining of expanding metagenomic datasets and comparative genomics. Our current understanding of methanotrophy does not preclude the discovery of additional thermophilic methanotrophs and novel methanotrophic pathways. Indeed, we anticipate a continued expansion in knowledge in this field, particularly as the impacts of climate change and greenhouse gas emissions become more pressing.
FUNDING
This work was supported by the GNS Science New Zealand's Geothermal Future research programme, the Royal Society of New Zealand (Marsden Grant GNS1601 to CRC) and a Freemasons New Zealand Postgraduate Scholarship to KMH.
Conflict of interest. None declared.