-
PDF
- Split View
-
Views
-
Cite
Cite
Kiran Preet Padda, Akshit Puri, Chris Chanway, Endophytic nitrogen fixation – a possible ‘hidden’ source of nitrogen for lodgepole pine trees growing at unreclaimed gravel mining sites, FEMS Microbiology Ecology, Volume 95, Issue 11, November 2019, fiz172, https://doi.org/10.1093/femsec/fiz172
- Share Icon Share
ABSTRACT
Lodgepole pine (Pinus contorta var. latifolia) trees have been thriving on unreclaimed gravel mining sites in British Columbia, Canada, with tissue nitrogen-content and growth-rate unaffected by extremely low soil nitrogen-levels. This indicates that pine trees could be accessing a hidden nitrogen source to fulfill their nitrogen requirements – possibly via endophytic nitrogen-fixation. Endophytic bacteria originally isolated from native pine trees growing at gravel sites were selected (n = 14) for in vitro nitrogen-fixation assays and a year long greenhouse study to test the overall hypothesis that naturally occurring endophytic nitrogen-fixing bacteria sustain pine tree growth under nitrogen-limited conditions. Each of the 14 bacteria colonized the internal tissues of pine trees in the greenhouse study and fixed significant amounts of nitrogen from atmosphere (23%–53%) after one year as estimated through 15N isotope dilution assay. Bacterial inoculation also significantly enhanced the length (31%–64%) and biomass (100%–311%) of pine seedlings as compared to the non-inoculated control treatment. In addition, presence of the nifH gene was confirmed in all 14 bacteria. Our results support the possibility that pine trees associate with nitrogen-fixing bacteria, capable of endophytic colonization, to survive at unreclaimed gravel mining pits and this association could potentially be utilized for effective reclamation of highly disturbed sites in a sustainable manner.
INTRODUCTION
Lodgepole pine (Pinus contorta var. latifolia) is a highly adaptable gymnosperm species that can thrive in extremely nitrogen-limited environments, from bare gravel substrates to dry sandy soils (Chapman and Paul 2012; Anand, Grayston and Chanway 2013). Due to the ability of lodgepole pine trees to grow in nitrogen-poor conditions, their tissue nitrogen-content is often poorly linked to the soil nitrogen-levels (Kranabetter et al.2006). Long-standing sub-boreal and boreal forests (particularly, pine stands) are reported to accumulate more nitrogen in vegetation than can be explained by known sources of nitrogen, thus limiting our ability to understand and predict the nitrogen inputs for these forests (Moyes et al.2016). Mineralization of organic nitrogen by free-living soil microorganisms has long been considered the primary process by which plant-available nitrogen is released into the soil but this process seems inadequate for the release of sufficient nitrogen at pine stands with little to no organic matter or where low temperatures limit soil nitrogen turnover (Weetman, Fournier and Schnorbus 1988). Although nodulating actinorhizal plants are common in early succession forests under humid and cool environments, they are typically absent in old-growth boreal forests (Walker 1993; Vitousek et al.2002), suggesting that these nitrogen-poor ecosystems could be relying on some hidden nitrogen source.
Endophytic diazotrophs, nitrogen-fixing bacteria living asymptomatically within plant tissues, could offer a unique nitrogen-input pathway for their host and the ecosystem (Knoth et al.2014). Several angiosperm and gymnosperm species, especially those growing in low-nutrient environments, have consequently evolved special strategies to deal with nitrogen limitation, possibly via endophytic nitrogen-fixation (Reinhold-Hurek and Hurek 2011; Yang et al.2016, 2017). Biological nitrogen fixation (BNF) by endophytic bacteria has been studied widely in various crop species, including sugarcane (Saccharum officinarum L.) (Boddey et al.1991), corn (Zea mays L.) (Puri, Padda and Chanway 2015) and canola (Brassica napus L.) (Padda, Puri and Chanway 2016, 2017). However, very few studies have reported nitrogen fixation by endophytic bacteria in deciduous and coniferous tree species (Doty et al.2009; Carrell and Frank 2014). In a recent study, endophytic diazotrophs were estimated to provide 6.8–13.6 μg N m−2 d−1 to limber pine (Pinus flexis) trees (Moyes et al.2016). Significant nitrogenase (enzyme responsible for BNF) activity has also been demonstrated by endophytic bacteria of lodgepole pine and hybrid white spruce (Picea glauca x engelmannii) trees (Puri, Padda and Chanway 2018, 2019). In addition to BNF, endophytic diazotrophs have also been reported to help Douglas-fir (Pseudotsuga menziesii) and western red cedar (Thuja plicata) survive under extreme drought and degraded edaphic conditions (Aghai et al.2019).
Chapman and Paul (2012) provided compelling evidence that lodgepole pine trees growing on severely nitrogen-poor gravel could be accessing biologically fixed nitrogen in conjugation with certain symbionts. Gravel mining pits located in the Sub-Boreal Pine-Spruce dry-cool (SBPSdc) biogeoclimatic zone of British Columbia, Canada represent one of the most nitrogen-limited and highly disturbed environments, due to the combination of gravelly parent material, lack of topsoil and vegetation and low precipitation-borne nitrogen inputs (Chapman and Paul 2012). In fact, the soil nitrogen levels at these pits were found to be six-fold lower than nearby undisturbed forest sites with intact topsoil. Yet, lodgepole pine trees have been reported to grow well at these gravel pits. What's more puzzling is the fact that the foliar nitrogen-content and growth rate of pine trees growing at pits was comparable to pine trees growing on undisturbed soils in a nearby forest, despite such large differences in soil nitrogen levels (Chapman and Paul 2012). In addition, the δ15N content in tissues of pine trees at gravel pits was significantly lower than pine trees growing on undisturbed soils (Chapman and Paul 2012), suggesting that pit trees have access to an unknown nitrogen source, possibly associative or symbiotic nitrogen-fixation. This is consistent with the theory that the δ15N levels are lower in plants when they can acquire nitrogen in the form of 14N through BNF, thereby resulting in dilution of their 15N levels (Högberg 1997; Unkovich 2013).
Previously, we sampled young lodgepole pine trees (< 5 years old and < 30 cm height) from two of the six gravel mining pits reported by Chapman and Paul (2012), namely, Anah pit (52°09’42.7’ N, 123°10’24.3’ W, 1132 m a.s.l.) and Skulow pit (52°18’54.1’ N, 121°53’39.3’ W, 1064 m a.s.l.), with the objective to determine if lodgepole pine trees naturally harbor nitrogen-fixing endophytes in their tissues (Padda, Puri and Chanway 2018). Seventy-seven potential endophytic diazotrophs were isolated from needle, stem and root tissues of pine trees. Of these, 32 endophytes were tested positive for nitrogenase activity using the acetylene reduction assay (ARA) (Padda, Puri and Chanway 2018). Since in planta inoculation experiments have demonstrated that endophytes can influence principal ecological traits of the plant phenotype, in this study, we explored the potential of our endophytic isolates to fix nitrogen and stimulate lodgepole pine growth under nitrogen-limited conditions in a greenhouse study. The specific hypotheses tested in this study were: (i) native endophytic bacteria colonize and survive in biologically significant numbers inside lodgepole pine tissues following re-inoculation; (ii) these bacteria fix nitrogen from the atmosphere and transfer it to their host and (iii) inoculation with these bacteria significantly enhances the growth rate of pine seedlings.
MATERIALS AND METHODS
Selection of endophytic bacteria
Endophytic diazotrophic strains (n = 32) whose nitrogenase activity was identified and reported previously by Padda, Puri and Chanway (2018) were used in this study. Antibiotic-resistant derivatives of these bacterial strains were raised by growing each strain on combined carbon medium (CCM) (Rennie 1981) amended with an antibiotic agent (200 mg l−1 of rifamycin) to facilitate the evaluation of endophytic bacterial colonization in the greenhouse study (Bal and Chanway 2012a). The nitrogenase activity of antibiotic-resistant strains was re-confirmed using ARA following the procedure outlined in Bal et al. (2012) and Padda, Puri and Chanway (2018). Briefly, for this assay, bacterial strains were grown in 22 ml crimp-top vials filled with 9 ml CCM broth amended with 200 mg l−1 rifamycin and incubated at room temperature with shaking until the broth appeared turbid (OD600 = 0.5). Three replicate vials were used per bacterial strain. Acetylene gas was injected into each vial to a final volume of 10% of headspace (v/v). Correspondingly, triplicate vials of three types of controls were used: CCM broth inoculated with bacteria without acetylene; non-inoculated CCM broth with 10% acetylene; and non-inoculated CCM broth without acetylene. After 48 h, a 1 ml sample of gas was removed from each vial and the ethylene content was measured using flame ionization gas chromatography. The amount of acetylene converted to ethylene by each strain was obtained by subtracting the ethylene content detected for the three controls from the ethylene content detected for the strain. The whole nitrogenase assay was repeated to check for consistency of the acetylene reduction activity of strains. Consequently, 14 endophytic bacterial strains that showed consistently positive results in ARA were selected for nifH gene analysis and the greenhouse study (Table 1). Bacterial strains were stored at −80°C in CCM containing 200 mg l−1 rifamycin and amended with 20% (v/v) glycerol.
List of endophytic bacterial strains used in this study originally isolated from lodgepole pine (Pinus contorta) trees growing at two unreclaimed mining pits in the central interior of British Columbia (Padda, Puri and Chanway 2018).
Bacterial strains . | Antibiotic-resistant derivative . | Origin . | |
---|---|---|---|
. | . | Gravel site . | Tissue . |
Pseudomonas mandelii AS1 | AS1r | Anah pit | Stem |
Pseudomonas graminis AN1 | AN1r | Anah pit | Needle |
Rathayibacter tanaceti AN2 | AN2r | Anah pit | Needle |
Frigoribacterium endophyticum AN3 | AN3r | Anah pit | Needle |
Pseudomonas rhizosphaerae AN4 | AN4r | Anah pit | Needle |
Pseudomonas frederiksbergensis AN5 | AN5r | Anah pit | Needle |
Pseudomonas migulae AR1 | AR1r | Anah pit | Root |
Pseudomonas mandelii SS1 | SS1r | Skulow pit | Stem |
Pseudomonas frederiksbergensis SS2 | SS2r | Skulow pit | Stem |
Herbiconiux solani SS3 | SS3r | Skulow pit | Stem |
Pseudomonas lini SN1 | SN1r | Skulow pit | Needle |
Flavobacterium aquidurense SN2 | SN2r | Skulow pit | Needle |
Caballeronia sordidicola SR1 | SR1r | Skulow pit | Root |
Rhizobium herbae SR2 | SR2r | Skulow pit | Root |
Bacterial strains . | Antibiotic-resistant derivative . | Origin . | |
---|---|---|---|
. | . | Gravel site . | Tissue . |
Pseudomonas mandelii AS1 | AS1r | Anah pit | Stem |
Pseudomonas graminis AN1 | AN1r | Anah pit | Needle |
Rathayibacter tanaceti AN2 | AN2r | Anah pit | Needle |
Frigoribacterium endophyticum AN3 | AN3r | Anah pit | Needle |
Pseudomonas rhizosphaerae AN4 | AN4r | Anah pit | Needle |
Pseudomonas frederiksbergensis AN5 | AN5r | Anah pit | Needle |
Pseudomonas migulae AR1 | AR1r | Anah pit | Root |
Pseudomonas mandelii SS1 | SS1r | Skulow pit | Stem |
Pseudomonas frederiksbergensis SS2 | SS2r | Skulow pit | Stem |
Herbiconiux solani SS3 | SS3r | Skulow pit | Stem |
Pseudomonas lini SN1 | SN1r | Skulow pit | Needle |
Flavobacterium aquidurense SN2 | SN2r | Skulow pit | Needle |
Caballeronia sordidicola SR1 | SR1r | Skulow pit | Root |
Rhizobium herbae SR2 | SR2r | Skulow pit | Root |
List of endophytic bacterial strains used in this study originally isolated from lodgepole pine (Pinus contorta) trees growing at two unreclaimed mining pits in the central interior of British Columbia (Padda, Puri and Chanway 2018).
Bacterial strains . | Antibiotic-resistant derivative . | Origin . | |
---|---|---|---|
. | . | Gravel site . | Tissue . |
Pseudomonas mandelii AS1 | AS1r | Anah pit | Stem |
Pseudomonas graminis AN1 | AN1r | Anah pit | Needle |
Rathayibacter tanaceti AN2 | AN2r | Anah pit | Needle |
Frigoribacterium endophyticum AN3 | AN3r | Anah pit | Needle |
Pseudomonas rhizosphaerae AN4 | AN4r | Anah pit | Needle |
Pseudomonas frederiksbergensis AN5 | AN5r | Anah pit | Needle |
Pseudomonas migulae AR1 | AR1r | Anah pit | Root |
Pseudomonas mandelii SS1 | SS1r | Skulow pit | Stem |
Pseudomonas frederiksbergensis SS2 | SS2r | Skulow pit | Stem |
Herbiconiux solani SS3 | SS3r | Skulow pit | Stem |
Pseudomonas lini SN1 | SN1r | Skulow pit | Needle |
Flavobacterium aquidurense SN2 | SN2r | Skulow pit | Needle |
Caballeronia sordidicola SR1 | SR1r | Skulow pit | Root |
Rhizobium herbae SR2 | SR2r | Skulow pit | Root |
Bacterial strains . | Antibiotic-resistant derivative . | Origin . | |
---|---|---|---|
. | . | Gravel site . | Tissue . |
Pseudomonas mandelii AS1 | AS1r | Anah pit | Stem |
Pseudomonas graminis AN1 | AN1r | Anah pit | Needle |
Rathayibacter tanaceti AN2 | AN2r | Anah pit | Needle |
Frigoribacterium endophyticum AN3 | AN3r | Anah pit | Needle |
Pseudomonas rhizosphaerae AN4 | AN4r | Anah pit | Needle |
Pseudomonas frederiksbergensis AN5 | AN5r | Anah pit | Needle |
Pseudomonas migulae AR1 | AR1r | Anah pit | Root |
Pseudomonas mandelii SS1 | SS1r | Skulow pit | Stem |
Pseudomonas frederiksbergensis SS2 | SS2r | Skulow pit | Stem |
Herbiconiux solani SS3 | SS3r | Skulow pit | Stem |
Pseudomonas lini SN1 | SN1r | Skulow pit | Needle |
Flavobacterium aquidurense SN2 | SN2r | Skulow pit | Needle |
Caballeronia sordidicola SR1 | SR1r | Skulow pit | Root |
Rhizobium herbae SR2 | SR2r | Skulow pit | Root |
DNA extraction and nifH gene analysis
Fourteen selected bacterial strains were tested for the presence of the nifH gene to further examine their nitrogenase activity. Frozen strains were thawed and streaked onto CCM agar amended with rifamycin and then grown in CCM broth amended with rifamycin on a rotary shaker for 2 d at 30°C. Bacterial cells were harvested by centrifugation and the genomic DNA of each strain was extracted by using the DNeasy UltraClean Microbial Kit (Qiagen Inc., Valencia, CA, USA) following the steps outlined by the manufacturer. The concentration of the isolated DNA was evaluated by using a NanoDrop 2000c spectrophotometer (Thermo Scientific, Wilmington, DE, USA) and the quality of the DNA was confirmed by electrophoresis on a 0.8% agarose gel.
The nifH gene of each isolate was amplified using either of the following nifH primer sets: nifH1 [5′-TGYGAYCCNAARGCNGA-3′] and nifH2 [5′-ADNGCCATCATYTCNCC-3′] (Zehr and McReynolds 1989); IGK3 [5′-GCIWTHTAYGGIAARGGIGGIATHGGIAA-3′] and DVV [5′-ATIGCRAAICCICCRCAIACIACRTC-3′] (Ando et al.2005; Gaby and Buckley 2012); nifH19F [5′-GCIWTITAYGGNAARGGNGG-3′] and nifH-univ463R [5′- GCRTAIABNGCCATCATYTC-3′] (Bürgmann et al.2004; Gaby and Buckley 2012) (Table 2). PCR amplification was performed in 25 μl reaction volumes containing 2.5 μl of 1x PCR buffer (without MgCl2), 1.25 μl of MgCl2 (2.5 mM), 0.5 μl of Taq DNA Polymerase (5 U/μl), 0.5 μl of dNTP mix (consisting of four nucleotides: dATP, dCTP, dGTP, dTTP), 2.5 μl of each primer (1 μM), and 2.5 μl of template DNA (1 ng/μl) (Gaby and Buckley 2012; Paul, Chapman and Chanway 2013). The final volume was adjusted to 25 μl with nuclease-free water. Amplifications were performed using the MJ Mini gradient thermal cycler (Bio-Rad Laboratories Inc., Hercules, CA, USA) with the following program: initial cell lysis and denaturation for 5 min at 95°C; followed by 35 cycles of denaturation (1 min at 94°C), annealing (1 min at 55°C) and extension (1 min at 72°C) and a final extension for 10 min at 72°C. Amplification of PCR products was confirmed by electrophoresis on 1% agarose gel for 1 h at 100 volts.
Amount of ethylene produced by each endophytic bacterial strain in acetylene reduction assay (ARA) and PCR amplification of the nifH gene indicated by ‘+’.
Bacterial strains . | ARA (nmol C2H4 ml−1)a . | nifH gene . | |
---|---|---|---|
. | . | Presence of nifH . | Primer Set . |
Pseudomonas mandelii AS1r | 1.5 ± 0.21b | + | nifH1/nifH2 |
Pseudomonas graminis AN1r | 2.0 ± 0.13 | + | nifH1/nifH2 |
Rathayibacter tanaceti AN2r | 1.7 ± 0.44 | + | nifH1/nifH2 |
Frigoribacterium endophyticum AN3r | 0.7 ± 0.09 | + | nifH1/nifH2 |
Pseudomonas rhizosphaerae AN4r | 0.9 ± 0.11 | + | nifH1/nifH2 |
Pseudomonas frederiksbergensis AN5r | 1.0 ± 0.34 | + | IGK3/DVV |
Pseudomonas migulae AR1r | 2.3 ± 0.41 | + | nifH1/nifH2 |
Pseudomonas mandelii SS1r | 1.7 ± 0.53 | + | nifH1/nifH2 |
Pseudomonas frederiksbergensis SS2r | 1.4 ± 0.27 | + | IGK3/DVV |
Herbiconiux solani SS3r | 0.8 ± 0.10 | + | nifH19F/nifH- univ463R |
Pseudomonas lini SN1r | 1.8 ± 0.20 | + | nifH1/nifH2 |
Flavobacterium aquidurense SN2r | 2.1 ± 0.42 | + | nifH1/nifH2 |
Caballeronia sordidicola SR1r | 1.5 ± 0.34 | + | nifH1/nifH2 |
Rhizobium herbae SR2r | 1.0 ± 0.11 | + | nifH1/nifH2 |
Bacterial strains . | ARA (nmol C2H4 ml−1)a . | nifH gene . | |
---|---|---|---|
. | . | Presence of nifH . | Primer Set . |
Pseudomonas mandelii AS1r | 1.5 ± 0.21b | + | nifH1/nifH2 |
Pseudomonas graminis AN1r | 2.0 ± 0.13 | + | nifH1/nifH2 |
Rathayibacter tanaceti AN2r | 1.7 ± 0.44 | + | nifH1/nifH2 |
Frigoribacterium endophyticum AN3r | 0.7 ± 0.09 | + | nifH1/nifH2 |
Pseudomonas rhizosphaerae AN4r | 0.9 ± 0.11 | + | nifH1/nifH2 |
Pseudomonas frederiksbergensis AN5r | 1.0 ± 0.34 | + | IGK3/DVV |
Pseudomonas migulae AR1r | 2.3 ± 0.41 | + | nifH1/nifH2 |
Pseudomonas mandelii SS1r | 1.7 ± 0.53 | + | nifH1/nifH2 |
Pseudomonas frederiksbergensis SS2r | 1.4 ± 0.27 | + | IGK3/DVV |
Herbiconiux solani SS3r | 0.8 ± 0.10 | + | nifH19F/nifH- univ463R |
Pseudomonas lini SN1r | 1.8 ± 0.20 | + | nifH1/nifH2 |
Flavobacterium aquidurense SN2r | 2.1 ± 0.42 | + | nifH1/nifH2 |
Caballeronia sordidicola SR1r | 1.5 ± 0.34 | + | nifH1/nifH2 |
Rhizobium herbae SR2r | 1.0 ± 0.11 | + | nifH1/nifH2 |
nanomoles of ethylene produced per millilitre of culture vial headspace
Mean ± standard error; n = 3 per strain
Amount of ethylene produced by each endophytic bacterial strain in acetylene reduction assay (ARA) and PCR amplification of the nifH gene indicated by ‘+’.
Bacterial strains . | ARA (nmol C2H4 ml−1)a . | nifH gene . | |
---|---|---|---|
. | . | Presence of nifH . | Primer Set . |
Pseudomonas mandelii AS1r | 1.5 ± 0.21b | + | nifH1/nifH2 |
Pseudomonas graminis AN1r | 2.0 ± 0.13 | + | nifH1/nifH2 |
Rathayibacter tanaceti AN2r | 1.7 ± 0.44 | + | nifH1/nifH2 |
Frigoribacterium endophyticum AN3r | 0.7 ± 0.09 | + | nifH1/nifH2 |
Pseudomonas rhizosphaerae AN4r | 0.9 ± 0.11 | + | nifH1/nifH2 |
Pseudomonas frederiksbergensis AN5r | 1.0 ± 0.34 | + | IGK3/DVV |
Pseudomonas migulae AR1r | 2.3 ± 0.41 | + | nifH1/nifH2 |
Pseudomonas mandelii SS1r | 1.7 ± 0.53 | + | nifH1/nifH2 |
Pseudomonas frederiksbergensis SS2r | 1.4 ± 0.27 | + | IGK3/DVV |
Herbiconiux solani SS3r | 0.8 ± 0.10 | + | nifH19F/nifH- univ463R |
Pseudomonas lini SN1r | 1.8 ± 0.20 | + | nifH1/nifH2 |
Flavobacterium aquidurense SN2r | 2.1 ± 0.42 | + | nifH1/nifH2 |
Caballeronia sordidicola SR1r | 1.5 ± 0.34 | + | nifH1/nifH2 |
Rhizobium herbae SR2r | 1.0 ± 0.11 | + | nifH1/nifH2 |
Bacterial strains . | ARA (nmol C2H4 ml−1)a . | nifH gene . | |
---|---|---|---|
. | . | Presence of nifH . | Primer Set . |
Pseudomonas mandelii AS1r | 1.5 ± 0.21b | + | nifH1/nifH2 |
Pseudomonas graminis AN1r | 2.0 ± 0.13 | + | nifH1/nifH2 |
Rathayibacter tanaceti AN2r | 1.7 ± 0.44 | + | nifH1/nifH2 |
Frigoribacterium endophyticum AN3r | 0.7 ± 0.09 | + | nifH1/nifH2 |
Pseudomonas rhizosphaerae AN4r | 0.9 ± 0.11 | + | nifH1/nifH2 |
Pseudomonas frederiksbergensis AN5r | 1.0 ± 0.34 | + | IGK3/DVV |
Pseudomonas migulae AR1r | 2.3 ± 0.41 | + | nifH1/nifH2 |
Pseudomonas mandelii SS1r | 1.7 ± 0.53 | + | nifH1/nifH2 |
Pseudomonas frederiksbergensis SS2r | 1.4 ± 0.27 | + | IGK3/DVV |
Herbiconiux solani SS3r | 0.8 ± 0.10 | + | nifH19F/nifH- univ463R |
Pseudomonas lini SN1r | 1.8 ± 0.20 | + | nifH1/nifH2 |
Flavobacterium aquidurense SN2r | 2.1 ± 0.42 | + | nifH1/nifH2 |
Caballeronia sordidicola SR1r | 1.5 ± 0.34 | + | nifH1/nifH2 |
Rhizobium herbae SR2r | 1.0 ± 0.11 | + | nifH1/nifH2 |
nanomoles of ethylene produced per millilitre of culture vial headspace
Mean ± standard error; n = 3 per strain
Greenhouse study
A yearlong greenhouse study was set-up to evaluate the ability of selected bacteria to endophytically colonize lodgepole pine seedlings and to quantify the amount of nitrogen fixed and seedling growth promoted by each bacterium. Lodgepole pine seeds were inoculated with each of the 14 selected bacterial strains. Non-inoculated seeds were used as a control. This resulted in 15 treatments: 14 bacteria-inoculated and 1 control, each replicated 65 times.
Pre-treatment of seeds
For the greenhouse study, lodgepole pine seeds were obtained from the British Columbia Ministry of Forests Tree Seed Center, Surrey, BC, Canada and originated from sites located near Williams Lake, BC (52° 27′ N latitude, 123° 20′ W longitude, elevation 1188 m, SBPSdc zone). Seeds were surface-sterilized by immersion in 30% hydrogen peroxide for 90 s, followed by three 30 s rinses in sterile distilled water. The effectiveness of the surface sterilization was confirmed by imprinting sterilized seed on tryptic soy agar (TSA) and then checking for microbial contamination 48 h later. Seeds found to be free of surface contamination were placed in sterile cheesecloth bags containing moist autoclaved sand and stratified for 28 d at 4°C. Stratified seeds were again imprinted on TSA plates for 48 h to confirm the absence of surface contamination. About 10 randomly selected surface-sterilized stratified seeds were crushed and imprinted on TSA plates amended with 200 mg l−1 rifamycin for 48 h to confirm the absence of internal seed contamination by any of the selected bacterial strains prior to inoculation.
Inoculum preparation and seedling growth assay
Bacterial inoculum was prepared by thawing frozen cultures of each of the 14 selected bacterial strains and streaking a loopful onto CCM agar amended with rifamycin (200 mg l−1) and incubating at 30°C for 48 h. After the colonies grew, a loopful of each strain was inoculated into 1 l flasks containing 500 ml of fresh CCM broth amended with rifamycin (200 mg l−1). Flasks were secured on a rotary shaker and agitated for 48 h at room temperature. Bacterial cells were harvested by centrifugation (3000 x g, 30 min), washed twice with sterile phosphate buffer saline (PBS) and re-suspended in the same buffer to a density of 106 colony forming units (cfu) per ml.
Lodgepole pine seedlings were grown in Ray Leach Cone-tainers (height, 210mm; diameter, 38mm) filled to 67% capacity with an autoclaved sand–Turface mixture (69% w/w silica sand; 29% w/w Turface; 2% w/w CaCO3). Each Cone-tainer was fertilized with 20 ml of a nutrient solution composed of Ca(15NO3)2 (5% 15N label), 0.0576 g l−1; Na2FeEDTA, 0.02 g l−1; KH2PO4, 0.14 g l−1; MgSO4, 0.49 g l−1; H3BO3, 0.001 g l−1; MnCI2.4H2O, 0.001 g l−1; ZnSO4.7H2O, 0.001 g l−1; CuSO4.5H2O, 0.0001 g l−1 and NaMoO4.2H2O, 0.001 g l−1 (Padda, Puri and Chanway 2017). After adding the nutrient solution to each Cone-tainer, the sand–Turface mixture was thoroughly mixed to spatially homogenize the nutrient solution. Three surface-sterilized pine seeds were aseptically sown in each Cone-tainer and covered with 5 mm of autoclaved silica sand after the application of sterilized nutrient solution. Immediately after sowing, 5 ml of bacterial suspension of each strain was pipetted directly over the seeds into Cone-tainers designated for each treatment. The non-inoculated control seeds received 5 ml of sterile PBS containing no bacteria. All cone-tainers were kept in 98 cell-trays (length, 610 mm; width, 305 mm; height, 172 mm) and placed in the University of British Columbia Plant Services Centre greenhouse under a 16 h photoperiod with an intensity of at least 300 µmol s−1 m−2. Seedlings were thinned to contain the single largest germinant per Cone-tainer once emergence was complete. Seedlings were watered as required with sterile distilled water and received modified nutrient solution without Ca(15NO3)2 once every month during the 1 yr growth trial. Tray positions were randomized weekly to reduce positional effects during the trial.
Evaluation of endophytic colonization
For evaluation of endophytic colonization, five randomly selected lodgepole pine seedlings of each treatment were harvested destructively 4, 8 and 12 months after inoculation. Seedlings were rinsed in a 2 l flask containing 1 l sterile distilled water for removal of loosely adhering growth media. Seedlings were surface-sterilized in 0.6% (w/v) sodium hypochlorite for 5 min, rinsed three times with sterile distilled water and imprinted on TSA plates for 24 h to check for surface contamination. Samples of root, stem and leaf tissues were triturated separately in 1 ml of sterile PBS using a sterilized mortar and pestle. Triturated tissue suspensions of control and bacteria-inoculated seedlings were diluted serially and 0.1 ml of each dilution was plated onto CCM supplemented with 100 mg l−1 cycloheximide (to suppress fungal growth) and 200 mg l−1 rifamycin (to inhibit the growth of other bacteria). The growth of 14 strains used in the greenhouse study was not inhibited by rifamycin since they had acquired antibiotic resistance. Plates were incubated at room temperature for 7 d and numbers of CFU per gram of fresh tissue weight were evaluated.
Seedling growth response
To measure seedling length and biomass, 10 randomly selected lodgepole pine seedlings from each treatment were harvested destructively 4, 8 and 12 months after inoculation. Seedlings were removed from Cone-tainers and loosely adhering growth-media particles were removed from roots by washing under running water before measuring length. Each seedling was then oven-dried at 65°C for 2 d to determine seedling biomass (dry weight).
Estimation of biological nitrogen fixation
Statistical analysis
To assess the treatment effects on the growth of seedlings, a completely randomized experimental design with 65 replicates per treatment was used. Analysis of variance was performed by using the statistical package SAS University Edition (SAS Institute Inc., Cary, NC, USA) to evaluate the effect of bacterial inoculation on seedling growth parameters (seedling length, seedling biomass, atom % 15N excess, foliar nitrogen content). F-tests and t-tests were performed to separate and compare the means. The confidence level α was set to 0.05 to determine the significance of the model and treatment effects.
RESULTS
Acetylene reduction activity & PCR amplification of nifH
Antibiotic-resistant derivatives of all 32 bacterial strains showed positive nitrogenase activity in the ARA. Of these, 14 bacterial strains (seven originating from Anah gravel pit and seven originating from Skulow gravel pit) that showed consistently positive results in duplicate assays were selected for further analyses. The mean amount of ethylene produced by the 14 selected bacterial strains ranged from 0.7 to 2.3 nmol ml−1 (Table 2). The nitrogenase activity of these strains was also tested through amplification of the nifH gene. PCR amplification of nifH showed that all bacterial strains contained the nifH gene (Table 2). Since this method requires appropriate nitrogenase specific primers, we used multiple primer sets to amplify the nifH gene of bacterial strains.
Greenhouse study
Endophytic colonization
Each bacterial strain successfully colonized lodgepole pine roots endophytically after 4, 8 and 12 months of inoculation with population densities ranging from 103 to 106 cfu g−1 fresh tissue weight (Fig. 1). Half of these bacterial strains (AS1r, AN1r, AN2r, AR1r, SN1r, SN2r and SR2r) colonized root tissues with high population densities (105–106 cfu g−1) throughout the seedling growth period. Nine bacterial strains (AS1r, AN1r, AN2r, AN3r, AR1r, SS1r, SN1r, SN2r and SR2r) colonized stem tissues of lodgepole pine with a population size of 103–105 cfu g−1 fresh tissue weight (Fig. 1). Strains AN1r, AN2r, AR1r, SN1r and SN2r consistently colonized the stem tissues with 105 cfu g−1 fresh tissue weight throughout the growth trial. At the 12-month harvest, endophytic bacterial population densities in roots and stems were highest for Pseudomonas migulae AR1r (∼9.5 × 105 cfu g−1), with a 4.6-fold increase in stem population during the growth trial. Of the 14 bacterial strains, four strains (AN1r, AN2r, SN1r and SN2r) were detected inside needle tissues at all three harvests with population sizes ranging from 102–103 cfu g−1 fresh tissue weight (Fig. 1). Needle population sizes for Pseudomonas graminis AN1r and Pseudomonas lini SN1r increased 8-fold and 10.5-fold, respectively, between 4- and 12-month harvests. Overall, after 12 months of inoculation, strains AN1r, AR1r and SN1r were the top colonizers of pine tissues with a population size of > 106 cfu g−1. No endophytes were detected in surface-sterilized tissue extracts of control plants.
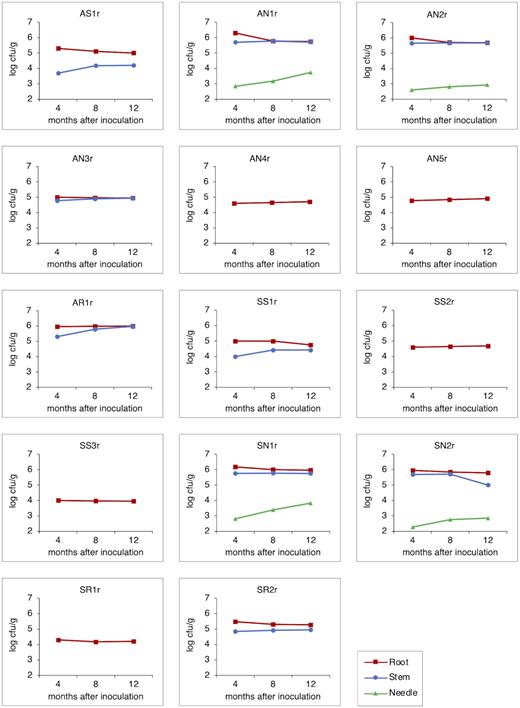
Mean endophytic population densities of bacterial strains expressed as log of colony forming units (CFU) per gram fresh weight of root, stem and needle tissue 4, 8 and 12 months after inoculation (n = 5 seedlings per treatment). For clarity of presentation, error bars were omitted.
Effect of inoculation on seedling growth
Bacteria-inoculated lodgepole pine seedlings demonstrated no signs of pathogenesis; rather all inoculation treatments exhibited a positive effect on seedling growth (Figs 2 and 3 and Fig. S1, Supporting Information). Bacterial inoculation considerably increased seedling length, with up to: 46% increase at the 4-month harvest, 40% increase at the 8-month harvest and 64% increase at the 12-month harvest. Four months after inoculation, all bacteria-inoculated seedlings, except SS1r, SS3r, SR1r and SR2r, were significantly longer than control seedlings (Fig. 2A). In particular, seedlings inoculated with strain AN3r and SN1r were 1.5-fold longer than control seedlings. Similarly, at the 8-month harvest, all but two (SS3r and SR1r) inoculation treatments had significantly higher seedling length as compared to control treatment (Fig. 2B). A significant increase (1.3–1.6-fold) in seedling lengths was observed for all bacteria-inoculated treatments in comparison to control after 12 months (Fig. 2C). Specifically, the AR1r-inoculated treatment outperformed eight other bacteria-inoculated treatments (AS1r, AN4r, AN5r, SS2r, SS3r, SN2r, SR1r and SR2r) in terms of seedling length. Additionally, AN1r, AN2r and SN1r inoculation, resulted in > 50% increase in the length of pine seedlings, performing significantly better than the SS3r and SR1r treatments.
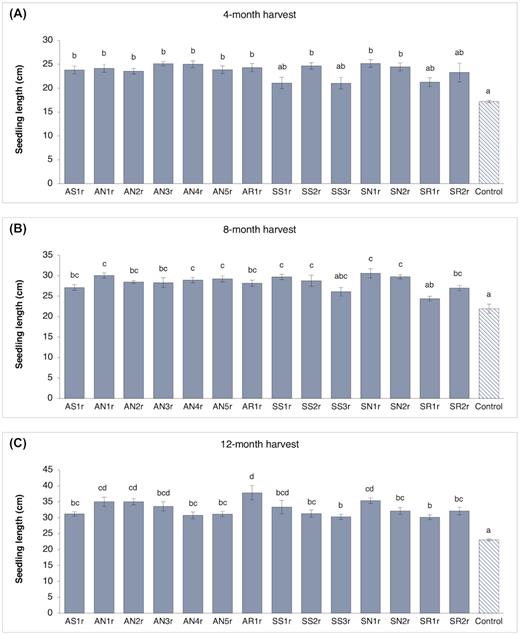
Seedling length (mean and standard error; n = 10 seedlings per treatment) of lodgepole pine (Pinus contorta) seedlings inoculated with different endophytic bacteria measured at (A) 4-month harvest, (B) 8-month harvest and (C) 12-month harvest. Bars with the different letter are significantly different (P < 0.05).
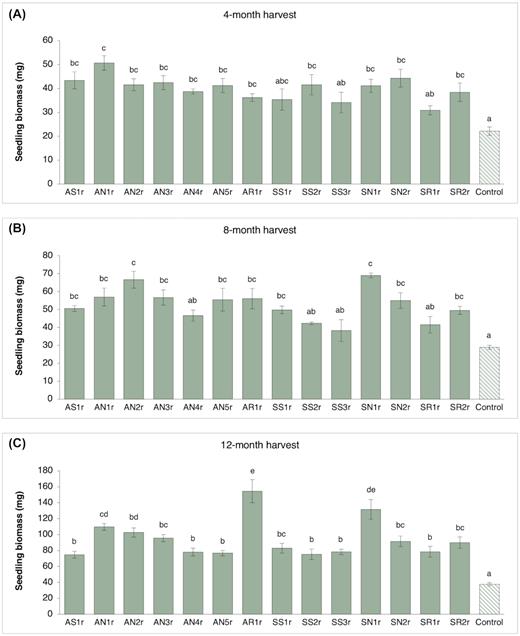
Seedling biomass (mean and standard error; n = 10 seedlings per treatment) of lodgepole pine (Pinus contorta) seedlings inoculated with different endophytic bacteria measured at (A), 4-month harvest, (B), 8-month harvest and (C), 12-month harvest. Bars with different letters are significantly different (P < 0.05).
Bacterial inoculation contributed to a significant increase in biomass (dry weight) of lodgepole pine seedlings over non-inoculated control plants throughout the greenhouse study (Figs. 3 and Fig. S1, Supporting Information). At the 4-month harvest, most bacteria-inoculated treatments had significantly greater seedling biomass than the control (Fig. 3A). AN1r and SN1r inoculation considerably enhanced seedling biomass (by > 100%) as compared to the control group. Additionally, AS1r, AN2r, AN3r, AN5r, SS2r and SN1r inoculation resulted in an increase of 80%–100% biomass while AN4r, AR1r and SR2r inoculation increased biomass by 60%–80%. Eight months after inoculation, half of the bacteria-inoculated treatments had nearly 2-fold higher biomass than the control treatment (Fig. 3B). AN2r and SN1r inoculation enhanced the biomass of pine seedlings by over 130%, performing considerably better than AN4r, SS2r, SS3r and SR1r inoculation treatments. All bacteria-inoculated treatments stimulated seedling biomass at the 12-month harvest, accumulating > 100% higher biomass than controls (Fig. 3C). Inoculation with AR1r, SN1r and AN1r strains led to a significant increase in biomass production – 311%, 250% and 200%, respectively. Notably, the biomass of AR1r-inoculated pine seedlings was considerably greater (1.5–2-fold) than all other bacteria-inoculated treatments (except SN1r). SN1r treatment also performed significantly better (> 1.5-fold higher biomass) than most other bacteria-inoculated treatments (Fig. 3C).
Estimation of biological nitrogen fixation
Foliar nitrogen content (mg per plant) of pine seedlings inoculated with bacteria was up to 3-fold and 10-fold higher than the control at the 6- and 12-month harvests, respectively, despite the fact that seedlings were grown under very limited soil nitrogen conditions (similar to gravel mining sites) in this experiment (Fig. 4). Six months after inoculation, a significant increase (> 2-fold) in foliar nitrogen content was observed for 11 bacteria-inoculated treatments (except SS2r, SS3r and SR1r) as compared to the control treatment (Fig. 4A). At the 12-month harvest, nine inoculation treatments had significantly higher foliar nitrogen content (> 3-fold) in comparison to the control (Fig. 4B). Notably, the foliar nitrogen content of seedlings inoculated with AR1r, SN1r and AN1r was 10-fold, 6.5-fold and 5-fold higher than control seedlings, respectively. In addition, the AR1r-inoculated seedlings performed significantly better than all other bacterial treatments by accumulating up to 4-fold more foliar nitrogen.
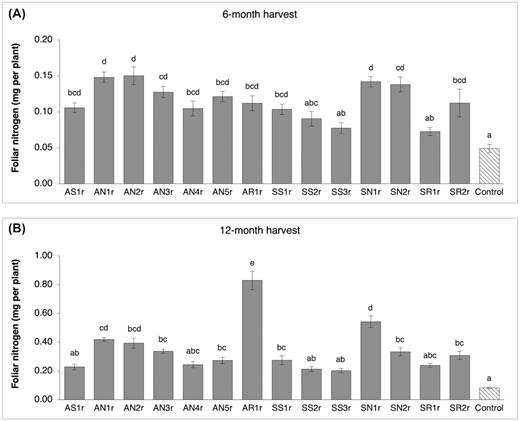
Foliar nitrogen content (mean and standard error; n = 10 seedlings per treatment) of lodgepole pine (Pinus contorta) seedlings harvested (A), 6 months after inoculation and (B), 12 months after inoculation. Bars with different letters are significantly different (P < 0.05).
In the 15N isotope dilution assay, the atom % 15N excess in the foliage was significantly lower in inoculated seedlings after 1 year, suggesting the occurrence of BNF (Table 3). Based on the atom % 15N excess values, pine seedlings inoculated with diazotrophic bacteria derived up to 17% of their nitrogen from the atmosphere after 6 months of inoculation, which increased up to 53% after 12 months (Table 3). Specifically, strains AN1r, AR1r and SN1r fixed the highest amounts of nitrogen (50% or higher) among all bacterial strains after 1 year. Since lodgepole pine seeds could have contained native unculturable nitrogen-fixing bacteria, the % Ndfa reported here is an estimation of the BNF contributed by the inoculated bacteria over the BNF which could have occurred in non-inoculated control seedlings.
Atom % 15N excess in foliage and % nitrogen derived from atmosphere (Ndfa) by each bacterial strain in association with lodgepole pine (Pinus contorta) seedlings at 6- and 12-month harvests.
Treatment . | 6-month harvest . | 12-month harvest . | ||
---|---|---|---|---|
. | Atom % 15N excess in foliage . | Ndfa (%) . | Atom % 15N excess in foliage . | Ndfa (%) . |
AS1r | 0.80 ± 0.03*,a | 11 | 0.48 ± 0.02bc | 29 |
AN1r | 0.78 ± 0.01b | 14 | 0.34 ± 0.03de | 50 |
AN2r | 0.77 ± 0.01b | 14 | 0.38 ± 0.02cd | 45 |
AN3r | 0.76 ± 0.01b | 15 | 0.41 ± 0.03bcd | 41 |
AN4r | 0.79 ± 0.02a | 12 | 0.46 ± 0.01bc | 32 |
AN5r | 0.74 ± 0.01b | 17 | 0.45 ± 0.02bc | 34 |
AR1r | 0.76 ± 0.01b | 15 | 0.32 ± 0.02de | 53 |
SS1r | 0.77 ± 0.03b | 14 | 0.48 ± 0.02bc | 30 |
SS2r | 0.78 ± 0.04b | 13 | 0.50 ± 0.02bc | 27 |
SS3r | 0.84 ± 0.04a | 6 | 0.52 ± 0.02b | 23 |
SN1r | 0.80 ± 0.01a | 11 | 0.34 ± 0.03de | 51 |
SN2r | 0.76 ± 0.04b | 15 | 0.40 ± 0.03bcd | 41 |
SR1r | 0.82 ± 0.02a | 8 | 0.52 ± 0.02b | 24 |
SR2r | 0.80 ± 0.02a | 11 | 0.42 ± 0.03bcd | 38 |
Control | 0.90 ± 0.01a | - | 0.68 ± 0.03a | - |
Treatment . | 6-month harvest . | 12-month harvest . | ||
---|---|---|---|---|
. | Atom % 15N excess in foliage . | Ndfa (%) . | Atom % 15N excess in foliage . | Ndfa (%) . |
AS1r | 0.80 ± 0.03*,a | 11 | 0.48 ± 0.02bc | 29 |
AN1r | 0.78 ± 0.01b | 14 | 0.34 ± 0.03de | 50 |
AN2r | 0.77 ± 0.01b | 14 | 0.38 ± 0.02cd | 45 |
AN3r | 0.76 ± 0.01b | 15 | 0.41 ± 0.03bcd | 41 |
AN4r | 0.79 ± 0.02a | 12 | 0.46 ± 0.01bc | 32 |
AN5r | 0.74 ± 0.01b | 17 | 0.45 ± 0.02bc | 34 |
AR1r | 0.76 ± 0.01b | 15 | 0.32 ± 0.02de | 53 |
SS1r | 0.77 ± 0.03b | 14 | 0.48 ± 0.02bc | 30 |
SS2r | 0.78 ± 0.04b | 13 | 0.50 ± 0.02bc | 27 |
SS3r | 0.84 ± 0.04a | 6 | 0.52 ± 0.02b | 23 |
SN1r | 0.80 ± 0.01a | 11 | 0.34 ± 0.03de | 51 |
SN2r | 0.76 ± 0.04b | 15 | 0.40 ± 0.03bcd | 41 |
SR1r | 0.82 ± 0.02a | 8 | 0.52 ± 0.02b | 24 |
SR2r | 0.80 ± 0.02a | 11 | 0.42 ± 0.03bcd | 38 |
Control | 0.90 ± 0.01a | - | 0.68 ± 0.03a | - |
*Mean ± standard error; n = 10 seedlings per treatment
Values with different letters are significantly different (P < 0.05)
Atom % 15N excess in foliage and % nitrogen derived from atmosphere (Ndfa) by each bacterial strain in association with lodgepole pine (Pinus contorta) seedlings at 6- and 12-month harvests.
Treatment . | 6-month harvest . | 12-month harvest . | ||
---|---|---|---|---|
. | Atom % 15N excess in foliage . | Ndfa (%) . | Atom % 15N excess in foliage . | Ndfa (%) . |
AS1r | 0.80 ± 0.03*,a | 11 | 0.48 ± 0.02bc | 29 |
AN1r | 0.78 ± 0.01b | 14 | 0.34 ± 0.03de | 50 |
AN2r | 0.77 ± 0.01b | 14 | 0.38 ± 0.02cd | 45 |
AN3r | 0.76 ± 0.01b | 15 | 0.41 ± 0.03bcd | 41 |
AN4r | 0.79 ± 0.02a | 12 | 0.46 ± 0.01bc | 32 |
AN5r | 0.74 ± 0.01b | 17 | 0.45 ± 0.02bc | 34 |
AR1r | 0.76 ± 0.01b | 15 | 0.32 ± 0.02de | 53 |
SS1r | 0.77 ± 0.03b | 14 | 0.48 ± 0.02bc | 30 |
SS2r | 0.78 ± 0.04b | 13 | 0.50 ± 0.02bc | 27 |
SS3r | 0.84 ± 0.04a | 6 | 0.52 ± 0.02b | 23 |
SN1r | 0.80 ± 0.01a | 11 | 0.34 ± 0.03de | 51 |
SN2r | 0.76 ± 0.04b | 15 | 0.40 ± 0.03bcd | 41 |
SR1r | 0.82 ± 0.02a | 8 | 0.52 ± 0.02b | 24 |
SR2r | 0.80 ± 0.02a | 11 | 0.42 ± 0.03bcd | 38 |
Control | 0.90 ± 0.01a | - | 0.68 ± 0.03a | - |
Treatment . | 6-month harvest . | 12-month harvest . | ||
---|---|---|---|---|
. | Atom % 15N excess in foliage . | Ndfa (%) . | Atom % 15N excess in foliage . | Ndfa (%) . |
AS1r | 0.80 ± 0.03*,a | 11 | 0.48 ± 0.02bc | 29 |
AN1r | 0.78 ± 0.01b | 14 | 0.34 ± 0.03de | 50 |
AN2r | 0.77 ± 0.01b | 14 | 0.38 ± 0.02cd | 45 |
AN3r | 0.76 ± 0.01b | 15 | 0.41 ± 0.03bcd | 41 |
AN4r | 0.79 ± 0.02a | 12 | 0.46 ± 0.01bc | 32 |
AN5r | 0.74 ± 0.01b | 17 | 0.45 ± 0.02bc | 34 |
AR1r | 0.76 ± 0.01b | 15 | 0.32 ± 0.02de | 53 |
SS1r | 0.77 ± 0.03b | 14 | 0.48 ± 0.02bc | 30 |
SS2r | 0.78 ± 0.04b | 13 | 0.50 ± 0.02bc | 27 |
SS3r | 0.84 ± 0.04a | 6 | 0.52 ± 0.02b | 23 |
SN1r | 0.80 ± 0.01a | 11 | 0.34 ± 0.03de | 51 |
SN2r | 0.76 ± 0.04b | 15 | 0.40 ± 0.03bcd | 41 |
SR1r | 0.82 ± 0.02a | 8 | 0.52 ± 0.02b | 24 |
SR2r | 0.80 ± 0.02a | 11 | 0.42 ± 0.03bcd | 38 |
Control | 0.90 ± 0.01a | - | 0.68 ± 0.03a | - |
*Mean ± standard error; n = 10 seedlings per treatment
Values with different letters are significantly different (P < 0.05)
DISCUSSION
In this study, we characterized the nitrogen-fixing potential of lodgepole pine endophytes through in vitro microbiological techniques (ARA and nifH gene amplification) as well as in planta inoculation in a greenhouse study. We quantified and compared the amount of nitrogen fixed and plant-growth promoted (biomass and length) by bacteria, previously isolated from internal tissues of lodgepole pine trees growing on gravel mining sites. Our results provide the first evidence that BNF by bacteria capable of endophytic colonization could be a significant nitrogen pathway for lodgepole pine trees thriving on highly disturbed bare gravel sites. These findings support the overall hypothesis that native culturable endophytic bacterial communities of lodgepole pine can re-colonize their plant host along with providing them with substantial amounts of fixed nitrogen and consequently stimulating their growth. Given that the soil nitrogen levels at the gravel mining sites were extremely low (Chapman and Paul 2012), coupled with the lack of nitrogen-fixing nodulating plant species in the vicinity of the sampling sites (Padda, Puri and Chanway 2018), our observations strengthen the postulation that gravel pit pine trees harbor endophytic bacteria as an evolutionary approach to meet their nitrogen demands.
Using the conventional ARA technique (a process analogous to BNF), we confirmed the nitrogenase enzyme activity of antibiotic-resistant derivatives of endophytic bacterial strains previously isolated and reported in Padda, Puri and Chanway (2018). We found that ethylene production rates did not vary between the antibiotic-resistant and the wild-type strains, which is consistent with previous results of Shishido, Loeb and Chanway (1995) and Bal et al. (2012), thus indicating that antibiotic-resistance mutation had no effect on the nitrogen-fixing ability of the bacteria. Fourteen potential endophytic diazotrophs that showed consistently positive results for nitrogenase activity in duplicate assays were selected for the greenhouse study (Tables 1 and 2). Since ARA is an indirect method mostly used to qualitatively determine the nitrogen-fixing potential of bacteria (Brighigna et al.1992; Lin et al.2012), we subsequently used more direct and robust methods in this study—nifH gene amplification and a 15N isotope dilution assay. Each of the 14 selected endophytic strains that reduced acetylene to ethylene in the ARA was found positive for the presence of the nifH gene by PCR amplification (Table 2). Often a particular nifH primer set is not appropriate to amplify the nifH gene of all bacterial strains (Doty et al.2009), which is why different primer sets were used in this study.
From the results of 15N isotope dilution assay, we infer that every diazotrophic bacteria used in the greenhouse study significantly contributed to the nitrogen requirements of lodgepole pine seedlings after one year through BNF (Table 3), which supports our hypothesis that these bacteria play a considerable role in sustaining the growth of lodgepole pine seedlings at highly nitrogen-poor gravel mining sites. Since pine seedlings were grown in a nitrogen-limited environment and nitrogen was provided only once at the onset of the greenhouse experiment with a concentration of 0.001 g nitrogen per Cone-tainer, it is logical to assume that soil nitrogen would eventually get depleted thereby triggering the nitrogen-fixation mechanism of diazotrophs. Initially, limited nitrogen was derived from the atmosphere by the inoculated seedlings at the 6-month harvest, however, with time, the proportion of derived nitrogen increased nearly 3- to 4-fold for all bacteria-inoculated treatments. Our results are consistent with previous research findings, supporting the theory that in planta nitrogen-fixation by endophytic diazotrophs becomes more prominent as the soil nitrogen levels decrease with time (Bal and Chanway 2012a; Yang et al.2016). Likewise, the quantity of nitrogen fixed from the atmosphere by nodulating plants and their symbionts significantly reduces in nitrogen-rich environments (Danso, Hera and Douka 1987; Vargas, Mendes and Hungria 2000). This can be attributed to the energy-intensive nature of the BNF process, driving plants to invest in this process only when other substantial sources of nitrogen (like soil nitrogen) are either rare or absent (Reed, Cleveland and Townsend 2011). Since it remains unclear how the specific needs of endophytes to perform BNF are met (i.e. how they deal with oxygen that obstructs nitrogenase activity or how they attain energy and macro- and micro-nutrients to carry out this reaction), future research in the direction of characterizing the in situ colonization patterns and gene expressions could elucidate this under-explored yet important nitrogen source (Wurzburger 2016). Additionally, the foliar nitrogen content of all bacteria-inoculated seedlings was greater than the controls, with nine bacterial treatments accumulating 200% or higher foliar nitrogen in pine needles (Fig. 4B). These results imply that bacteria-inoculated seedlings had access to an additional source of nitrogen, likely atmospheric nitrogen (14N) as indicated by their lower atom % 15N values (Table 3). In addition, the larger, branched root network of bacteria-inoculated pine seedlings could have played a part in gathering more nitrogen from the growth media as compared to the control (Fig. S1, Supporting Information). Furthermore, the extended root and shoot system of inoculated seedlings may have also been partially responsible for the dilution of 15N observed in their foliar tissues (Table 3).
Among all bacterial treatments, P. graminis AN1r, P. migulae AR1r and P. lini SN1r inoculated seedlings acquired highest amounts of nitrogen via BNF (nearly 50%) (Table 3), which is comparable with the amount of nitrogen fixed by endophytic strains of poplar (Populus trichocarpa) (Knoth et al.2014), lodgepole pine (Anand, Grayston and Chanway 2013) and western red cedar (Bal and Chanway 2012b). It is noteworthy that these three strains belong to Pseudomonas, a genus well-known for its ability to provide various benefits to plants including BNF (reviewed by Puri, Padda and Chanway 2017, 2018). Interestingly, P. graminis strains have been isolated from poplar trees growing at riverbank gravel sites in Washington, USA (Doty et al.2009) and reported for their ability to fix nitrogen and enhance poplar growth in greenhouse and field conditions (Knoth et al.2014). To the best of our knowledge, our study is the first to quantify the in planta nitrogen-fixation by P. migulae and P. lini. Previously, the nitrogen-fixing ability of these bacterial species has only been verified in vitro using nifH gene amplification (Suyal et al.2014; Ehsan et al.2016).
The seedling length and biomass results demonstrate that bacterial inoculation stimulated the growth of lodgepole pine seedlings under nitrogen-poor conditions (Fig. 3 and Fig. S1, Supporting Information). Variation in biomass accumulation was observed between different bacterial treatments. Inoculation with strains P. graminis AN1r, Rathayibacter tanaceti AN2r, Frigoribacterium endophyticum AN3r, P. lini SN1r and Flavobacterium aquidurense SN2r consistently promoted seedling growth, predominantly biomass (∼2-fold or higher), throughout the greenhouse study (Fig. 3). Apart from that, a significant upward trend in biomass allocation was noted for P. lini SN1r and P. migulae AR1r inoculated seedlings with nearly 3- and 4-fold increases, respectively, between 4- and 12-month harvests (Fig. 3A and C). Anand and Chanway (2013) reported similar results for western red cedar where the rate of growth promotion after endophytic inoculation escalated considerably with time. The growth enhancement observed for pine seedlings inoculated with these six strains could be linked to the significantly greater foliar nitrogen content (>300%) in these seedlings as well as the substantial amounts of fixed nitrogen accumulated by these strains (>40%). A similar trend has been reported for growth stimulation as a result of endophytic nitrogen-fixation in deciduous and coniferous tree species (Knoth et al.2014; Tang et al.2017). In addition, it should be noted that other plant-growth-promoting mechanisms such as phytohormone modulation, phosphate solubilization and siderophore production could also be involved in the pine growth enhancement observed in this study and require further evaluation. Pseudomonas graminis bacterial strains have been reported to stimulate the growth of poplar trees via nitrogen-fixation and phytohormone modulation through indole-3-acetic acid production (IAA) (Knoth et al.2014). Similarly, P. lini strains were documented for their in vitro plant growth-promoting abilities viz., IAA production, phosphate solubilization, siderophore production and nifH gene amplification along with their ability to enhance length and biomass of wheat (Triticum aestivum L.) crop upon inoculation (Kim et al.2011; Ehsan et al.2016). Rathayibacter tanaceti and F. endophyticum species are novel plant-growth-promoting and nitrogen-fixing actinobacterial species, which have never been reported previously as plant-beneficial bacteria. Although in vitro plant-growth-promotion abilities of P. migulae and F. aquidurense species have been demonstrated (Suyal et al.2014; Xu 2014), they have not been previously tested in planta for growth promotion. Therefore, to the best of our knowledge, this is the first study to report in planta growth promotion and nitrogen-fixation for these four bacterial species.
Each bacterial strain tested in the greenhouse study was able to successfully colonize at least one or more of the internal root, stem or needle tissues of lodgepole pine seedlings with population densities comparable to those observed in tree species like hybrid spruce, poplar and western red cedar (Shishido and Chanway 2000; Germaine et al.2004; Anand and Chanway 2013). Interestingly, the four needle-colonizers of pine seedlings in this study—P. graminis AN1r, R. tanaceti AN2r, P. lini SN1r and F. aquidurense SN2r—were originally isolated from needle tissues of pine trees growing on the gravel mining pits (Table 1; Fig. 1). One possible explanation for inhabiting pine needles could be that these bacteria have easy access to carbohydrates due to the prevalence of photosynthesis in needle tissues, which is crucial for fueling nitrogenase enzyme activity and bacterial growth. This is consistent with the findings of Germaine et al. (2004) which supported the concept of tissue-specific colonization by Pseudomonas species within poplar trees. Since root hairs offer a logical point of entry for endophytic bacteria, it may be the reason that all bacterial inoculants colonized root tissues (Kandel, Joubert and Doty 2017). Substantial seedling growth promotion was observed particularly for those bacterial treatments (AN1r, AN2r, AN3r, AR1r, SN1r, SN2r and SR2r) that colonized more than one tissue type of pine seedlings in biologically significant numbers (Figs 1, 2 and 3). The ability of these strains to fix considerable amounts of nitrogen and stimulate pine growth could be linked to their potential to survive and proliferate in multiple tissues of the host plant (Anand, Grayston and Chanway 2013). As summarized in Fig. 5, endophytic colonization had a strong correlation with nitrogen-fixation (R2 = 0.81) as well as seedling biomass (R2 = 0.93) at the 12-month harvest. These results suggest that the endophytic colonization potential of diazotrophic bacteria may affect their ability to fix nitrogen and promote plant growth (Fig. 5). Notably, the endophytic population sizes of strains AN1r, AR1r and SN1r were highest among all bacterial strains, which is consistent with the nitrogen-fixation and biomass accumulation results observed for these three strains (Fig. 5). Therefore, it can be concluded that the best endophytic colonizers of pine trees were the best nitrogen-fixers and plant-growth-promoters in this greenhouse trial. However, the growth enhancement and nitrogen-fixation observed could also be in part due to external colonization by these bacterial strains (not checked in this study). Therefore, we can't definitively conclude that the endophytic population was solely responsible for the observed effects. Moreover, the unculturable bacteria present in the plant microbiome could also be playing a crucial role in the survival and growth of pine trees at the gravel mining sites, which needs to be further explored. In addition, the performance of bacteria that showed highest potential in this study should also be examined under natural conditions at these sites, i.e. when they are subjected to biotic and abiotic stresses.
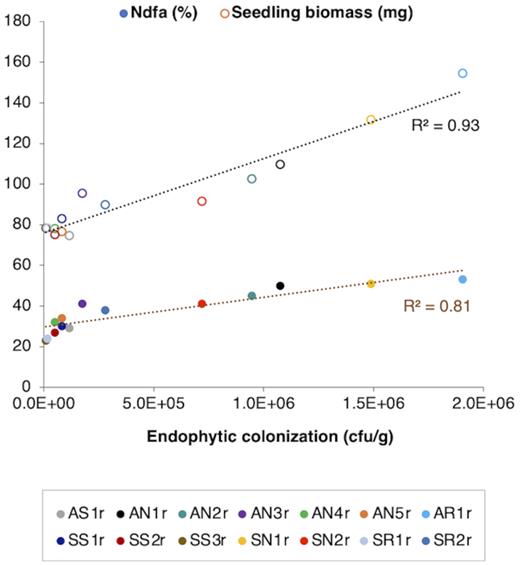
Correlation of endophytic population sizes of bacterial strains in lodgepole pine tissues (aggregated for all tissue types) with seedling biomass and % nitrogen derived from the atmosphere (Ndfa), 1 year after inoculation.
In conclusion, our study highlights the potential ecological role that endophytic bacteria could be playing in naturally restoring highly disturbed gravel mining sites in BC by stimulating the growth of native lodgepole pine trees. These sites are regarded as one of the most nitrogen-limited environments where trees (lodgepole pine) have been found to grow (Chapman and Paul 2012) and based on our results, we can conclude that endophytic bacteria capable of fixing nitrogen could be a significant nitrogen source for these trees. Forming associations with these bacteria could be essential for survival and growth of pine trees under extremely nitrogen-poor environments at these sites, where significant biological nitrogen inputs from other sources were absent. Pine trees at these pits could be relying heavily on bacteria from members of the Pseudomonas genus since the top three strains (P. graminis AN1r, P. migulae AR1r and P. lini SN1r) that showed the greatest potential for nitrogen-fixation and plant growth enhancement in the greenhouse study were pseudomonads. We believe that these three Pseudomonas strains should be further evaluated with pine trees in field-based studies to better evaluate the potential of this symbiotic association for effective reclamation of highly disturbed sites in both natural and managed ecosystems.
ACKNOWLEDGEMENTS
The authors are grateful to Dr William Kenneth Chapman for his pioneering research idea to study gravel mining sites in BC. Special thanks to Clive Dawson and Andre Bindon from Analytical Chemistry Services Laboratory, BC Ministry of Environment & Climate Change Strategy for their help in acetylene reduction experiments.
FUNDING
This work was supported by the Natural Sciences and Engineering Research Council of Canada (NSERC) Discovery Grant (RGPIN 41832–13) to Dr Chris P. Chanway.
Conflict of interest. None declared.