-
PDF
- Split View
-
Views
-
Cite
Cite
Jinghuan Luo, Hui Chen, Xiaoyu Han, Yanfang Sun, Zhiguo Yuan, Jianhua Guo, Microbial community structure and biodiversity of size-fractionated granules in a partial nitritation–anammox process, FEMS Microbiology Ecology, Volume 93, Issue 6, June 2017, fix021, https://doi.org/10.1093/femsec/fix021
- Share Icon Share
Abstract
The performance of a granule-based partial nitritation–anammox process is expected to be affected by the granule size distribution, but little is known about the impact of granule size on microbial community structure and diversity. To reveal how the microbial composition and diversity vary with granule size, granules from a partial nitritation–anammox reactor were size-fractionated into five classes (<0.2, 0.2–0.5, 0.5–0.8, 0.8–1.0 and >1.0 mm). Microbial communities and diversity in these size-fractionated granules were investigated using 16S rRNA gene high-throughput sequencing. It was found that larger granules harbor more diverse microbial communities than small granules. Both quantitative PCR and 16S rRNA gene sequencing indicated that the abundance of anammox bacteria (dominated by Candidatus Brocadia) exhibited an increasing trend with granule size. In contrast, the abundance of ammonia-oxidizing bacteria (Nitrosomonas) decreased with increasing granule size. Moreover, larger granules harbored more diverse anammox bacteria, with four genera found in the largest granules while only two with limited abundance were detected in the smallest granules. The findings highlight an important role for granule size in shaping community structure and biodiversity.
INTRODUCTION
Anaerobic ammonium oxidation (anammox) bacteria have the unique metabolic ability to oxidize ammonium with nitrite to form nitrogen gas (Strous et al. 1999). As an economical and environmentally friendly process, autotrophic nitrogen removal through coupling partial nitritation with anammox reactions has been successfully used to treat various types of wastewaters, in particular those containing a high concentration of nitrogen but a relatively low level of organic carbon, such as digester supernatant (Joss et al. 2009) and landfill leachate (Li et al. 2014). In a one-stage partial nitritation–anammox (PNA) process, ammonia-oxidizing bacteria (AOB) oxidize approximately half of the ammonium to nitrite, while the anammox bacteria convert the nitrite formed by the AOB and the remaining ammonium to dinitrogen gas. Compared with the conventional nitrification–denitrification process, the PNA process does not require organic carbon and also requires much less oxygen (about 60% less) (Guo et al. 2013; Lackner et al. 2014). In addition, the PNA process produces much less waste sludge requiring further treatment and disposal.
Due to the slow growth rate of anammox bacteria (with a maximum doubling time of 3–7 days reported recently; Kartal et al. 2012; Lotti et al. 2014a; Lotti et al. 2015a), adequate biomass retention should be a prerequisite for the successful implementation of the PNA process. Granular sludge, a kind of compact and dense bacterial aggregate with much better settling properties than flocculated sludge (Lemaire, Webb and Yuan 2008), is therefore commonly adopted to achieve an efficient retention of anammox bacteria in bioreactors (Gonzalez-Gil et al. 2015). To date, there are over 100 full-scale plants worldwide employing the PNA process, in which more than 30 plants employ a granule-based PNA process (Lackner et al. 2014). Through microbial auto-immobilization, each granule is composed of a complex mixture of symbiotic microorganisms. Synergistic or competitive relationships among the millions of microbes in a granule are necessary, and thus the consortium shapes a special microbial ecosystem (McHugh et al. 2003). In this sense, each granule is a micro-level model system for microbial ecology. In a one-stage PNA process, a granule comprises both an aerobic outer layer for AOB and an anaerobic inner layer for anammox bacteria, which can cooperate to achieve autotrophic nitrogen removal (Wang et al. 2014; Speth et al. 2016). Therefore, the study of anammox-based granular communities has obvious applied significance; however, the importance of granular sludge as a micro-level model system for microbial ecology has been largely overlooked so far.
One of the most crucial parameters for a granular PNA process is the granule size, which determines the surface/volume ratio, and also the aerobic–anaerobic zone separation in a granule. The granule size also affects substrate transport/transfer in granules and further the abundance and activity of functional microorganisms (Volcke et al. 2012; Wang et al. 2014). A few studies have indicated that nitrogen removal performance or microbial community structure in PNA-based systems could be significantly affected by the granule size distribution (Vlaeminck et al. 2010; Volcke et al. 2012; Wang et al. 2014). Generally, larger granules were found to be more desirable for the anammox reaction mainly owing to a lower oxygen penetration in an increased granule size, while smaller granules seemed to accumulate nitrite or nitrate (Nielsen et al. 2005; Vlaeminck et al. 2008; Volcke et al. 2012). Meanwhile, other studies reported higher total nitrogen removal by smaller granules than larger ones, since the ammonium oxidation capacity of AOB could be the rate-limiting factor for the PNA process (Volcke et al. 2010; Wang et al. 2014). However, previous studies primarily focused on modelling how granule size affects the process performance by mathematical simulations (Volcke et al. 2010; Volcke et al. 2012), or qualifying abundances of key functional microorganisms (including AOB, nitrite-oxidizing bacteria (NOB) and anammox bacteria) by using real-time quantitative PCR (qPCR) (Wang et al. 2014) or fluorescence in situ hybridization (FISH) (Nielsen et al. 2005; Vlaeminck et al. 2008). Little is known of whether granules with different particle sizes shape different global microbial communities, and whether larger or smaller granules would harbor a higher global microbial diversity in the PNA-based systems (Guo et al. 2016). In addition, the global microbial biodiversity plays a significant role in functional stability and resource use efficiency of an ecosystem (Girvan et al. 2005; Ptacnik et al. 2008), but few studies are available of microbial biodiversity in granular PNA reactors so far.
In comparison with conventional molecular biological approaches (e.g. PCR or FISH) that detect insufficient sequences and underestimate the biodiversity (Wang et al. 2016), the high-throughput sequencing developed in recent years can provide relatively comprehensive information for microbial communities (Guo et al. 2015; Goodwin, McPherson and McCombie 2016). Using high-throughput sequencing, thousands of phylogenetically informative gene fragments can be sequenced with the overwhelming superiority of unprecedented sequencing depth (Glenn 2011). It can detect qualitative and quantitative microbial information even for the sub-dominant groups with a rather low abundance of 0.01–0.1% (Guo and Zhang 2012), thus providing an accurate technique to describe microbial community structure in a complex microbial ecosystem. Based on these advantages, high-throughput sequencing has been successfully employed for the observation of anammox-based communities (Gilbert et al. 2014; Chu et al. 2015). For example, Bagchi et al. (2016) recently reported that larger granules showed more abundant anammox bacteria than smaller ones in a granule-based anammox reactor by using high-throughput sequencing and qPCR techniques. However, to the best of our knowledge, there have not been studies reported on the global microbial structure in different granule sizes in a PNA reactor with the use of this high-throughput technology.
This study aims to dissect microbial communities and compare diversity in size-fractionated anammox-based granules, by recognizing a granule micro-level model system. We collected five granular sludge samples with different sizes from a lab-scale PNA reactor and preformed Illumina MiSeq high-throughput sequencing. Global microbial communities and unique species were characterized in granules of different size. The functional microorganisms involving in nitrogen conversion are further discussed. The results will help to better understand the effect of granule size on microbial community in the PNA-based process, and highlight the importance of recognizing granules as a micro-level ecological system.
MATERIALS AND METHODS
Bioreactor operation and sampling
Granular sludge samples were collected from a lab-scale sequencing batch reactor (SBR) performing autotrophic nitrogen removal, with a working volume of 120 L. Before the sampling, the reactor had been operated continuously for more than 1 year. The influent of the PNA reactor was the effluent from a primary clarifier, from a full-scale wastewater treatment plant (located in Beijing, China) with NH4HCO3 added. Figure 1 shows the red granular sludge developed in this reactor at the time of the sampling.
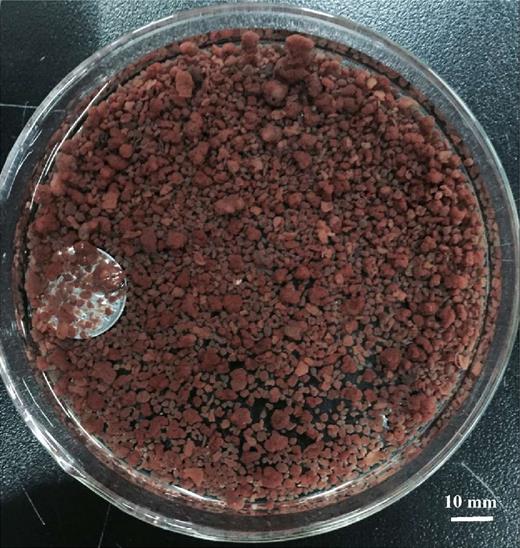
Adequate mixing was provided to ensure a homogeneous biomass contribution. To further ensure representative samples were obtained, sludge (100 mL) was sampled from three points (in the bottom, middle and upper regions, approximately 33 mL from each point) in the reactor. Then 100 mL granule samples were divided into five subsamples by the wet-sieving method using a series of sieves (0.2, 0.5, 0.8 and 1.0 mm sized mesh) (Laguna et al. 1999). These subsamples included S1 (<0.2 mm), S2 (0.2–0.5 mm), S3 (0.5–0.8 mm), S4 (0.8–1.0 mm) and S5 (>1.0 mm) for further DNA extractions.
DNA extraction, qPCR and 16S rRNA gene sequencing
DNA was immediately extracted using the FastDNA SPIN Kit for Soil (MP Biomedicals, Santa Ana, CA, USA) according to the manufacturer's instructions. Following DNA extraction, the integrity of the DNA was verified using gel electrophoresis. The concentration and purity of extracted DNA were determined with a NanoDrop 2000 spectrophotometer (Thermo Fisher Scientific, Waltham, MA, USA).
Genes copy numbers of AOB, NOB, anammox bacteria and the universal bacterial 16S rRNA genes for the total bacteria were quantified using an Applied Biosystems 7300 Real-time PCR system (ABI, USA). qPCR of total bacteria was performed with the primers 341f and 534r (Koike et al. 2007), and the qPCR assay of anammox bacteria was performed with the primers Amx-368f and Amx-820r (Zhu et al. 2011) under the condition previously described (Guo et al. 2016). For AOB, the primers Bac518F and Bac786R (Park et al. 2010) were used. In addition, the qPCR assays of Nitrospira and Nitrobacter bacteria were performed with the primers 341f and NTSPAr (Geets et al. 2007), and the primers FGPS872f and FGPS1269r (Ahn, Yu and Chandran 2008), respectively. For the qPCR, the 25 μL of each reaction contained 1× Sybr Green I, 1× Dye (Takara), 200 nM of each primer, 0.5 mg mL−1 BSA, and 2 μL of the DNA template. As described in the previous study (Guo et al. 2016), triplicate PCR assays were conducted for 10-fold serially diluted standard plasmids, the appropriately diluted samples and the negative controls. Standard plasmids carrying the target genes were obtained by TA cloning and these were extracted using a TIANpure Mini Plasmid kit (Tiangen, China). Standard curves covered five to eight orders of magnitude with R2 greater than 0.99.
For high-throughput 16S rRNA gene sequencing, the barcoded bacterial universal primers 338F (5΄-barcode-ACTC-CTACGGGAGGCAGCA-3΄) and 806R (5΄-GGACTACHVGGGTW-TCTAAT-3΄), where barcode is an eight-base sequence unique to each sample, were used to amplify the V3–V4 regions of 16S rRNA genes. Extracted DNA was PCR amplified in triplicate: 20 μL mixture containing 4 μL 5× FastPfu Buffer, 2 μL dNTPs (2.5 mM), 0.8 μL each primer (5 μM), 0.4 μL FastPfu Polymerase (TransGen, China), and 10 ng template DNA. The reaction condition were: an initial denaturation at 98°C for 30 s, 30 cycles of denaturation at 98°C for 5 s, annealing at 56°C for 20 s and elongation at 72°C for 20 s, and a final elongation step at 72°C for 5 min. PCR products were analyzed and extracted from 2% agarose gel, which was further purified using AxyPrep DNA Gel Extraction Kit (Axygen Biosciences, Union City, CA, USA). The purified PCR products were then quantified using QuantiFluor™-ST (Promega, USA). Sequencing was carried out on an Illumina MiSeq platform (Illumina, USA) at Major-bio (Shanghai, China) according to standard protocols, producing 2 × 250 bp paired-end reads. Raw sequence data can be accessed from NCBI Sequence Read Archive (SRA) database (Accession Number: SRP080297).
Sequencing analysis
Raw sequence data were demultiplexed, quality-filtered using QIIME 1.17 software (Caporaso et al. 2010), and chimeric sequences were filtered out with UCHIME (Edgar et al. 2011) using the default setting. After the above filters were applied, the minimum number of selected sequences in five samples was 22 834. To fairly compare the five samples at the same sequencing depth, subsampling at depth of 22 834 sequences for each sample was performed to equalize sample sizes for further analysis. Then, high quality sequences were subsequently clustered into operational taxonomic units (OTUs) at 97% similarity level using UPARSE version 7.1 (Edgar 2013), and representative sequences from each OTU were aligned against the SILVA (SSU115) 16S rRNA database (Quast et al. 2013) using RDP Classifier (Wang et al. 2007) at a confidence threshold of 70%. Alpha diversity of OTU libraries was described using the Chao1, Shannon and abundance-based coverage estimator (ACE) metrics as implemented in QIIME (Caporaso et al. 2010). Phylogenetic analyses were conducted using the MEGA 6.06 program (Tamura et al. 2013). The phylogenetic tree of 16S rRNA gene sequences was constructed using the neighbor-joining topology. Bootstrap values were obtained from data resampling of 1000 replicates.
RESULTS
Microbial community overview
For achieving stable PNA process, the laboratory-scale SBR was operated under low dissolved oxygen (DO) conditions (0.2–0.9 mg L−1) for more than 1 year before sampling. The operational performance of this reactor at the sludge sampling time can be found in Table 1. Fed with ammonium at concentrations of 655.5 ± 58.2 (mean ± SD) mg N L−1, the reactor achieved stable nitrogen removal with the average effluent concentrations of ammonium, nitrite and nitrate being 54.3 ± 21.5, 9.2 ± 3.7 and 26.4 ± 7.2 mg N L−1, respectively. The ammonium removal efficiency was 92.1 ± 3.2%. The obtained maximum nitrogen conversion rate was 0.33 kg N m−3 d−1. An average nitrite to ammonium consumption ratio of 1.37 ± 0.31 was observed, which matches the theoretical anammox process stoichiometry (i.e. 1.32).
Reactor operational conditions and performance during the sampling time. Values shown as mean ± standard deviation. DO, dissolved oxygen COD, chemical oxygen demand.
Parameter . | Unit . | Value . |
---|---|---|
DO | mg L−1 | 0.46 ± 0.30 |
Temperature | °C | 27 ± 2 |
Sludge settling time | min | 30 |
Sludge retention time | d | 12–15 |
Influent COD | mg L−1 | 131 ± 50 |
Effluent COD | mg L−1 | 50 ± 20 |
COD removal efficiency | % | 63.4 ± 7.8 |
Influent ammonium concentration | mg N L−1 | 655.5 ± 58.2 |
Effluent ammonium concentration | mg N L−1 | 54.3 ± 21.5 |
Ammonium removal efficiency | % | 92.1 ± 3.2 |
Ammonium loading rate | kg N m−3 d−1 | 0.18 ± 0.09 |
Ammonium removal rate | kg N m−3 d−1 | 0.17 ± 0.07 |
Effluent nitrite concentration | mg N L−1 | 9.2 ± 3.7 |
Effluent nitrate concentration | mg N L−1 | 26.4 ± 7.2 |
Parameter . | Unit . | Value . |
---|---|---|
DO | mg L−1 | 0.46 ± 0.30 |
Temperature | °C | 27 ± 2 |
Sludge settling time | min | 30 |
Sludge retention time | d | 12–15 |
Influent COD | mg L−1 | 131 ± 50 |
Effluent COD | mg L−1 | 50 ± 20 |
COD removal efficiency | % | 63.4 ± 7.8 |
Influent ammonium concentration | mg N L−1 | 655.5 ± 58.2 |
Effluent ammonium concentration | mg N L−1 | 54.3 ± 21.5 |
Ammonium removal efficiency | % | 92.1 ± 3.2 |
Ammonium loading rate | kg N m−3 d−1 | 0.18 ± 0.09 |
Ammonium removal rate | kg N m−3 d−1 | 0.17 ± 0.07 |
Effluent nitrite concentration | mg N L−1 | 9.2 ± 3.7 |
Effluent nitrate concentration | mg N L−1 | 26.4 ± 7.2 |
Reactor operational conditions and performance during the sampling time. Values shown as mean ± standard deviation. DO, dissolved oxygen COD, chemical oxygen demand.
Parameter . | Unit . | Value . |
---|---|---|
DO | mg L−1 | 0.46 ± 0.30 |
Temperature | °C | 27 ± 2 |
Sludge settling time | min | 30 |
Sludge retention time | d | 12–15 |
Influent COD | mg L−1 | 131 ± 50 |
Effluent COD | mg L−1 | 50 ± 20 |
COD removal efficiency | % | 63.4 ± 7.8 |
Influent ammonium concentration | mg N L−1 | 655.5 ± 58.2 |
Effluent ammonium concentration | mg N L−1 | 54.3 ± 21.5 |
Ammonium removal efficiency | % | 92.1 ± 3.2 |
Ammonium loading rate | kg N m−3 d−1 | 0.18 ± 0.09 |
Ammonium removal rate | kg N m−3 d−1 | 0.17 ± 0.07 |
Effluent nitrite concentration | mg N L−1 | 9.2 ± 3.7 |
Effluent nitrate concentration | mg N L−1 | 26.4 ± 7.2 |
Parameter . | Unit . | Value . |
---|---|---|
DO | mg L−1 | 0.46 ± 0.30 |
Temperature | °C | 27 ± 2 |
Sludge settling time | min | 30 |
Sludge retention time | d | 12–15 |
Influent COD | mg L−1 | 131 ± 50 |
Effluent COD | mg L−1 | 50 ± 20 |
COD removal efficiency | % | 63.4 ± 7.8 |
Influent ammonium concentration | mg N L−1 | 655.5 ± 58.2 |
Effluent ammonium concentration | mg N L−1 | 54.3 ± 21.5 |
Ammonium removal efficiency | % | 92.1 ± 3.2 |
Ammonium loading rate | kg N m−3 d−1 | 0.18 ± 0.09 |
Ammonium removal rate | kg N m−3 d−1 | 0.17 ± 0.07 |
Effluent nitrite concentration | mg N L−1 | 9.2 ± 3.7 |
Effluent nitrate concentration | mg N L−1 | 26.4 ± 7.2 |
Analysis of the 16S rRNA genes from five samples revealed that microbial communities varied with granule size. At phylum level (Fig. 2), a wide variety of phyla were observed and each phylum presented diverse microbial abundance in different granule sizes. The dominant Proteobacteria and Bacteroidetes contributed significantly to the microbial community for granules of all sizes, similar to the results obtained from some full-scale anammox bioreactors (Gonzalez-Martinez et al. 2015). Proteobacteria was the most abundant phylum with a range of 34.1% (S5) to 56.0% (S1), while Bacteroidetes made up the second most predominant phylum (from 17.5% to 23.6%), followed by Chlorobi (3.5–10.0%), Spirochaetae (3.2–11.2%), Chloroflexi (2.1–7.2%) and Planctomycetes (0.2–20.9%). In addition, there were another 23 kinds of minor phyla, which mainly included Verrucomicrobia, SHA-109, Firmicutes, Acidobacteria and Cyanobacteria. In addition to the organisms described above, our sequence data showed that the abundance of Candidate division WS6 in larger granules (S3, S4 and S5) was higher than that in smaller granules (S1 and S2). Candidate division WS6, belonging to the ‘Candidate phyla radiation’, are often detected in anammox-based processes, but their functions are not clear. A recent genome-wide study predicted Candidate phyla WS6 could be located in the anammox granule core and support a fermentative lifestyle (Speth et al. 2016).
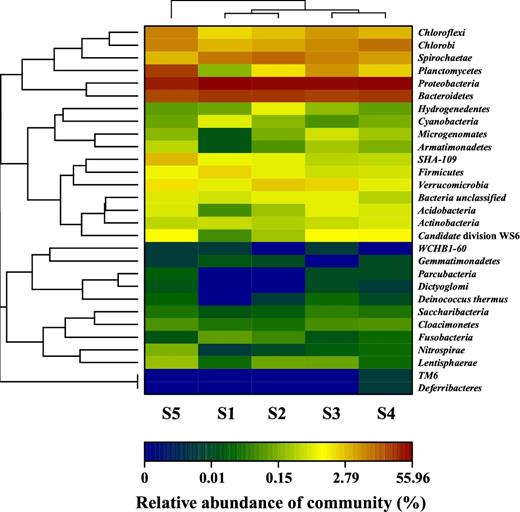
Microbial community structure and its relative abundance in granules of five size distributions at phylum level.
In order to present the similarity and difference among these granules, shared and unique OTUs in size-fractionated granules were analyzed as shown in a Venn diagram (Fig. 3). There were a total 565 OTUs observed, with 269 or 47.6% shared by five samples with different granule sizes. It should be noteworthy that 362 of the OTUs (i.e. accounting for 64.1% of the total OTUs) simultaneously existed in granules S3–S5, which means they have many common microbial species. Additionally, the smallest granule, S1, possessed only nine unique OTUs, among which Nitrosomonas (a well-known AOB) and Azospirillum (a free-living nitrogen-fixing bacterium) were found. The largest granule, S5, harbored 31 unique OTUs, which mainly included an uncultured species affiliated to Chlorobiales and Comamonas among many other uncultured bacteria. This implies many more nitrogen-conversion microbes in S5 than any other granules (details will be given later).
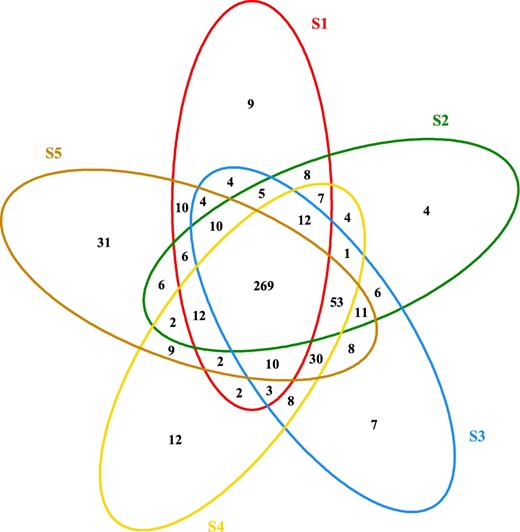
Biodiversity comparison in different size-fractionated granules
In order to reveal if larger granules harbor more diverse microbial communities, we compared the OTU number and alpha-diversity indices at the same sequencing depth of 22 834 sequences for each sample. Rarefaction curves of all granules at 97% of sequence similarity approached an asymptote, indicating their good coverage (all above 99.6%) for microbial diversity in the anammox-based granular sludge (data not shown). The number of OTUs for each granule size gradually increased from S1 (373) to S5 (473). Alpha-diversity indices (including ACE and Chao1 for richness, and Shannon H and Simpson D for diversity) obtained from these five anammox-based granules are further summarized in Table 2. In general, larger granules had a higher microbial richness, as estimators ACE and Chao1 progressively increased from 438 to 529 and from 448 to 547 with increasing granule size from <0.2 mm to >1 mm, respectively. In terms of microbial diversity, Shannon H rose from 4.02 in Sample S1 to 4.17 in Sample S5 (except for S4 a little lower than S3), whereas Simpson D showed the second lowest value of 0.0418 for S5 and the highest 0.0543 for S3 (to some extent, showing a downward trend). Generally, both increasing Shannon H and decreasing Simpson D indicate larger granules harbor a relatively higher biodiversity, compared with smaller granules in this anammox-based PNA system.
Microbial richness and diversity in granules with five size distributions. Values are means and 97% confidence interval.
. | . | . | OTU richness . | OTU diversity . | ||
---|---|---|---|---|---|---|
Sample . | Size (mm) . | OTU . | ACE . | Chao1 . | Shannon H . | Simpson D . |
S1 | <0.2 | 373 | 438 (414, 474) | 448 (415, 506) | 4.02 (4, 4.04) | 0.0388 (0.0379, 0.0398) |
S2 | 0.2–0.5 | 416 | 480 (457, 515) | 486 (456, 537) | 4.09 (4.07, 4.11) | 0.042 (0.0408, 0.0432) |
S3 | 0.5–0.8 | 441 | 509 (485, 546) | 524 (489, 584) | 4.09 (4.07, 4.11) | 0.0543 (0.0526, 0.0561) |
S4 | 0.8–1.0 | 436 | 496 (475, 530) | 523 (486, 590) | 4.04 (4.01, 4.06) | 0.0531 (0.0515, 0.0547) |
S5 | >1.0 | 473 | 529 (509, 560) | 547 (515, 602) | 4.17 (4.14, 4.19) | 0.0418 (0.0407, 0.0428) |
. | . | . | OTU richness . | OTU diversity . | ||
---|---|---|---|---|---|---|
Sample . | Size (mm) . | OTU . | ACE . | Chao1 . | Shannon H . | Simpson D . |
S1 | <0.2 | 373 | 438 (414, 474) | 448 (415, 506) | 4.02 (4, 4.04) | 0.0388 (0.0379, 0.0398) |
S2 | 0.2–0.5 | 416 | 480 (457, 515) | 486 (456, 537) | 4.09 (4.07, 4.11) | 0.042 (0.0408, 0.0432) |
S3 | 0.5–0.8 | 441 | 509 (485, 546) | 524 (489, 584) | 4.09 (4.07, 4.11) | 0.0543 (0.0526, 0.0561) |
S4 | 0.8–1.0 | 436 | 496 (475, 530) | 523 (486, 590) | 4.04 (4.01, 4.06) | 0.0531 (0.0515, 0.0547) |
S5 | >1.0 | 473 | 529 (509, 560) | 547 (515, 602) | 4.17 (4.14, 4.19) | 0.0418 (0.0407, 0.0428) |
Microbial richness and diversity in granules with five size distributions. Values are means and 97% confidence interval.
. | . | . | OTU richness . | OTU diversity . | ||
---|---|---|---|---|---|---|
Sample . | Size (mm) . | OTU . | ACE . | Chao1 . | Shannon H . | Simpson D . |
S1 | <0.2 | 373 | 438 (414, 474) | 448 (415, 506) | 4.02 (4, 4.04) | 0.0388 (0.0379, 0.0398) |
S2 | 0.2–0.5 | 416 | 480 (457, 515) | 486 (456, 537) | 4.09 (4.07, 4.11) | 0.042 (0.0408, 0.0432) |
S3 | 0.5–0.8 | 441 | 509 (485, 546) | 524 (489, 584) | 4.09 (4.07, 4.11) | 0.0543 (0.0526, 0.0561) |
S4 | 0.8–1.0 | 436 | 496 (475, 530) | 523 (486, 590) | 4.04 (4.01, 4.06) | 0.0531 (0.0515, 0.0547) |
S5 | >1.0 | 473 | 529 (509, 560) | 547 (515, 602) | 4.17 (4.14, 4.19) | 0.0418 (0.0407, 0.0428) |
. | . | . | OTU richness . | OTU diversity . | ||
---|---|---|---|---|---|---|
Sample . | Size (mm) . | OTU . | ACE . | Chao1 . | Shannon H . | Simpson D . |
S1 | <0.2 | 373 | 438 (414, 474) | 448 (415, 506) | 4.02 (4, 4.04) | 0.0388 (0.0379, 0.0398) |
S2 | 0.2–0.5 | 416 | 480 (457, 515) | 486 (456, 537) | 4.09 (4.07, 4.11) | 0.042 (0.0408, 0.0432) |
S3 | 0.5–0.8 | 441 | 509 (485, 546) | 524 (489, 584) | 4.09 (4.07, 4.11) | 0.0543 (0.0526, 0.0561) |
S4 | 0.8–1.0 | 436 | 496 (475, 530) | 523 (486, 590) | 4.04 (4.01, 4.06) | 0.0531 (0.0515, 0.0547) |
S5 | >1.0 | 473 | 529 (509, 560) | 547 (515, 602) | 4.17 (4.14, 4.19) | 0.0418 (0.0407, 0.0428) |
Key microorganisms for nitrogen conversion
Considering the PNA reactor is a nitrogen-driven ecosystem, we further unraveled key microorganisms involved in nitrogen conversion in five classes of size-fractionated granules. Overall, AOB were found in all granules (S1–S5), while anammox bacteria were far more abundant than other nitrogen-conversion microorganisms in the largest granules (S5). Figures 4 and 5 illustrate the abundance of Nitrosomonas (AOB, affiliated to β-Proteobacteria) was 10.6% in sample S1, and 4.7–7.1% in samples S2–S5. In comparison, the abundance of Candidatus Brocadia, one well-known anammox genus affiliated to Planctomycetales, increased substantially from 0–5.0% in samples S1–S4 to 19.4% in sample S5. The abundance of NOB including Nitrospira and Nitrobacter was very limited in all five samples, indicating stable partial nitritation was achieved in this PNA reactor. Specifically, Nitrospira appeared with a negligible abundance of less than 0.2% for all granule sizes, while Nitrobacter was not detected (Fig. 2). Similarly, Bagchi et al. (2016) also observed limited abundances of both Nitrospira-like (less than 0.01%) and Nitrobacter-like (less than 0.001%) bacteria in their granular anammox reactor during the whole experimental period. Meanwhile, genera capable of heterotrophic denitrification, including Thauera (1.5–19.1%), Saprospiraceae uncultured (7.1–11.9%) and Arcobacter (0.4–4.8%), had a high relative abundance in all sample classes.
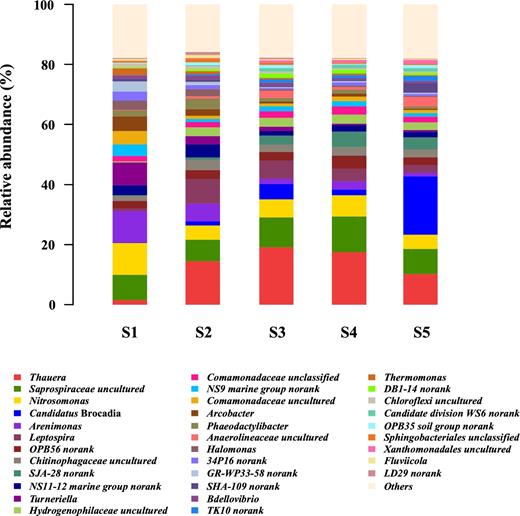
Microbial community structure and its relative abundance in granules with five size distributions at genus level.
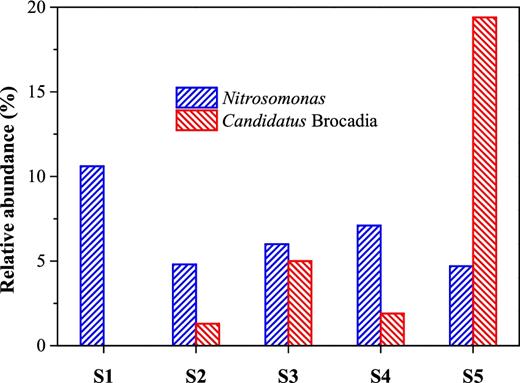
Abundance of Nitrosomonas (AOB) and Candidatus Brocadia (anammox bacteria) in granules with five size distributions.
Moreover, the abundance of nitrogen-relating populations in the five samples was further analyzed with qPCR. Both quantitative PCR and 16S rRNA gene sequencing indicated that the abundance of anammox bacteria exhibited an increasing trend with granule size (Table 3). The abundances of AOB and anammox detected by high-throughput sequencing were relatively lower than those by qPCR. For example, the qPCR approach had higher abundances of anammox bacteria in all granules (0.6 ± 0.2 to 26.8 ± 2.1%), compared with the high-throughput sequencing analysis (0–19.4%).
Abundances of AOB, NOB and anammox bacteria, based on real-time qPCR and high-throughput sequencing data, respectively. ND: not detectable. qPCR data shown as mean ± standard deviation.
Method . | Parameter . | S1 . | S2 . | S3 . | S4 . | S5 . |
---|---|---|---|---|---|---|
qPCR | AOB relative to total bacteria copies (%) | 14.6 ± 1.2 | 8.6 ± 0.9 | 7.2 ± 0.5 | 6.2 ± 0.7 | 6.3 ± 0.4 |
NOB relative to total bacteria copies (%) | <0.01 | <0.01 | <0.01 | <0.01 | <0.01 | |
Anammox relative to total bacteria copies (%) | 0.6 ± 0.2 | 5.4 ± 0.5 | 7.8 ± 0.9 | 8.5 ± 0.8 | 26.8 ± 2.1 | |
16S rRNA sequencing | AOB relative abundance (%) | 10.6 | 4.8 | 6.0 | 7.1 | 4.7 |
NOB relative abundance (%) | ND | ND | ND | ND | 0.2 | |
Anammox abundance (%) | ND | 1.3 | 5.0 | 1.9 | 19.4 |
Method . | Parameter . | S1 . | S2 . | S3 . | S4 . | S5 . |
---|---|---|---|---|---|---|
qPCR | AOB relative to total bacteria copies (%) | 14.6 ± 1.2 | 8.6 ± 0.9 | 7.2 ± 0.5 | 6.2 ± 0.7 | 6.3 ± 0.4 |
NOB relative to total bacteria copies (%) | <0.01 | <0.01 | <0.01 | <0.01 | <0.01 | |
Anammox relative to total bacteria copies (%) | 0.6 ± 0.2 | 5.4 ± 0.5 | 7.8 ± 0.9 | 8.5 ± 0.8 | 26.8 ± 2.1 | |
16S rRNA sequencing | AOB relative abundance (%) | 10.6 | 4.8 | 6.0 | 7.1 | 4.7 |
NOB relative abundance (%) | ND | ND | ND | ND | 0.2 | |
Anammox abundance (%) | ND | 1.3 | 5.0 | 1.9 | 19.4 |
Abundances of AOB, NOB and anammox bacteria, based on real-time qPCR and high-throughput sequencing data, respectively. ND: not detectable. qPCR data shown as mean ± standard deviation.
Method . | Parameter . | S1 . | S2 . | S3 . | S4 . | S5 . |
---|---|---|---|---|---|---|
qPCR | AOB relative to total bacteria copies (%) | 14.6 ± 1.2 | 8.6 ± 0.9 | 7.2 ± 0.5 | 6.2 ± 0.7 | 6.3 ± 0.4 |
NOB relative to total bacteria copies (%) | <0.01 | <0.01 | <0.01 | <0.01 | <0.01 | |
Anammox relative to total bacteria copies (%) | 0.6 ± 0.2 | 5.4 ± 0.5 | 7.8 ± 0.9 | 8.5 ± 0.8 | 26.8 ± 2.1 | |
16S rRNA sequencing | AOB relative abundance (%) | 10.6 | 4.8 | 6.0 | 7.1 | 4.7 |
NOB relative abundance (%) | ND | ND | ND | ND | 0.2 | |
Anammox abundance (%) | ND | 1.3 | 5.0 | 1.9 | 19.4 |
Method . | Parameter . | S1 . | S2 . | S3 . | S4 . | S5 . |
---|---|---|---|---|---|---|
qPCR | AOB relative to total bacteria copies (%) | 14.6 ± 1.2 | 8.6 ± 0.9 | 7.2 ± 0.5 | 6.2 ± 0.7 | 6.3 ± 0.4 |
NOB relative to total bacteria copies (%) | <0.01 | <0.01 | <0.01 | <0.01 | <0.01 | |
Anammox relative to total bacteria copies (%) | 0.6 ± 0.2 | 5.4 ± 0.5 | 7.8 ± 0.9 | 8.5 ± 0.8 | 26.8 ± 2.1 | |
16S rRNA sequencing | AOB relative abundance (%) | 10.6 | 4.8 | 6.0 | 7.1 | 4.7 |
NOB relative abundance (%) | ND | ND | ND | ND | 0.2 | |
Anammox abundance (%) | ND | 1.3 | 5.0 | 1.9 | 19.4 |
There were four anammox bacteria (Candidatus Kuenenia, Brocadia, Anammoxoglobus and Jettenia) clustered phylogenetically with known anammox lineages, sharing more than 97% sequence similarity with described anammox species (Fig. 6). All of four anammox phylotypes were affiliated to the family Brocadiaceae and accounted for 0.1–20.6% of the total bacterial 16S rRNA gene sequences in these five anammox granule samples. The abundance and composition of anammox communities in the granules, as revealed by sequencing of bacterial 16S rRNA gene amplicons and phylogenetic assignment to known anammox taxa, were highly distinct in each granule size. The total number of anammox sequences in the granules showed an upward tendency with increasing granule size, except for S3 with a number higher than S4. The first dominant anammox microorganism in all five samples was Candidatus Brocadia (Fig. 6). Interestingly, larger granules also harbor more diverse anammox bacteria. Four different anammox genera (i.e. Candidatus Kuenenia, Brocadia, Anammoxoglobus and Jettenia) can be observed in the largest granule, S5, with relatively high numbers of sequence reads. In contrast, only two anammox genera (i.e. Candidatus Brocadia and Jettenia) existed in the smallest granule, S1, with limited sequence reads (Fig. 6). Candidatus Anammoxoglobus with a limited abundance was detected in S1–S4 (<2 sequence reads), in comparison with the relatively high abundance in S5 (154 sequence reads).

Phylogenetic tree of anammox bacteria based on the obtained 16S rRNA gene sequences belong to anammox in this study and representative database sequences.
DISCUSSION
Larger granules harboring more diverse communities: implications
By recognizing anammox-based granules as a micro-level model system for microbial ecology, this study discussed the granule size-related microbial community composition and diversity. The results here for the first time revealed that larger granules harbor a higher microbial biodiversity compared with smaller ones. In addition to more diverse communities with general functions (such as fermentation and heterotrophic denitrification), larger granules also provide appropriate niches for the proliferation of more diverse anammox bacteria, with four distinct anammox genera found in the largest granules but only two in the smallest ones. Compared with floc-based or biofilm-based systems, it could be argued that granulation could result in a lower biodiversity as only microorganisms suitable for granule formation might be enriched. However, the substrate gradient in a granule could give rise to more ecological niches and thus a higher biodiversity (Fuchsman et al. 2011; Winkler et al. 2013). Our high-throughput sequencing results also suggest that the biodiversity in these granules increases with increasing granule size, and the largest granule size sustained the highest diversity.
The relationship between microbial biodiversity and functional stability of a natural ecosystem has been debated for decades (McCann 2000; Bell et al. 2005; Wittebolle et al. 2009). It is especially unclear for an engineered ecosystem (Saikaly and Oerther 2011). In the PNA reactor, as an engineered ecosystem, the community structure will be affected not only by operational conditions (including temperature, dissolved oxygen, influent composition; Daims, Taylor and Wagner 2006; Weissbrodt, Shani and Holliger 2014), but also by sludge aggregates (such as flocs, biofilms and granules; Guo et al. 2016). Although there are reports that reduced soil biodiversity showed no direct effects on resistance or resilience of key microbial groups after a disturbance (Griffiths et al. 2001; Wertz et al. 2007), generally a higher biodiversity is reported to enhance stability and productivity for a natural ecosystem, whereas a lower biodiversity usually negatively affects the functioning of a mono-enriched system (Rowan et al. 2003; Griffiths et al. 2004; Girvan et al. 2005; Ptacnik et al. 2008). When suffering from a disturbance, less abundant functional populations in a species-rich ecosystem can buffer and replace negatively affected groups to preserve the functional stability (Yachi and Loreau 1999). Briones and Raskin (2003) demonstrated that stability was better associated to functional redundancy than microbial diversity, i.e. the greater functional redundancy and complementation, the better functional stability. Granules incorporate functional stability into a compact structure, and this mainly relies on functionally important groups. Therefore, to achieve a better functional stability, our results might imply an engineered ecosystem (like a PNA reactor) should hold large rather than small granules, as large ones not only harbor a higher microbial diversity but also have a greater functional redundancy (e.g. both AOB and anammox bacteria, as well as more anammox genera than in small ones).
Nitrogen conversion microorganisms in different size-fractionated granules
The Illumina sequencing analysis shows that microbial communities and their abundances in a PNA reactor could be significantly affected by the granule size distribution. Concerning nitrogen conversion-related microbes, smaller granules were enriched by AOB, while larger granules were enriched by abundant anammox bacteria coupled with a fraction of AOB. This tendency is in accordance with some experimental or modelling findings by previous studies (Vlaeminck et al. 2010; Volcke et al. 2012), which reported a higher bacterial abundance or activity and a more favored conversion during this anammox-based process in larger granules. On one hand, the increasing granule size gives a relatively lower surface/volume ratio, thus resulting in a higher ammonium surface loading during this autotrophic nitrogen removal process. A shorter oxygen permeation depth and accordingly a less aerobic or more anaerobic space in a larger granule will therefore be formed, which provides spaces for anammox bacterial growth (Volcke et al. 2012). On the other hand, in a smaller granule with a higher surface/volume ratio, oxygen can permeate relatively deeper and provide a more sufficient aerobic space for the growth of AOB (de Kreuk et al. 2007). In addition, the performance of a granule-based reactor could also depend on the ratio of small to large granules, and further study is required to find an optimal ratio.
The genus Candidatus Brocadia were the dominant anammox bacteria for all five granules in this study. Gonzalez-Gil et al. (2015) and van der Star et al. (2007) found, using sequencing or FISH, that the dominant anammox bacteria (almost 100%) were Candidatus Brocadia in anammox granular sludges. It was reported that Brocadia-like anammox generally oxidize organic acids as their supplementary electron donor much more easily than Kuenenia-like anammox (Kartal et al. 2008), which might result in the predominant anammox Candidatus Brocadia in granules here with organic matter provided from the primary clarifier effluent (chemical oxygen demand 131 ± 50 mg L−1, see Table 1). Hu et al. (2010) also observed that the Brocadia anammox lineage dominated their reactors when supplied with organics, further suggesting Brocadia-like species could better handle organics. Interestingly, the genus Candidatus Jettenia still possessed a relatively high abundance in the smallest granules in our PNA reactor. This might imply that Candidatus Jettenia could have a higher tolerance for oxygen compared with other anammox phylotypes. Sequences affiliated to all anammox genera other than Candidatus Scalindua were detected. Observations of anammox bacterial diversity have demonstrated that Candidatus Scalindua was mostly found in saline-related environments (Sonthiphand, Hall and Neufeld 2014), so it is reasonable that no Candidatus Scalindua was detected in this engineered freshwater system.
The presence of NOB in PNA systems would negatively affect nitrogen removal efficiency, as these organisms compete for nitrite with anammox bacteria and produce nitrate. In the PNA system here, NOB were nearly suppressed due to high ammonium concentrations (>500 mg L−1) and low DO concentration (0.2–0.9 mg L−1), without negative effects on the reactor performance. In particular, the DO diffusion profile plays an important role on shaping functional microorganisms in different size-fractionated granules (Lotti et al. 2014b). Compared with AOB, NOB have a lower oxygen affinity (half-saturation constants of AOB to NOB: 0.6 vs 2.2 mg O2 L−1) (Volcke et al. 2010; Joss et al. 2011). The low DO concentration (0.2–0.9 mg L−1) controlled in the present PNA process might be favorable to NOB suppression. However, it was also reported that AOB would mainly occupy the more external layer, while NOB are relegated more internally (Lotti et al. 2015b), suggesting that NOB are more prone to the limiting DO condition in biofilm or granules. In this study, Nitrospira was detected but with an abundance of less than 0.2% in the largest granule (S5), while negligible in other smaller granules (S1–S4) in this PNA reactor. Moreover, various heterotrophic denitrifiers with a high abundance were found in all granular samples, presumably performing nitrate reduction during the autotrophic nitrogen removal process. This heterotrophic denitrification should be of great importance for an enhanced total nitrogen removal, since during an anammox reaction there is still 11% of the transformed nitrogen left in the effluent as nitrate (Ni et al. 2010). Mozumder et al. (2014) also demonstrated a better nitrogen removal performance when heterotrophs were present in a granule-based PNA reactor.
Overall, this study experimentally investigated the granule size-related microbial community composition and diversity. It revealed, for the first time, that larger granules possess a higher microbial biodiversity in comparison with small granules. For a PNA process, AOB and anammox bacteria should cooperate well in order to achieve a satisfactory completely autotrophic nitrogen removal. The findings above indicate that larger granules were enriched with both AOB and anammox bacteria, whereas smaller granules were dominated by AOB. A granule size of >1.0 mm provided favorable niches for both AOB and anammox bacteria, enabling partial nitritation and anammox reactions in the same granules. The results herein shed light on PNA granules as a micro-level model system for microbial ecology, and guide optimization of the autotrophic nitrogen removal process. Nonetheless, it should be further investigated whether larger granules enhance both biodiversity and functional redundancy, thus promoting the functional stability of this granular ecosystem, through using metagenomics, metatranscriptomics and metaproteomics.
Acknowledgments
This work was financially supported by the Australian Research Council Discovery Early Career Researcher Award (DE 130101401) and the University of Queensland Early Career Researcher Project. Jinghuan Luo would like to acknowledge the China Scholarship Council (CSC) for the scholarship support.
Conflict of interest. None declared.