-
PDF
- Split View
-
Views
-
Cite
Cite
Julie Mounier, Arantxa Camus, Isabelle Mitteau, Pierre-Joseph Vaysse, Philippe Goulas, Régis Grimaud, Pierre Sivadon, The marine bacterium Marinobacter hydrocarbonoclasticusSP17 degrades a wide range of lipids and hydrocarbons through the formation of oleolytic biofilms with distinct gene expression profiles, FEMS Microbiology Ecology, Volume 90, Issue 3, December 2014, Pages 816–831, https://doi.org/10.1111/1574-6941.12439
- Share Icon Share
Abstract
Hydrophobic organic compounds (mainly lipids and hydrocarbons) represent a significant part of the organic matter in marine waters, and their degradation has an important impact in the carbon fluxes within oceans. However, because they are nearly insoluble in the water phase, their degradation by microorganisms occurs at the interface with water and thus requires specific adaptations such as biofilm formation. We show that Marinobacter hydrocarbonoclasticusSP17 develops biofilms, referred to as oleolytic biofilms, on a large variety of hydrophobic substrates, including hydrocarbons, fatty alcohols, fatty acids, triglycerides, and wax esters. Microarray analysis revealed that biofilm growth on n-hexadecane or triolein involved distinct genetic responses, together with a core of common genes that might concern general mechanisms of biofilm formation. Biofilm growth on triolein modulated the expression of hundreds of genes in comparison with n-hexadecane. The processes related to primary metabolism and genetic information processing were downregulated. Most of the genes that were overexpressed on triolein had unknown functions. Surprisingly, their genome localization was restricted to a few regions identified as putative genomic islands or mobile elements. These results are discussed with regard to the adaptive responses triggered by M. hydrocarbonoclasticusSP17 to occupy a specific niche in marine ecosystems.
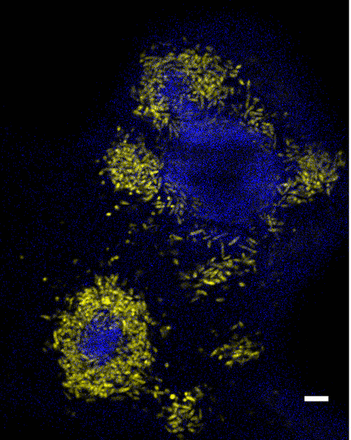
This paper describes the genetic study of a microbial biofilm dedicated to the degradation of a large panel of marine hydrophobic organic compounds in order to identify the biological processes involved in the recycling of the insoluble oily carbon in oceans.
Introduction
Hydrophobic organic compounds (HOCs), which include lipids and hydrocarbons, are ubiquitous in the marine environment. Their hydrophobic properties make them virtually absent in the water phase. They are instead represented in the particulate phase as living organisms, cell compartments such as membranes or carbon storage bodies, and nonaqueous liquids, adsorbed onto inorganic or organic surfaces or embedded in organic gels. Lipids account for c. 15% of the organic matter in the euphotic zone of the equatorial oceans (Wakeham et al., 1997). They are among the most labile organic compounds in oceans because they are almost completely degraded in the upper 100 m, thus allowing some bacteria to degrade them efficiently (Wakeham et al., 1997; Yoshimura & Hama, 2012). Considering that microorganisms are believed to take up substrates only as water-dissolved molecules, this suggests that some heterotrophic bacteria may have developed efficient strategies to adhere to these hydrophobic interfaces and to solubilize and transport hydrophobic substrates, making them more bioavailable (Harms et al., 2010a).
To date, the characterization of marine bacterial species specialized in the degradation of HOCs has mostly been focused on hydrocarbon-degrading capabilities as they respond to social concerns toward the pollution of marine environments. These studies have focused on bacterial species that are highly specialized in hydrocarbon degradation, referred to as marine hydrocarbonoclastic bacteria (Yakimov et al., 2007). These bacteria belong to only a few genera such as Alcanivorax, Cycloclasticus, Oleiphilus, Oleispira, and Thallassolituus. However, their high substrate specificity most likely excludes them from being involved in the recycling of lipids produced in the euphotic zone of oceans.
In contrast to terrestrial hydrocarbon degraders, which tend to be metabolically versatile (Mara et al., 2012), descriptions of marine bacteria that are able to degrade a wide range of lipids and hydrocarbons are very rare in the literature (Golyshin et al., 2002; Klein et al., 2008; Tanaka et al., 2010). Marinobacter hydrocarbonoclasticus SP17 was initially described as an hydrocarbonoclastic bacterium isolated from an oil-contaminated marine sediment (Gauthier et al., 1992). This bacterium was further shown to form biofilms at the interface between water and n-alkanes (C8 to C28) or metabolizable n-alcohols (C12 and C16) (Klein et al., 2008; Vaysse et al., 2009; Grimaud et al., 2012). Marinobacter hydrocarbonoclasticus SP17 forms biofilms only on hydrophobic substrates that it can metabolize. No biofilm was observed on nonmetabolizable HOCs such as branched alkanes (Klein et al., 2008). This substrate specificity suggests that biofilm formation is determined by the presence of a nutritive interface. Furthermore, the rate of n-hexadecane degradation decreased dramatically when the biofilm was disorganized, thus confirming that biofilm formation may constitute an efficient adaptive strategy for assimilating alkanes (Klein et al., 2008). Such biofilms, which have been observed with many strains or consortia degrading aliphatic or aromatic hydrocarbons, are thought to provide bacteria with efficient mechanisms to access hydrocarbons. In a few studies, they have been shown to increase the rate of mass transfer of hydrocarbons by reducing the diffusion path of the substrate (Golyshin et al., 2002; Johnsen et al., 2005; Grimaud, 2010; Harms et al., 2010b; Jiménez et al., 2011; Jung et al., 2011; Notomista et al., 2011; Tribelli et al., 2012). A proteomic study was conducted on M. hydrocarbonoclasticus SP17 biofilm growing on n-hexadecane (Vaysse et al., 2009). It revealed that biofilm cells expressed a specific proteome in which 50% of the detected proteins had their quantity levels altered when compared to planktonic cells growing exponentially on acetate. The adaptation to alkane utilization as a carbon and energy source therefore involves a global change in cell physiology.
We conducted a phenotypic study, and the findings indicate that M. hydrocarbonoclasticus SP17 can grow a biofilm on a larger variety of HOCs than suspected in previous studies. This result makes M. hydrocarbonoclasticus SP17 one of the first marine bacterial strains described thus far that degrades both hydrocarbons and lipids. To obtain a more comprehensive picture of biofilm development on diverse HOCs, we compared whole-genome transcriptomic data obtained from M. hydrocarbonoclasticus SP17 biofilms growing on n-hexadecane or on triolein and from planktonic cells growing exponentially on acetate. Gene expression patterns confirmed that biofilm growth on each HOC involved distinct genetic responses together with a core of common genes that might concern general mechanisms of biofilm formation. Taken together, the results presented in this study shed light on bacterial cellular processes that may play a significant role in the recycling of a large fraction of the organic matter in the oceans.
Materials and methods
Bacterial strains and growth assays
The Marinobacter hydrocarbonoclasticus SP17 (ATCC 49840) strain was initially described by Gauthier et al. (1992). Marinobacter hydrocarbonoclasticus SP17 was cultivated in synthetic seawater (SSW) supplemented with 20 mM Na acetate (further referred to as SSW acetate) as described by Klein et al. (2008). The M. hydrocarbonoclasticus MJ6-1 fluorescent strain derives from the SP17 strain. It was obtained by transformation of the spontaneous streptomycin-resistant JM1 strain (rpsLK58T, SmR) with the pUC18T-mini-Tn7T-Tp-eyfp plasmid (Choi & Schweizer, 2006; Mounier, 2013). The mini-Tn7 transposon integrated at the single Tn7 integration site of the M. hydrocarbonoclasticus SP17 genome (Grimaud et al., 2012). This transposon expresses the eYFP fluorescent protein due to the modified E. coli lac operon promoter PA1/04/03.
Biofilm growth on the HOC substrates that are solid at 30 °C was tested in 24-well polystyrene plates (Evergreen Scientific). All the chemicals were purchased from Sigma-Aldrich. Approximately 0.2 g of solid HOC were melted for 1 h at 90 °C in wells and cooled at room temperature. Exponentially growing cells in SSW acetate were harvested by centrifugation at 10 000 g for 15 min at room temperature and suspended to a final OD600 nm of 0.1 in SSW medium. In total, 1.5 mL of the cell suspension was added to each HOC-coated well and incubated at 30 °C at 100 r.p.m. A no-cell control was also performed with sterile SSW medium for each HOC. After 24 h of cultivation, the culture medium containing the planktonic cells was gently sucked out. Cells adhered to the solid substrate were stained for 3 min with 0.4 mL of crystal violet 1% (w/v). In these conditions, the adhesion of the cells to the polystyrene walls in comparison with the HOC substrates was negligible. After two washes with MilliQ water, crystal violet was extracted with 0.75 mL of acetic acid 10% (v/v)–ethanol 50% (v/v), and the absorbance was read at 595 nm. OD595 nm from the no-cell controls was subtracted. All the measurements were performed in triplicate.
Biofilm growth on the HOC substrates that are liquid at 30 °C was tested in 50-mL glass serum flasks at 30 °C at 50 r.p.m. (Klein et al., 2008). The inoculation was carried out with 10 mL of the same cell suspension as described above. Each liquid HOC was then added at a final concentration of 0.2% (v/v). After 24 h of cultivation, the biofilms that formed at the water–HOC interfaces were retained on nylon membranes with a porosity of 33 μm (Nitex®), stained for 3 min with 0.3 mL of crystal violet 1% (w/v) and washed twice with 10 mL of deionized water. Crystal violet was then extracted as described above, and the OD595 nm was determined. All the measurements were performed in triplicate.
Microscopy and imaging
Microscopic observations of M. hydrocarbonoclasticus biofilm cells were performed on the MJ6-1 strain grown in the same conditions as those used to prepare RNAs for transcriptomic analyses, except that pyrene (Sigma-Aldrich) was dissolved at 6 mg mL−1 in n-hexadecane or triolein prior to inoculation. Biofilm samples were mounted on glass slides, and observations were made using an Axio Observer.Z1 inverted microscope (Zeiss, Germany) equipped with a 63× (Plan APO, N.A. 1.4, M 27) oil immersion lens. Differential interference contrast (DIC) observations were performed with the Zeiss PA 63×/1.4 HR III optical component.
Epifluorescence microscopy was performed either by standard optical treatment or with the Zeiss ApoTome.2 attachment. Triolein or n-hexadecane particles stained with pyrene were visualized using epifluorescence illumination (excitation filter G365, Beamsplitter FT 395, emission BP 445/50). eYFP fluorescence was revealed with a BP 470/40 excitation filter and a Beamsplitter FT 495 and observed through a 445/50 emission filter. Images were acquired using a CCD Zeiss Axiocam 506 mono camera monitored by the zeiss zen 2012 software. Image treatments were performed with imagej software (http://imagej.nih.gov/ij/).
RNA preparation, cDNA synthesis and labeling
Exponentially growing planktonic cells or biofilm samples were prepared as previously described (Vaysse et al., 2009). Total RNA from 50 mL of planktonic exponentially growing cells (OD600 nmc. 0.4) on SSW 20 mM acetate or from biofilm cells from 300 mL-biofilm cultures in 500-mL Erlenmeyer flasks were isolated using the Extract-All kit (Eurobio). RNA was extracted in triplicate from independent biological samples. Remaining traces of DNA were removed with DNase I (Invitrogen), and DNase-treated RNA was immediately processed on RNA Easy Clean Up columns (Qiagen). RNA quality and quantity were checked with an RNA 600 Nano kit on a BioAnalyser 2100 (Agilent) and a NanoDrop ND-1000 Spectrophotometer (Labtech). Five μg of total RNA was reverse-transcribed using Superscript III enzyme and the first-strand cDNA synthesis kit (Invitrogen) using random hexamers. cDNAs were eventually purified using the MinElute Reaction Cleanup kit (Qiagen). cDNA quality and quantity were checked as described for RNA. Cy3 labeling of cDNAs was performed with the One-Color DNA Labeling Kit (Roche NimbleGen) using Cy3-random nonamers. Quantities of Cy3-labeled cDNA were estimated at 240 nm.
M. hydrocarbonoclasticus SP17 whole-genome microarray design, hybridization and image acquisition
The assembled whole-genome sequence of M. hydrocarbonoclasticus SP17 was shared into 59 noncoding RNA sequences (tRNAs and rRNAs), 1739 intergenic regions longer than 60 bp and 3807 coding sequences (Grimaud et al., 2012). Probe design and microarray printing were performed by Roche NimbleGen. Six 45-60-mer oligonucleotides were designed for each of these genomic objects, three of them being in the forward direction and accompanied by their reverse complement (reverse strand). The oligonucleotides were chosen to span the 5′ and 3′ ends and an intermediate position. There were 182 genomic objects shorter than 300 bases for which only one or two probes could be selected (two or four probes if both strands are counted). Twenty-two groups of sequences sharing 100% of identity were represented by the same set of probes, and three genes were not represented. All probes were synthesized in duplicate together with 6186 random sequences with no similarity to any M. hydrocarbonoclasticus SP17 sequence, leading to 72 546 features per hybridization zone. The array design is available in the ArrayExpress database (www.ebi.ac.uk/arrayexpress) under accession number A-MEXP-2398.
Marinobacter hydrocarbonoclasticus SP17 arrays were provided under the Roche NimbleGen 4x72k format. The cDNA labeling, microarray hybridization, and scanning were all performed at the BioChip Platform (Toulouse, France) following the specifications provided in the NimbleGen Arrays User's Guide ‘Gene Expression Analysis’. A NimbleGen Mixer was adhered to the 4x72k slides, and the Cy3-labeled cDNAs were loaded for hybridization onto the microarrays using the NimbleGen Hybridization System. The scanning of the 4x72k microarrays was performed on a Roche MS200 scanner using the nimblescan software v2.6 protocol. The individual slide images were separated for further processing.
Data processing and statistical analysis
Three arrays hybridized from three independent biological samples generated a total of 36 raw values for each genomic object in each growth condition. The raw data are available in the ArrayExpress database under accession number E-MTAB-2593. Raw values obtained from intergenic sequences were not kept in the following analyses. Transcriptomic results were processed using the ANAIS software as follows (Simon & Biot, 2010). Array quality was assessed at the probe level. Robust multi-array analysis background normalization and quantile normalization were performed for intra- and interarray normalization, respectively (Bolstad et al., 2003; Irizarry et al., 2003). Gene expression values were summarized by median polish of the corresponding normalized probe set values. A total of 3839 genes with signal intensities above a 95% random threshold were kept for further studies. Gene differential expression is given as standard fold-change, which was calculated as the ratio between the normalized gene expression value for each experimental condition and the referent experimental condition. Significant fold-changes were statistically determined using an anova-FDR adjusted P ≤ 0.05 (Benjamini & Hochberg, 1995).
The ‘triangle plot’ representation (Fig. 3) of expression ratios reduced three-valued expression points (A, B, C) to two dimensions by plotting A/2 + B vs. A/(A + B + C). Location in the plot is based on proportionality among expression levels, ignoring magnitude. Genes whose expression was high in one condition and low in the other two lie near a specific corner of the triangle, genes that were high in two conditions and low in the third are plotted along one edge of the triangle, and genes that were roughly equally expressed in all conditions fall toward the center. All analyses were performed in the R environment (http://www.r-project.org/) with the ade4 package for construction of the triangle plots.
Sequence analysis and data mining
Biological interpretation of the transcriptomic datasets at the biological process level was carried out by mapping genes of the different expression categories in the KEGG pathway database (Kanehisa et al., 2013). Lists of genes were downloaded in the ‘search&color’ advanced mapping tool (http://www.genome.jp/kegg/tool/map_pathway1.html) and compared to the KEGG annotation of the M. hydrocarbonoclasticus SP17 genome available in the database.
Whole-genome alignments were performed with the mauve 2.3.1 Java application using M. hydrocarbonoclasticus SP17 (GenBank ID: FO203363) and VT8 (formerly M. aquaolei VT8, (Márquez & Ventosa, 2005) (GenBank ID: CP000514.1) full genome sequences downloaded from the NCBI Microbial Genomes database (http://www.ncbi.nlm.nih.gov/genome) (Darling et al., 2004). Alignments were performed using default settings. The putative genomic islands in M. hydrocarbonoclasticus SP17 genome were predicted by an integrated method using the islandviewer program suite (Dhillon et al., 2013). The results were visualized with the dnaplotter Java application of the artemis genome viewer software (Carver et al., 2009). The results were exported as.svg files which were further manipulated in inkscape 0.48.4 (http://inkscape.org) to generate Figs 7 and 8.
Results and discussion
Marinobacter hydrocarbonoclasticus SP17 forms oleolytic biofilms on various hydrophobic organic compounds
Marinobacter hydrocarbonoclasticus SP17 was previously shown to be able to degrade a wide range of n-alkanes (C8 to C28) and two n-alkanols (C12 and C16) through the formation of a biofilm at the HOC–water interface (Klein et al., 2008). To further define the substrate range of this strain, biofilm formation was tested on a wider range of HOCs provided as the sole source of carbon and energy (Table 1; Fig. 1). Biofilm growth was observed either on liquid or solid, saturated or unsaturated aliphatic compounds such as medium-chain alkanes, alkenes, fatty alcohols and acids, triglycerides, or wax esters. Although M. hydrocarbonoclasticus SP17 was initially recognized as a marine hydrocarbonoclastic bacterium and thus considered to play an important role in hydrocarbon degradation (Gauthier et al., 1992; Yakimov et al., 2007), its wide metabolic capacity indicates that it should instead be considered as a versatile degrader of oily organic substrates. As such, we propose to refer to M. hydrocarbonoclasticus SP17 biofilm as an oleolytic biofilm. Its ability to grow as a biofilm on a wide range of poorly soluble HOCs makes this bacterium well adapted to the recycling of particulate hydrophobic organic carbon in the ocean (Volkman & Tanoue, 2002; Azam & Malfatti, 2007).
Biofilm growth ability of M. hydrocarbonoclasticus SP17 on various hydrophobic organic compounds
Percentages are in v/v.
Biofilm growth relates to crystal violet staining intensity (OD595 nm; see Materials and methods).
Biofilm growth ability of M. hydrocarbonoclasticus SP17 on various hydrophobic organic compounds
Percentages are in v/v.
Biofilm growth relates to crystal violet staining intensity (OD595 nm; see Materials and methods).
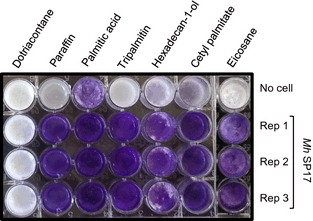
Biofilm growth phenotype of Marinobacter hydrocarbonoclasticusSP17 on various solid HOCs. Biofilm formation was estimated by crystal violet staining after 24 h of growth on a medium-chain (eicosane, C20) or a long-chain alkane (dotriacontane, C32), on a mix of medium- and long-chain alkanes (paraffin, C19 to C31), on a saturated fatty acid (palmitic acid, C16), on a saturated fatty alcohol (hexadecan-1-ol, C16), on a saturated triglyceride (tripalmitin), and on a wax ester (cetyl palmitate, C32). Growth tests were performed in triplicate (rep 1-3). A no-cell control that was initially inoculated with sterile SSW medium shows reference crystal violet staining.
Biofilm formation on n-hexadecane or triolein involves different genetic programs
Considering the variety of the physical and chemical natures of the HOC–water interfaces listed in Table 1, we were interested in whether specific cellular processes are involved in the recognition of the nutritive status of the interface, the adhesion to the interface, and the catabolic pathways that sustain substrate assimilation and biofilm growth on the different HOCs. A transcriptomic study using a microarray chip carrying the whole genome of M. hydrocarbonoclasticus SP17 was performed to identify genes regulated in response to biofilm formation on the alkane n-hexadecane and the unsaturated triglyceride triolein. These two compounds were chosen because they are representative of two substrate classes that M. hydrocarbonoclasticus SP17 may degrade in the environment, that is hydrocarbons of anthropogenic or biological origin and biogenic lipids. The expression profiles of the whole set of M. hydrocarbonoclasticus SP17 genes were measured in cells from 24-h biofilms formed at the water–HOC interfaces and from planktonic cells growing exponentially on acetate. Microscopic observations on samples obtained with M. hydrocarbonoclasticus MJ6-1 YFP-tagged cells grown in the same conditions confirmed that mature biofilms were produced onto both types of HOCs in these conditions (Fig. 2). Gene expression levels in these three conditions were then compared to each other (Supporting Information, Table S1).

Microscopy of Marinobacter hydrocarbonoclasticus biofilms on n-hexadecane and triolein. Marinobacter hydrocarbonoclasticus SP17-derived MJ6-1 strain expressing eYFP was grown for 24 h in SSW medium supplemented with n-hexadecane (a, b) or triolein (c, d) labeled with pyrene. (a) and (c) differential interference contrast (DIC) images of one focus plane showing mature biofilms at the water–HOC interface. (b) Epifluorescence microscopy of the same focus plane observed in (a) with bacterial cells expressing eYFP (yellow fluorescence) onto n-hexadecane (blue fluorescence). (d) Structured-illumination epifluorescence microscopy (ApoTome) of the same focus plane observed in (c) with bacterial cells expressing eYFP (yellow fluorescence) onto triolein (blue fluorescence).
A total of 1222 genes were identified as differentially expressed in at least one biofilm condition, that is on either n-hexadecane or triolein interface, in comparison with planktonic exponential growth on acetate (significant FDR(BH) P < 0.05) (Table S2). The range of differential expression ratios between n-hexadecane or triolein and acetate conditions varied from −6.21 (MARHY3166; conserved hypothetical protein) to +4.61 (MARHY2837; Ferredoxin) and from −7.05 (MARHY3166) to +2.48 (MARHY1868; hypothetical protein), respectively. This relatively low range of differential expression was expected as biofilms are known to gather cells of different physiological states that might correlate with distinct transcriptome profiles. One gene that is highly differentially expressed in one particular cell type should therefore see its expression signal lowered by the RNA produced in the other cell types. Nevertheless, the MARHY3166 gene showed the strongest downexpression on both HOCs, thus indicating that its still unknown function is most likely rather necessary for planktonic growth than for biofilm growth.
Three groups of genes comprising six expression categories were defined according to relative gene expression levels between biofilm growth conditions on HOCs and planktonic growth on acetate (Fig. 3a; Table S2): the BF HEX group contained the genes that were overexpressed (HEX+ category, 66 genes) or downexpressed (HEX− category, 119 genes) only in biofilms on n-hexadecane, the BF TRI group contained the genes that were overexpressed (TRI+, 442 genes) or downexpressed (TRI−, 409 genes) only in biofilms on triolein, and the BF COM group contained the genes that were overexpressed (HEX+TRI+, 59 genes) or downexpressed (HEX−TRI−, 127 genes) in biofilm conditions on both n-hexadecane and triolein. These results also show that biofilm growth on each HOC involved distinct genetic responses together with a core of common genes that might play a role in the general mechanism of biofilm formation on any HOC. One striking result is the large difference in the number of affected genes between the two HOC substrates. Although being assimilated by M. hydrocarbonoclasticus SP17 through a common biofilm lifestyle, to grow as a biofilm on n-hexadecane seemed to require less genetic changes than on triolein when compared to exponential growth on acetate. Hexadecane may present bacterial cells with an interface of different physicochemical properties or nutritive status in comparison with triolein. Another explanation could be associated with the numerous sources of triglycerides that exist in the marine environment where they can be found, for example in cell debris or as oil bodies, organelles or membranes in living vegetal, and animal or prokaryotic cells. One could therefore consider that forming a biofilm on triolein may signify for the bacterial cells to modulate a larger panel of biological processes.
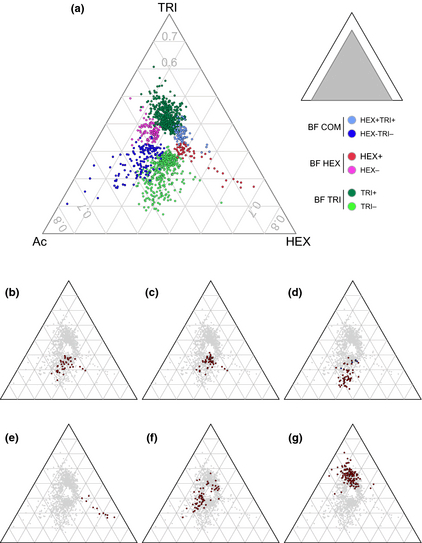
‘Triangle plot’ of gene relative expression among the three culture conditions. Every gene is represented by a point whose proximity to each of the substrate-labeled corners reflects the relative expression in that growth condition (see ‘Materials and Methods’ for the numerical formula). Genes expressed in a single condition lie close to the corner, whereas those expressed equally in two conditions vs. the third lie close to the triangle sides. Genes not significantly biased in any pair-wise comparison (P > 0.05) are not shown. The small gray triangle shows the position of the main triangle plot in a 1 × 1 × 1 side triangle. (a) Genes of the HEX+, HEX−, TRI+, TRI−, HEX+TRI+, and HEX−TRI− expression categories are highlighted as colored open circles (a). (b–d) Triangles highlight genes of the TRI+ category mapped in the KEGG PATHWAY database as involved in carbohydrate metabolism, amino acid metabolism, and translation, respectively. Genes of the last category were shared between those coding ribosomal proteins (red dots) and those involved in other translation mechanisms (blue dots). (e) Key genes of the HEX+ category. f) Genes annotated as involved in chemotaxis and motility. (g) Genes of the 2-Mb region.
Biofilm growth on triolein strongly reduces primary metabolism and genetic information processing
Considering the large differences in gene number observed between the BF TRI and BF HEX groups, we were interested in whether these differences could correspond to the modulation of specific biological processes. The 1222 genes of the BF TRI, BF HEX, and BF COM groups were mapped into the KEGG PATHWAY resource using the annotated M. hydrocarbonoclasticus SP17 genome provided in the KEGG genome database (Kanehisa et al., 2013). Only 43 BF HEX, 48 BF COM, and 258 BF TRI genes could be mapped; the remaining genes showed no significant sequence similarity to the genes mapped in the KEGG PATHWAY database (Table S3). The KEGG PATHWAY module generated a list of 700 annotation terms related to biological processes. This number was higher than the number of genes, as many of them were annotated as involved in several biological processes.
We classified these 700 annotation terms under the limited number of the main biological processes of the KEGG PATHWAY map and represented them in association with the expression profiles of the corresponding genes (Fig. 4). The main result that emerged from this analysis is that the TRI− category provided a high proportion of the KEGG annotation terms. Half of the TRI− genes (206 out of 409) were annotated by the KEGG module, whereas 387 of the 442 TRI+ genes (86%) were not mapped. This high proportion of genes with a remaining unknown function suggests that biofilm growth on triolein involves biological processes that still require characterization.
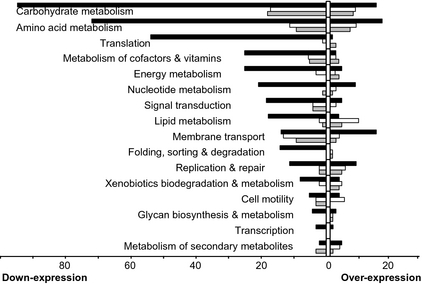
Classification and occurrence of the KEGG terms annotating the genes differentially expressed in biofilms on n-hexadecane and triolein. The annotation terms obtained from the mapping of the genes of the BF HEX (gray box), and the BF COM (open box) and BF TRI (black box) groups in the KEGG pathway/genome database were classified according to their expression profiles (down- or overexpressed). Numbers refer to the number of KEGG annotation terms in each category.
The biological processes whose gene expression seemed to decrease significantly in response to biofilm growth on triolein were carbon and energy metabolism and genetic information processing (Fig. 4; Table S3). More particularly, terms related to carbohydrate metabolism, fatty acid biosynthesis and degradation, nucleotide and amino acid metabolism, and translation were overrepresented in the annotated downexpressed genes (Figs 3b–d and 4). A schematic mapping of the genes involved in carbohydrate metabolism shows that many of the genes involved in glycolysis, fermentation, the tricarboxylic cycle, and the glyoxylate shunt were downexpressed with ratios ranging from −1.39 to −3.88 (Fig. 5). Moreover, oxidative phosphorylation was also affected with six genes of the ATP synthase cluster (MARHY3830-3835; −2.67, −2.43, −2.47, −1.98, −2.07 and −2), the cytochrome c reductase (MARHY0774-0776; −2.11, −2.68 and −2.75), and the cytochrome oxidase complex (MARHY0053, MARHY1519-1521; −1.45, −2.87, −2.65, −2.93). The downexpression of genes involved in amino acid metabolism was consistent with those of the carbohydrate metabolic pathway, as these two metabolisms are directly linked. Amino acid precursors are intermediates of the carbohydrate metabolism. Furthermore, 45 ribosomal subunit protein genes (21 small subunit genes and 24 large subunit genes) were also significantly downexpressed, together with genes involved in transcription, translation, and protein fate. Their downexpression ratios were the largest of this expression category (Fig. 3d; Table S2). Taken together, these results might signify that a major part of the cells enclosed in biofilm on triolein was less metabolically active than those on n-hexadecane or growing exponentially on acetate.
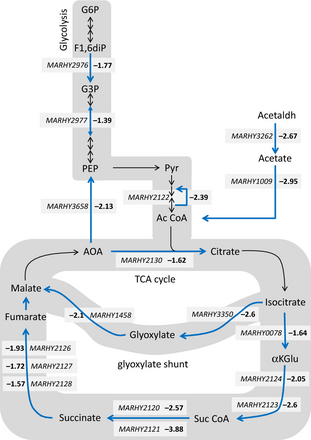
Representation of the carbohydrate metabolism response of Marinobacter hydrocarbonoclasticusSP17 biofilm on triolein. The figure summarizes the KEGG PATHWAY mapping of the genes of the TRI− expression category annotated as involved in glycolysis, fermentation, the tricarboxylic acid cycle, and the glyoxylate shunt. Gene names and TRI vs. AC ratios are indicated for genes showing significant differential expression (P ≤ 0.05). The MARHY2977 gene is also shown although its differential expression ratio is low. Not only its fold-change was statistically significant, but it also forms a gene cluster with MARHY2976, which is involved in the previous metabolic step and was similarly downexpressed on triolein. Only key metabolites are indicated. G6P, glucose-6-phosphate; F1,6diP, fructose-1,6-biphosphate; G3P, glyceraldehyde-3-phosphate; PEP, phosphoenol-pyruvate; Pyr, pyruvate; Ac CoA, acetyl CoA; Acetaldh, acetaldehyde; AOA, oxaloacetate; αKGlu, alpha-ketoglutarate; Suc CoA, succinyl CoA; TCA, tricarboxylic acid.
Gene expression in biofilms on n-hexadecane confirmed a role for proteins involved in alkane assimilation and the type VI secretion system
Genes involved in the primary steps of alkane oxidation provide one example of substrate-specific genes that are overexpressed only in biofilms on n-hexadecane. One gene encoding a terminal-1-alkane monooxygenase (MARHY2735) homologous to AlkM and a cluster of three genes expressing a cytochrome P450 alkane hydroxylase (MARHY2838, homologous to AhpG2), an alcohol dehydrogenase homologous to AlkJ (MARHY2839), and a ferredoxin (MARHY2837) were among the most overexpressed genes in this condition (HEX vs. AC expression ratios of +3.51, +3.26, +2.51 and +4.61, respectively), with no significant differential expression on triolein in comparison with acetate (Fig. 3e; Table S2). Consistent with gene expression studies carried out on alkane biodegradation pathways in bacteria, this induction of alkane oxidation genes was expected (Rojo, 2009; Sabirova et al., 2011). By contrast, MARHY3758, which is orthologous to almA, a flavin-binding monooxygenase gene involved in long-chain hydrocarbon (> 32 carbons) degradation in Acinetobacter and Alcanivorax species, showed no change in expression (Wang & Shao, 2012). This specific expression of medium-chain hydrocarbon oxidizing enzymes in contrast to the long-chain degrading enzyme AlmA confirms that the transcriptomic results are relevant to the experimental culture conditions.
Interestingly, other genes belonging to this HEX+ category also confirmed notable results obtained from a previous proteomic study carried out on M. hydrocarbonoclasticus SP17 biofilms grown on n-hexadecane (Vaysse et al., 2009). For instance, five genes, which showed specific and among the most important overexpression on n-hexadecane, produced proteins that had been described as being among the most abundant proteins detected in biofilms on n-hexadecane (Fig. 3e, Table S2). The MARHY2686 gene (HEX vs. AC ratio of +3.37) encodes a protein that was the most abundant in this condition. Interestingly, it forms a cluster with two other genes (MARHY2685 and MARHY2687) that showed the same specific HEX+ expression pattern (+1.8 and +2.6, respectively) (Table S2). The MARHRY3686 gene codes for a putative efflux pump. The four other genes are MARHY3634 (+3.05) and MARHY3635 (+2.19), two members of a type VI secretion system (T6SS) gene cluster, and MARHY0478 (+2.04) and MARHY0477 (+1.57), which present reduced alkane assimilation when mutated (Fig. 3e) (Mounier, 2013). Such a consistent pattern of expression from two independent experimental strategies together with mutant phenotypes reinforces the probability for a role of these proteins in biofilm growth on n-hexadecane.
Chemotaxis and motility genes are downregulated in biofilm conditions
Among the 133 genes annotated as being involved in chemotaxis and motility, 62 were differentially expressed in biofilm conditions with 73% of them being downregulated (Fig. 3f; Table S4). The main processes that were affected concerned the chemosensory signaling pathways, type IV and MSHA pili formation, and, to a lesser extent, flagellar assembly (Fig. 6). Although the differential expression ratios were approximately twofold, the high proportion of genes differentially expressed in this particular biological process highlights its putative role in biofilm lifestyle on HOCs. As discussed above, gene expression ratios determined from whole mature biofilms are expected to be lower than their actual levels in individual biofilm cells as biofilms comprise cells in different physiological states and with different transcriptomes. Nonetheless, the flagellin gene fliC (MARHY2517; HEX vs. AC ratio = −2.15, TRI vs. AC ratio = −2.27), the pilin gene pilA (MARHY2564; −2.3 and −3.37) as well as pilJ (MARHY3674; −1.69 and −2.38) and pilQ (MARHY0701; −1.63 and −2.26) were among the most downexpressed genes of the HEX−TRI− expression category (Fig. 3f; Table S4).

Differential expression of the chemotaxis and motility genes in biofilms at HOC–water interfaces. (a) Differential expression of the chemosensory system. The regulation of the flagellum assembly and rotation and type IV pili function use similar molecular mechanisms. A histidine kinase (CheA, ChpA) is coupled to a methyl-accepting chemotaxis receptor (MCP, PilJ) by an adaptor protein (CheW, PilI). Upon receipt of a signal, the histidine kinases autophosphorylate and phosphate groups are transferred to response regulators (CheY and CheB; PilG and PilH). Gray triangles in boxes represent downexpression; upper left triangle, n-hexadecane condition; and bottom right triangle, triolein condition. (b) Differential expression of genes in clusters of type IV pili genes. Downexpressed genes are represented as gray open arrows.
Chemotaxis controls motility apparatuses such as pili or flagella, to migrate toward favorable environments or away from detrimental environments. It is mediated by chemoreceptors that sense chemoeffectors by a specific receptor–ligand interaction that in turn activates intracellular signaling cascades to control cell movements (Krell et al., 2011; Porter et al., 2011). The transcriptomic data show that the expression of a majority of the proteins of the chemosensory signaling cascades that control flagellum assembly and rotation (CheA/CheW) and pili-dependent twitching motility (ChpA/PilJ) was reduced during M. hydrocarbonoclasticus SP17 biofilm growth on HOCs (Fig. 6a).
Biofilm formation on HOCs also affected the expression of flagellum genes, although to a lesser extent than chemotaxis or pili genes. Indeed, even though more than 50 M. hydrocarbonoclasticus SP17 genes were annotated as being involved in flagellum assembly and rotation, only a putative fliD (MARHY2507) was specifically downexpressed in biofilms on n-hexadecane (HEX vs. AC ratio of −1.46). Eight genes, that is fliC (HEX vs. AC ratio of −2.15 and TRI vs. AC ratio of −2.27), fliE (−1.29 and −1.35), flgC (−1.79 and −1.85), flgD (−1.55 and −1.99), flgF (−1.45 and −1.26), motA (+1.26 and +1.32), flhB (+1.35 and +1.34), and a putative flgL (MARH2170; +1.33 and +1.42), were affected in their expression in biofilm conditions on both HOCs (BF COM group). Biofilm growth on triolein specifically modulated the expression of fliM (TRI vs. AC ratio of −1.24), fliQ (+1.27), flhF (−1.64), and fgtA (+1.26). Interestingly, all these genes correspond mainly to the late-expressed genes that are involved in the export and assembly of the rod, hook, and filament parts of the flagellum. Flagella have been involved in different steps of biofilm formation in many bacterial species such as cell swimming to attractive surfaces, cell sticking, and swarming on surfaces and cell detachment (Sauer et al., 2004; Conrad, 2012; Friedlander et al., 2013; Partridge & Harshey, 2013). Consistently, flagellum motility is regulated during biofilm formation (Guttenplan & Kearns, 2013). Flagella and chemotaxis have been shown to play a role in biofilm formation in Marinobacter adhaerens because the cheA, cheB, chpA, or chpB mutants are impaired in biofilm formation on abiotic surfaces (Sonnenschein et al., 2012). Moreover, we observed that M. adhaerens formed biofilms on paraffin, whereas the fliC and fliA flagella mutants did not (unpublished results).
Type IV pili genes form clusters that are conserved across Proteobacteria phylum at different loci (Pelicic, 2008). The mapping of expression data in four clusters of type IV pili genes of the M. hydrocarbonoclasticus SP17 genome showed that the downregulation of gene expression was not restricted to individual genes but rather concerned gene clusters, thus suggesting a coregulation process aimed at reducing the role of the entire pili-mediated process. In particular, two pili gene clusters, that is pilMNOPQ and pilVWXY1, were downexpressed in biofilms either on n-hexadecane or on triolein, with expression ratios ranging from −1.38 to −2.26 (Fig. 6b; Table S4). Biofilm growth seemed to mainly regulate pili assembly genes. Marinobacter hydrocarbonoclasticus SP17 biofilm cells are then expected to have a reduced amount of these two extracellular motility structures. Such a downexpression of pili biogenesis genes was also described in A. borkumensis growing on n-hexadecane (Sabirova et al., 2011), although it concerned only four genes. Nevertheless, our study and the large number of genes affected could confirm that type IV pili downexpression might be considered as a characteristic feature of marine hydrocarbonoclastic bacteria growing at water–HOC interfaces. However, considering the variety of pilin sequences and roles, it is difficult to yield a hypothesis on the exact significance of such a downexpression in mature biofilms on HOCs.
The Marinobacter hydrocarbonoclasticus SP17 genome also contains a characteristic single large cluster of genes encoding a mannose-sensitive hemagglutinin (MSHA) pilus, a type IV pilus found in Vibrio cholerae, and other environmental bacteria (Marsh & Taylor, 1999; Thormann et al., 2004; Boyd et al., 2008; Saville et al., 2010). This MSHA pilus system has been involved in the adhesion of cells and biofilm formation onto a variety of abiotic and biotic surfaces such as glass (Thormann et al., 2004), chitin (Shime-Hattori et al., 2006), the cellulose-containing surface of the green alga Ulva australis (Dalisay et al., 2006), and zooplankton (Chiavelli et al., 2001). It is therefore of interest to note that up to ten genes out of the 16 that form this cluster were downregulated in biofilm conditions, with differential expression ratios ranging from −1.32 to −1.82. For instance, mshA that encodes the major pilin subunit showed an HEX vs. AC ratio of −1.48 and a Tri vs. AC ratio of −1.82.
The role of MSHA and type IV pili in promoting adherence to surfaces, cell aggregation, and biofilm formation is well documented (Giltner et al., 2012). Our results show that they may also mediate biofilm formation at HOC–water interfaces. As a downexpression in biofilm conditions signifies that these genes are rather overexpressed in planktonic cells growing on acetate, one can consider that their functions are less necessary in biofilms. This could support the hypothesis that they might be involved in the detection of and the adhesion to HOC–water interfaces. The validation of this hypothesis would be of great interest, as it would yield potential targets for future studies on HOC–water interface colonization in association with carbon recycling in the marine environment or hydrocarbon bioremediation in polluted areas.
Biofilm growth on triolein induced the transcriptional activation of a putative 104-kb genomic island
All the differentially expressed genes were mapped onto the genome of M. hydrocarbonoclasticus SP17 in association with their expression profiles (Fig. 7). This graphical representation revealed that most of the genes that showed overexpression on triolein (TRI+, and to some extent, HEX− categories) clustered in eight regions of the genome. On the other hand, very few genes overexpressed on n-hexadecane mapped to these regions. In particular, it pointed to a 168-kb genomic region located approximately between positions 1 877 000 and 2 045 000 (from MARHY1803 to MARHY2007) (Grimaud et al., 2012), which will be further referred to as the 2-Mb region. In total, 123 out of the 130 genes that were differentially expressed in this 203-gene-long 2-Mb region belonged to the TRI+ category (Fig. 3g; Table S2). This high density of similarly expressed genes at the same locus suggested the presence of a putative genomic island (GI). GIs are large (usually > 8 kb) mobile genetic elements that are horizontally transferred between bacteria. They generally convey genetic information that influences traits such as pathogenicity, symbiosis, resistance to antibiotics and toxic compounds, fitness, and adaptation (Dobrindt et al., 2004). The acquisition of GIs by bacteria is thought to provide genetic flexibility, particularly in environmental microorganisms that may be subjected to constant environmental changes. A recent study roughly estimated that more than 90% of the marine bacteria across the four major prokaryotic taxa in the oceans carry GIs in their genomes (Fernández-Gómez et al., 2012).
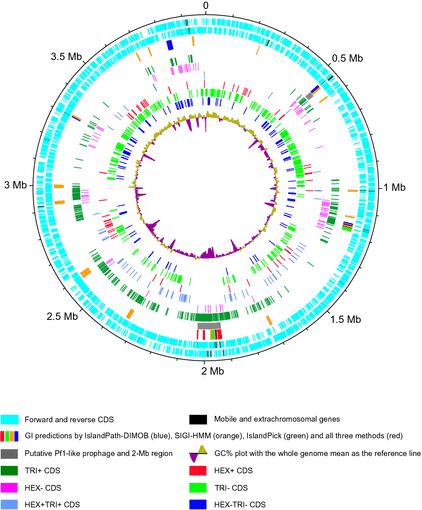
Mapping of the putative genetic features and the differentially expressed genes onto the Marinobacter hydrocarbonoclasticusSP17 genome. The M. hydrocarbonoclasticusSP17 genome map was generated with the dnaplotter Java application of the artemis genome viewer software. The tracks from the outside represent the scale in megabases; the forward coding and reverse coding sequences (CDS) with the CDS annotated as mobile and extra-chromosomal elements are in black; GI predictions by IslandPath-DIMOB (blue box), SIGI-HMM (orange box), IslandPick (green box), and all three methods (red box); the locations of a putative Pf1-like prophage (position 0.55 Mb) and the 2-Mb region (gray boxes); the genes of the TRI+, HEX−, HEX+TRI+, HEX+, TRI−, and HEX−TRI− categories, respectively, with same colors as in Fig. 3; and the %GC plot.
We first took advantage of the development of bioinformatic tools to test the presence of a GI in the 2-Mb region (Langille et al., 2010). Because bacteria gain a GI through horizontal gene transfer and can even lose it sporadically, the phyletic patterns of the GI and the host genomes may differ. We thus performed a genome comparative analysis between the M. hydrocarbonoclasticus SP17 and VT8 strains using the MAUVE whole-genome sequence alignment tool (Fig. 8) (Darling et al., 2004; Márquez & Ventosa, 2005). This comparison confirmed that a 104-kb-long region starting at position 1 927 665 (end of MARH1950) and ending at position 2 031 430 (between MARHY1992 and MARHY1993) was absent of the M. hydrocarbonoclasticus VT8 genome, whereas the whole core genome of M. hydrocarbonoclasticus SP17 was conserved (LCB weight of 7060). This region was also predicted as a putative GI by the IslandViewer tool, which combines three independent and accurate methods, that is IslandPath-Dimob, SIGI-HMM, and IslandPick; these methods are based on sequence composition and comparative-genome-based analyses (Fig. 7) (Dhillon et al., 2013). IslandViewer predicted the presence of 18 putative GIs in the M. hydrocarbonoclasticus SP17 genome (Table S5). In particular, it delineated three close putative GIs in the 2-Mb region, between positions 1 926 444 (MARHY1848) and 2 032 417 (MARHY1993) on the genome. A large part of this zone was identified by all three methods, thus reinforcing its prediction. As underlined by the IslandPath-DIMOB program, this region presented both a bias in GC% content (52.47%) in comparison with flanking genomic regions (57.43% and 56.69%; the mean genome GC% being 57.43%) and the presence of 10 genes of the ‘mobile and extrachromosomal elements’ COG annotation category, within which were four transposases, three putative integrases, one resolvase and one reverse transcriptase genes. Another interesting feature that is commonly associated with GIs is the high percentage of genes of unknown function, a percentage that is c. 55% in the 2-Mb region. This proportion is in accordance with percentages found in GIs in other marine bacteria (Fernández-Gómez et al., 2012). Together with other features such as the presence of a tRNA gene close to the GI insertion site (MARHYTRNA25-LEU at position 1 885 807, between MARHY1810 and MARHY1811) and flanking direct repeats (not shown), all these genomic data provide some confidence that this particular region is most likely a GI. Interestingly, M. hydrocarbonoclasticus VT8 strain, which lacks this 2-Mb GI, produced biofilms with twofold less biomass than SP17 strain on triolein (not shown), supporting a putative role for this 2-Mb region for biofilm growth on this substrate.
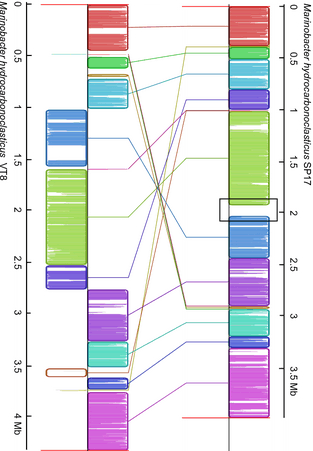
Genome alignment between Marinobacter hydrocarbonoclasticusSP17 and VT8 strains using the MAUVE algorithm. The MAUVE progressive alignments between M. hydrocarbonoclasticusSP17 and VT8 genomes defined locally collinear blocks of homologous regions. Homologous regions are indicated by similarly colored blocks and are connected by lines. The boundaries of colored blocks usually indicate the breakpoints of genome rearrangement, unless a sequence has been gained or lost in the breakpoint region. The black open box located around the 2-Mb position in the M. hydrocarbonoclasticusSP17 genome points to the putative 2-Mb GI region that has no homologous counterpart in the M. hydrocarbonoclasticusVT8 genome.
The relationship that exists between the overexpression of half of the genes of this 2-Mb region, other putative mobile elements, and biofilm growth on triolein is unclear, particularly as to whether this corresponds to the production of proteins involved in biofilm formation. This relationship is even more difficult to determine because 86 of the 137 differentially expressed genes have no known function. Furthermore, no clear bioprocess putatively involved in biofilm formation emerges from the gene annotation data, although functional categories seem conserved with other marine Gammaproteobacteria GIs (Fernández-Gómez et al., 2012). Nevertheless, a cluster of genes (MARHY1835 to MARH1843; TRI vs. AC ratios ranging from +1.28 to +1.58) is of particular interest as it concerns the metabolism of glycogen-like alpha-glucan polysaccharides. A similar cluster of genes in E. coli (glgBXCAP) together with glgS were involved in glycogen biosynthesis. Interestingly, E. coli ΔglgS mutant cells produced less glycogen, were hyperflagellated and hyperfimbriated, displayed elevated swarming motility, and displayed an increased ability to form biofilms on polystyrene surfaces, suggesting a link between glycogen production and biofilm formation (Rahimpour et al., 2013).
The overexpression of this 2-Mb region could point to a more general response that would link biofilm growth on triolein and transcriptional activation of mobile DNA elements. Indeed, in addition to this 2-Mb region, a careful analysis of the results presented in Fig. 7 shows that a systematic colocalization between all the IslandViewer-predicted GIs and genes overexpressed in biofilms on triolein appears to exist. Moreover, consistent with the expression profiles of the DNA mobility genes located within the 2-Mb region, 10 other genes of the same category dispersed within the core genome displayed the same overexpression on triolein (MARHY0506, MARHY0510, MARHY0965, MARHY1124 & MARHY1125, MARHY1129, MARHY1138 & MARHY1139, MARHY3218, and MARHY3796; TRI vs. AC expression ratios of +1.42, +1.54, +1.42, +1.41, +1.74, +1.99, +1.75, +1.44, +2.06, and +1.49, respectively). Among these are two prophage-like genes (MARHY0506 & MARHY0510) that are embedded in a putative Pf1-like prophage (Fig. 7). This could suggest a putative role for phage-mediated lysis in M. hydrocarbonoclasticus SP17 biofilm dynamics on HOCs. Such a role for prophages in biofilm formation has already been suggested in other environmental biofilm-forming bacteria. Cell lysis in Shewanella oneidensis MR-1 or in Streptococcus pneumoniae was shown to release biofilm-promoting factors such as e-DNA to mediate biofilm formation (Carrolo et al., 2010; Goedeke et al., 2011). The Pf4 phage is essential for several stages of the biofilm life cycle of Pseudomonas aeruginosa PAO1, particularly during the dispersal phase where it generates the typical hollow centers and cell detachment (Webb et al., 2003; Rice et al., 2008). The putative role of phage-mediated lysis during M. hydrocarbonoclasticus SP17 biofilm formation on HOCs is currently under investigation.
Conclusion
The wide range of oily organic substrates that are metabolized by M. hydrocarbonoclasticus SP17 defines it as an oleolytic bacterium. Because they are not soluble in water, oily organic compounds form interfaces onto which M. hydrocarbonoclasticus SP17 develops a biofilm that is necessary for their assimilation. The results obtained in this study show that M. hydrocarbonoclasticus SP17 oleolytic biofilms grown on n-hexadecane and triolein presented distinct gene expression profiles. The only well-identified biological process that they shared was the downexpression of the type IV and MSHA pili, indicating that pili-mediated motility might be less important for cells embedded in biofilms than for those growing planktonically. New transcriptome profiling experiments performed on other stages of biofilm growth, that is adhesion, microcolony formation, and cell detachment, will help to clarify pili role in the course of biofilm development. Biofilm growth on n-hexadecane modulated the expression of 185 genes in comparison with planktonic growth on acetate. The strongest overexpressions were measured for genes involved in the degradation of alkanes, as well as for an operon of two genes putatively involved in alkane assimilation. It also concerned a putative efflux pump and the type VI secretion system, thereby confirming results obtained from a previous proteomic study. Phenotypic analyses of mutant strains are currently underway to assess the role of these proteins in alkane degradation and the functioning of M. hydrocarbonoclasticus SP17 oleolytic biofilms. Surprisingly, biofilm growth on triolein involved the differential expression of many more genes than biofilm growth on n-hexadecane, with hundreds of genes being differentially expressed between these two biofilm conditions. Most of the differentially expressed genes with known functions described a general downexpression of the primary metabolism and translation machinery, suggesting a less active metabolic profile. A large majority of the genes that were overexpressed on triolein encoded putative proteins or had unknown functions, resulting in remaining questions about the biological processes involved in biofilm growth on this substrate. However, most of these genes clustered at a few loci that also systematically contained mobile and extrachromosomal elements. The largest locus was identified as a putative genomic island of c. 104 kb. Although we are still unable to draw a conclusion about the role of this GI, we could hypothesize that it is able to carry and transfer genes that are involved in biofilm growth on triolein. This GI is not present in the M. hydrocarbonoclasticus VT8 strain that formed less biofilm on triolein than SP17 (not shown), thus strengthening its putative role in this process. Genetic manipulations leading to its inactivation in M. hydrocarbonoclasticus SP17 and its transfer into VT8 genome will help to clarify its role.
We propose that M. hydrocarbonoclasticus SP17 is well adapted for the degradation of lipids and hydrocarbons of anthropogenic and biological origins found in marine environments. Similar metabolic profiling carried out on bacteria isolated from marine samples is now necessary to enlarge this group of marine oleolytic bacteria and to compare their metabolic properties to increase our knowledge of the overlooked process of hydrophobic carbon recycling in the oceans.
Acknowledgements
We are grateful to Lidwine Trouilh and Florence Hakil for their expert technical assistance in transcriptomics and molecular biology. We also thank Jean-Christophe Auguet for his very helpful expertise in R programing. J.M. was supported by a PhD fellowship from the French Ministère de l'Enseignement Supérieur et de la Recherche. This work was funded by the French national research agency (ANR) as part of the 2011 ‘AD'HOC’ project and the 6th European Framework Program, Contract 018391 FACEIT.
References
Author notes
Editor: Patricia Sobecky
French national research agency (ANR)
6th European Framework Program
018391 FACEIT