-
PDF
- Split View
-
Views
-
Cite
Cite
Vanessa Oliveira, Newton C.M. Gomes, Daniel F.R. Cleary, Adelaide Almeida, Artur M.S. Silva, Mário M.Q. Simões, Helena Silva, Ângela Cunha, Halophyte plant colonization as a driver of the composition of bacterial communities in salt marshes chronically exposed to oil hydrocarbons, FEMS Microbiology Ecology, Volume 90, Issue 3, December 2014, Pages 647–662, https://doi.org/10.1111/1574-6941.12425
- Share Icon Share
Abstract
In this study, two molecular techniques [denaturing gradient gel electrophoresis (DGGE) and barcoded pyrosequencing] were used to evaluate the composition of bacterial communities in salt marsh microhabitats [bulk sediment and sediment surrounding the roots (rhizosphere) of Halimione portulacoides and Sarcocornia perennis ssp. perennis] that have been differentially affected by oil hydrocarbon (OH) pollution. Both DGGE and pyrosequencing revealed that bacterial composition is structured by microhabitat. Rhizosphere sediment from both plant species revealed enrichment of operational taxonomic units closely related to Acidimicrobiales, Myxococcales and Sphingomonadales. The in silico metagenome analyses suggest that homologous genes related to OH degradation appeared to be more frequent in both plant rhizospheres than in bulk sediment. In summary, this study suggests that halophyte plant colonization is an important driver of hydrocarbonoclastic bacterial community composition in estuarine environments, which can be exploited for in situ phytoremediation of OH in salt marsh environments.
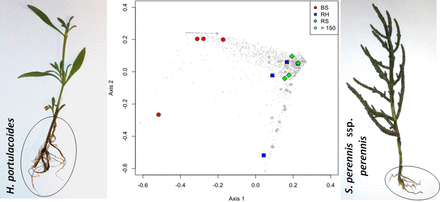
In-depth molecular characterization (DGGE and barcoded pyrosequencing) of bacterial communities in salt marsh sediments was used to provide a comprehensive overview of the rhizosphere bacterial communities associated with two important halophyte plants of a temperate estuary chronically exposed to oil hydrocarbon.
Introduction
Estuarine salt marshes are ecologically, commercially and economically important (Barbier et al., 2011). Due to their high productivity and location, they provide valuable ecosystem services (Coulon et al., 2012). However, being located between aquatic and terrestrial systems, they are vulnerable to perturbations from both environments (Bowen et al., 2012). Although salt marsh conservation is generally believed to be important for the maintenance of healthy coastal ecosystems, these ecosystems have been systematically destroyed due to urban and industrial growth (Reboreda & Cacador, 2007; Martins et al., 2008). Anthropogenic inputs of hydrocarbons are one of the major threats to salt marsh vegetation (Carman et al., 1996; Watts et al., 2006; Martins et al., 2008). Low-molecular-weight (LMW) aromatic hydrocarbons are particularly deleterious and can affect plants during all growth stages (Gao & Zhu, 2004; Watts et al., 2006, 2008).
Microbial degradation is the primary route for the breakdown of hydrocarbons; degradation depends largely on composition and adaptive response to the presence of hydrocarbons (Leahy & Colwell, 1990). The use of plants and their associated microorganisms to promote bioremediation of degraded areas has been previously demonstrated in several studies (Siciliano & Germida, 1998; Daane et al., 2001). Rhizosphere-associated microorganisms are currently regarded as pivotal in combating environmental contamination and there is growing interest in the development of new approaches for the mitigation of hydrocarbon contamination of coastal ecosystems (Daane et al., 2001; Ribeiro et al., 2011). Although information exists regarding the microbial processes involved in hydrocarbon degradation (McGenity, 2014) many others questions, such as the organization of microbial community structure or even the mechanism involved in their adaptation to the presence of oil contamination, need additional data to implement appropriate bioremediation strategies. Molecular techniques provide an opportunity to understand microbial diversity and functionality in oil-contaminated sites.
In a previous study, FISH was used to determine the relationship between different halophyte species and the relative abundance of prokaryote groups in salt marsh sediments and a metabolomics analysis revealed that volatile compounds released through the roots may underlie plant–bacteria associations in intertidal salt marshes (Oliveira et al., 2012). Here we provide, for the first time, a thorough in-depth molecular characterization of bacterial communities in bulk sediment and rhizosphere microhabitats in a temperate estuary (Ria de Aveiro, Portugal) chronically exposed to oil hydrocarbon (OH) pollution. Denaturing gradient gel electrophoresis (DGGE) and barcoded pyrosequencing (16S rRNA gene amplicons) were used to assess how plant species and OH pollution affect sediment bacterial composition in sampling sites exposed to different levels of contamination. In addition, the 16S rRNA gene sequences retrieved in this study were used to generate in silico metagenomes and evaluate the distribution of potential bacterial traits in different microhabitats.
Material and methods
Sampling sites and sample processing
Samples were obtained from four sites of the Ria de Aveiro estuarine system (Aveiro, Portugal). The Ria de Aveiro is a shallow estuary, sometimes considered a coastal lagoon, on the north-west coast of Portugal (40.7°N, 8.7°W). It is formed by a complex network of channels and extensive intertidal zones (Dias et al., 1999). Plant and sediment samples were taken at low tide, from four different sites (site A, 40°35′52.64″N, 8°45′0071″W; site B, 40°38′27.42′′N, 8°44′15.42′′W; site C, 40°37′32.18′′N, 8°44′09.12′′W; site D, 40°37′18.90′′N, 8°39′46.28′′W (Fig. 1). At each site, four composite samples of each plant species (Halimione portulacoides and Sarcocornia perennis ssp. perennis) were collected and stored separately in plastic bags for transportation. In the laboratory, bulk sediment and rhizosphere samples were separated. Roots were manually shaken to remove loosely bound sediment, which was discarded. The rhizosphere was extracted by suspending roots with tightly attached sediment particles in sterile deionized water. The mixture was kept in a rotary shaker for 30 min and the resulting sediment slurry was decanted into a sterile Falcon tube and centrifuged (5 min, 2468 g, 4°C). The supernatant was rejected (Phillips et al., 2008). Bulk sediment and rhizosphere extracts were stored at −20 °C for subsequent molecular analysis. For salt marsh physicochemical characterization, three sediment subsamples were collected from each sampling site.
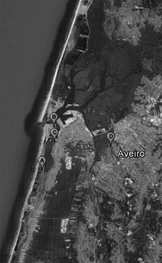
Ria de Aveiro (Portugal) with the location of sampling stations (A, B, C and D).
Sediment properties
For pH determination (Orion Model 290A), bulk sediment was suspended in water [1 : 5 (w/v)] (Faoun, 1984). Subsamples were analysed for organic matter content, as percentage of weight loss by ignition (8 h at 450 °C), moisture (percentage of water per quantity of fresh sediment weight) and sediment grain size (estimated from wet and dry sieving), following Quintino et al. (1989).
Hydrocarbon analysis
Bulk sediment was analysed for aliphatic and aromatic hydrocarbons after a Soxhlet extraction followed by GC-MS, conducted for three sediment subsamples of each site that were previously homogenized and freeze-dried. For standardization of the procedure, the internal calibration method was used in the quantification of 16 US Environmental Protection Agency priority polycyclic aromatic hydrocarbons (PAHs). Deuterated PAH surrogate standards were added to sediment samples to calculate the recovery efficiency during sample extraction, cleanup and analysis. For the aliphatic hydrocarbon fraction, two internal standards were used: undecane (C11H24) and tetracosane (C24H50).
Five grams of dried, homogenized sediment was extracted for 24 h in a Soxhlet apparatus with 150 mL of dichloromethane. Prior to extraction, the sediment was spiked with 25 μL (2 μg mL−1) deuterated surrogate standards (naphthalene-d8, acenapthlene-d10, phenanthrene-d10, chrysene-d12 and perylene-d12). Activated copper (Schubert et al., 1998) was added to the collection flask to avoid sulfur interference in GC-MS. The extracts were concentrated by rotary evaporation up to a volume of about 2–3 mL. The solvent was changed to 10 mL of hexane and the volume was further reduced to c. 1–2 mL. Hydrocarbons in the concentrated hexane extract were separated using a 2 : 1 alumina/silica gel column with anhydrous sodium sulfate overlaying the alumina in order to remove water. The column was used to clean-up and fractionate the extract. Elution was performed using 15 mL of hexane to obtain the first fraction (aliphatic hydrocarbons), followed by an elution with 30 mL of dicloromethane/hexane (1 : 1). These two eluents containing the PAHs were combined for analysis. The sample volume was reduced to 1 mL by rotary vacuum evaporator and further to 0.2 mL with a gentle pure nitrogen stream. A known quantity (2 mg mL−1) of the internal standard, hexamethylbenzene, was added prior to GC-MS.
GC-MS analyses were conducted in an Agilent Network GC system, namely an Agilent 6890 gas chromatograph equipped with a mass-selective detector (MSD 5973). The selective ion mode was used for aromatic hydrocarbons and the scan mode was used for aliphatic hydrocarbons. The hydrocarbons were separated using a VF-5MS fused silica column (30 m × 0.25 mm i.d. and 0.25 μm film thickness). GC-MS operating conditions were as follows: injector and transfer-line temperatures were maintained at 300 °C. The oven temperature program was initially isothermal at 60 °C for 1 min, increased to 200 °C at a rate of 10 °C min−1 (hold for 2 min), and then increased at a rate of 5 °C min−1 (hold 8 min) and kept isothermal at 300 °C. Helium was used as carrier gas, at a flow rate of 1.3 mL min−1. Aliquots of 1 μL were manually injected in the splitless mode with a 7.5-min solvent delay. For aromatic hydrocarbons, mass spectra were acquired at the electron impact (EI) mode at 70 eV. The mass scanning ranged between m/ᴢ20 and m/ᴢ500.
The surrogate recoveries added to sediment samples were 78 ± 18% for naphthalene-d8, 91 ± 19% for acenaphthene-d10, 114 ± 25% for phenanthrene-d10, 109 ± 24% for chrysene-d12 and 120 ± 20% for perylene-d10.
Total community DNA (TC-DNA) extraction
TC-DNA was extracted from bulk sediment and rhizosphere samples (0.5 g) with the MoBio Ultraclean soil DNA kit (Cambio) following the manufacturer's instructions.
PCR amplification of 16S rRNA gene fragments and DGGE
A nested PCR approach was used to amplify the 16S rRNA gene sequences from bulk and rhizosphere sediment (Gomes et al., 2008), which was more efficient for amplification of 16S rRNA gene fragments from sediment suitable for DGGE analyses. Briefly, in the first PCR the universal bacterial primers U27 and 1492R (Weisburg et al., 1991) were used. Reaction mixtures (25 μL) contained 12.5 μL DreamTaq PCR Master Mix (Fisher Scientific), 0.1 μM of each primer, 80 μg mL−1 bovine serum albumin and 1 μL of template DNA. The amplification conditions were as follows: 5 min of denaturation at 94 °C, and 25 cycles of 45 s at 94 °C, 45 s at 56 °C and 1.5 min at 72 °C; the PCR was finished by an extension step at 72 °C for 10 min. The amplicons obtained were used as template for a second PCR with the bacterial DGGE primers 984F-GC and 1378R (Heuer et al., 1997). The PCR reaction mixtures (25 μL) consisted of 12.5 μL DreamTaq PCR Master Mix (Fisher Scientific), 0.1 μM of each primer, 1% (v/v) dimethyl sulfoxide (DMSO) and 1 μL of template DNA. PCR amplification conditions were: initial denaturation (94 °C for 4 min); 30 cycles of denaturation (95 °C for 1 min), annealing (53 °C for 1 min) and extension (72 °C for 1.5 min); and a final extension (72 °C for 7 min). The GC-clamped amplicons were applied to a double-gradient polyacrylamide gel containing 6–10% acrylamide with a gradient of 40–58% of denaturants. The run was performed in Tris-acetate-EDTA buffer at 60 °C at a constant voltage of 80 V for 16 h using the DCode System (Universal Mutation Detection System, Bio-Rad). The DGGE gels were silver-stained (Heuer et al., 2001). Processing of the scanned DGGE gels was carried out using bionumerics software 6.6 (Applied Maths).
Barcoded pyrosequencing
A barcoded pyrosequencing approach was used for the analysis of bacterial communities in bulk and rhizosphere sediments. Composite samples (four subsamples) of bulk or rhizosphere sediments from each sampling site were used for pyrosequencing analysis. Fragments of the bacterial 16S rRNA gene were sequenced for each sample with primers V3 Forward (5′-ACTCCTACGGGAGGCAG-3′) and V4 Reverse (5′-TACNVRRGTHTCTAATYC-3′) (Wang & Qian, 2009), 1× Advantage 2 Polymerase Mix (Clontech), 1× Advantage 2 PCR Buffer, 0.2 μM of each PCR primer, 0.2 mM dNTPs (Bioron), 5% DMSO (Roche Diagnostics) and 2 μL genomic DNA template in a total volume of 25 μL. The PCR conditions were as follows: 4 min denaturation at 94 °C, followed by 25 cycles of 94 °C for 30 s, 44 °C for 45 s and 68 °C for 60 s, and a final extension at 68 °C for 10 min. Negative controls were included for all amplification reactions. Electrophoresis of duplicate PCR products was undertaken on a 1% (w/v) agarose gel and the 470-bp amplified fragments were purified using AMPure XP beads (Agencourt) or, if more than the expected fragment was amplified, gel-purified using High Pure PCR Product Purification Kit (Roche Diagnostics), according to the manufacturer's instructions. The amplicons were quantified by fluorometry with a PicoGreen dsDNA quantitation kit (Invitrogen, Life Technologies), pooled at equimolar concentrations and sequenced in the A direction with GS 454 FLX Titanium chemistry, according to the manufacturer's instructions (Roche, 454 Life Sciences) at Biocant (Cantanhede, Portugal). Sequences generated in this study can be downloaded from the NCBI Short Read Archive (study accession no.: SRP035868). Analysis of the pyrosequencing data was performed using previously described methods (Pires et al., 2012; Cleary et al., 2013; Polónia et al., 2014 see supplementary methods for a detailed description).
Data analysis
Two square matrices were imported into r (R Core Team, 2013) using the read.table() function: (1) containing the presence and raw abundance of all operational taxonomic units (OTUs) per sample generated with qiime and (2) containing band ‘abundance’ based on band intensity and position of the DGGE gel. In the OTU abundance matrix, sequences not classified as bacteria or classified as chloroplasts or mitochondria were removed prior to statistical analysis. Both matrices were log10(x+ 1)-transformed and a distance matrix was constructed using the Bray–Curtis index with the vegdist() function, in the vegan package (Oksanen et al., 2011) in r. The Bray–Curtis index is one of the most frequently applied (dis)similarity indices used in ecology (Legendre & Gallagher, 2001; Cleary, 2003; Cleary et al., 2013). Variation in OTU composition among microhabitats was assessed with principal coordinates analysis (PCO) using the cmdscale() function in r with the Bray–Curtis distance matrix as input. We tested for significant variation in composition among microhabitats using the adonis() function in vegan. In the adonis analysis, the Bray–Curtis distance matrix of species composition was the response variable with biotope as independent variable. In DGGE Bray–Curtis distance matrix, each biotope was the response variable with sampling sites as independent variable. The number of permutations was set to 999; all other arguments used the default values set in the function. Weighted averages scores were computed for OTUs on the first two PCO axes using the wascores() function in the vegan package. Significant differences among microhabitats in the relative abundance of the most abundant bacterial phyla, classes and selected orders and the dominant OTU in each microhabitat were tested with an analysis of deviance using the glm() function in r. Because data were proportional, a glm with the family argument set to binomial was first applied. Because the ratio of residual deviance to residual d.f. in the models substantially exceeded 1, family was set to ‘quasibinomial’. In the ‘quasibinomial’ family, the dispersion parameter is not fixed at 1 so that it can model over-dispersion. Using the glm model, we tested for significant variation among microhabitats using the anova() function in r with the F-test, which is more appropriate when dispersion is estimated by moments, as is the case of quasibinomial fits.
Phylogenetic tree
Selected sequences from dominant OTUs (> 150) and their closest relatives retrieved from GenBank (http://www.ncbi.nlm.nih.gov/) were aligned and a bootstrap consensus tree was built with 500 replicates with mega 5 (http://www.megasoftware.net/). The bootstrap value represents the percentage of replicate trees in which the associated taxa clustered together. For tree inference, the nearest-neighbor interchange (NNI) heuristic method and automatic initial tree selection were used. All positions containing gaps and missing data were eliminated.
In silico metagenome analysis
In the present study, picrust (Langille et al., 2013) was used to predict the metagenome of each sample. picrust is a bioinformatics tool that uses marker genes, in this case 16S rRNA, to predict metagenome gene functional content. These predictions are precalculated for genes in databases including KEGG (Kyoto Encyclopedia of Genes and Genomes) and COG (Clusters of Orthologous Groups of proteins). In this study, the kegg database focused on KEGG Orthologous (KOs) in the polyaromatic degradation pathway was used. r was used to generate bargraphs showing the relative abundance of total genes for each sample. Significant differences among biotopes in the relative abundance of total genes were tested with an analysis of deviance using the glm() function in r. The glm model was used to test for significant variation among biotopes using the anova() function in r with the F-test, which is more appropriate when dispersion is estimated by moments, as is the case of quasibinomial fits.
Results and discussion
Sediment properties and hydrocarbon contamination
The sediments of four sites were analysed comparatively based on their physicochemical properties (Table 1) and on the quantification and composition of aliphatic and aromatic hydrocarbons (Table 2). Texture sediment characterization classified all sediments as mud, with the exception of sediment from site B (Table 1). In terms of total hydrocarbons, the concentration calculated as the sum of partial concentrations (aliphatic and 16 PAHs) ranged from 5.86 to 21.39 ng g−1 dry weight (dw), with the highest concentrations observed in site C and the lowest in site B. Sites A and D presented similar levels of overall hydrocarbon contamination (11.05 and 11.47 ng g−1 dw, respectively). Aliphatic hydrocarbons consisted mainly of C10–C32 n-alkanes with total concentration ranging from 3.789 to 10.253 ng g−1 dw (Table 2). The total concentration of 16 PAHs in the sediment ranged from 1.285 (A) to 11.134 (C) ng g−1 dw (Table 3).
Sediment properties in four salt marsh sites of the estuarine system Ria de Aveiro (mean ± SD, n = 3)
Sampling site | ||||||||||||||
A | B | C | D | |||||||||||
pH | 7.12 ± 0.10 | 8.34 ± 0.20 | 7.49 ± 0.26 | 7.24 ± 0.24 | ||||||||||
Organic matter (%) | 4.54 ± 1.13 | 2.67 ± 1.15 | 5.81 ± 2.08 | 7.20 ± 2.97 | ||||||||||
Moisture (%) | 22.47 ± 5.03 | 17.74 ± 3.18 | 36.61 ± 8.18 | 40.10 ± 10.33 | ||||||||||
% Fines | 58.06 ± 0.06 | 20.96 ± 0.06 | 81.99 ± 0.13 | 62.67 ± 0.03 | ||||||||||
Sediment texture | Mud | Very fine sand | Mud | Mud |
Sampling site | ||||||||||||||
A | B | C | D | |||||||||||
pH | 7.12 ± 0.10 | 8.34 ± 0.20 | 7.49 ± 0.26 | 7.24 ± 0.24 | ||||||||||
Organic matter (%) | 4.54 ± 1.13 | 2.67 ± 1.15 | 5.81 ± 2.08 | 7.20 ± 2.97 | ||||||||||
Moisture (%) | 22.47 ± 5.03 | 17.74 ± 3.18 | 36.61 ± 8.18 | 40.10 ± 10.33 | ||||||||||
% Fines | 58.06 ± 0.06 | 20.96 ± 0.06 | 81.99 ± 0.13 | 62.67 ± 0.03 | ||||||||||
Sediment texture | Mud | Very fine sand | Mud | Mud |
Sediment properties in four salt marsh sites of the estuarine system Ria de Aveiro (mean ± SD, n = 3)
Sampling site | ||||||||||||||
A | B | C | D | |||||||||||
pH | 7.12 ± 0.10 | 8.34 ± 0.20 | 7.49 ± 0.26 | 7.24 ± 0.24 | ||||||||||
Organic matter (%) | 4.54 ± 1.13 | 2.67 ± 1.15 | 5.81 ± 2.08 | 7.20 ± 2.97 | ||||||||||
Moisture (%) | 22.47 ± 5.03 | 17.74 ± 3.18 | 36.61 ± 8.18 | 40.10 ± 10.33 | ||||||||||
% Fines | 58.06 ± 0.06 | 20.96 ± 0.06 | 81.99 ± 0.13 | 62.67 ± 0.03 | ||||||||||
Sediment texture | Mud | Very fine sand | Mud | Mud |
Sampling site | ||||||||||||||
A | B | C | D | |||||||||||
pH | 7.12 ± 0.10 | 8.34 ± 0.20 | 7.49 ± 0.26 | 7.24 ± 0.24 | ||||||||||
Organic matter (%) | 4.54 ± 1.13 | 2.67 ± 1.15 | 5.81 ± 2.08 | 7.20 ± 2.97 | ||||||||||
Moisture (%) | 22.47 ± 5.03 | 17.74 ± 3.18 | 36.61 ± 8.18 | 40.10 ± 10.33 | ||||||||||
% Fines | 58.06 ± 0.06 | 20.96 ± 0.06 | 81.99 ± 0.13 | 62.67 ± 0.03 | ||||||||||
Sediment texture | Mud | Very fine sand | Mud | Mud |
Concentration of aliphatic hydrocarbons (ng g−1 dw) and values of selected source diagnostic indices in four salt marsh sites of the estuarine system Ria de Aveiro (mean ± STD, n = 3)
Aliphatics (n-C10–C32) | Sampling site | |||||||||||||
A | B | C | D | |||||||||||
nC17 | 0.310 ± 0.130 | 0.160 ± 0.068 | 0.497 ± 0.332 | 0.491 ± 0.299 | ||||||||||
nC18 | 0.528 ± 0.068 | 0.370 ± 0.018 | 1.084 ± 0.188 | 1.170 ± 0.257 | ||||||||||
Pr (nC19) | 0.382 ± 0.103 | 0.326 ± 0.014 | 0.509 ± 0.161 | 0.474 ± 0.268 | ||||||||||
Ph (nC20) | 0.681 ± 0.305 | 0.235 ± 0.230 | 0.947 ± 0.226 | 0.813 ± 0.341 | ||||||||||
ΣAliphatics (n-C10-C32) | 9.764 ± 0.171 | 3.789 ± 0.058 | 10.253 ± 0.140 | 9.527 ± 0.142 | ||||||||||
Pr/Ph | 0.562 | 1.390 | 0.537 | 0.582 | ||||||||||
nC17/Pr | 0.812 | 0.490 | 0.977 | 1.037 | ||||||||||
nC18/Ph | 0.776 | 1.577 | 1.145 | 1.438 | ||||||||||
ΣTotal HC | 11.050 ± 0.111 | 5.859 ± 0.006 | 21.386 ± 0.002 | 11.471 ± 0.068 |
Aliphatics (n-C10–C32) | Sampling site | |||||||||||||
A | B | C | D | |||||||||||
nC17 | 0.310 ± 0.130 | 0.160 ± 0.068 | 0.497 ± 0.332 | 0.491 ± 0.299 | ||||||||||
nC18 | 0.528 ± 0.068 | 0.370 ± 0.018 | 1.084 ± 0.188 | 1.170 ± 0.257 | ||||||||||
Pr (nC19) | 0.382 ± 0.103 | 0.326 ± 0.014 | 0.509 ± 0.161 | 0.474 ± 0.268 | ||||||||||
Ph (nC20) | 0.681 ± 0.305 | 0.235 ± 0.230 | 0.947 ± 0.226 | 0.813 ± 0.341 | ||||||||||
ΣAliphatics (n-C10-C32) | 9.764 ± 0.171 | 3.789 ± 0.058 | 10.253 ± 0.140 | 9.527 ± 0.142 | ||||||||||
Pr/Ph | 0.562 | 1.390 | 0.537 | 0.582 | ||||||||||
nC17/Pr | 0.812 | 0.490 | 0.977 | 1.037 | ||||||||||
nC18/Ph | 0.776 | 1.577 | 1.145 | 1.438 | ||||||||||
ΣTotal HC | 11.050 ± 0.111 | 5.859 ± 0.006 | 21.386 ± 0.002 | 11.471 ± 0.068 |
Pr, pristine; Ph, phytane; Total HC, total hydrocarbons = sum of total aliphatics (n-C10-C32) + Σ16 PAHs.
Concentration of aliphatic hydrocarbons (ng g−1 dw) and values of selected source diagnostic indices in four salt marsh sites of the estuarine system Ria de Aveiro (mean ± STD, n = 3)
Aliphatics (n-C10–C32) | Sampling site | |||||||||||||
A | B | C | D | |||||||||||
nC17 | 0.310 ± 0.130 | 0.160 ± 0.068 | 0.497 ± 0.332 | 0.491 ± 0.299 | ||||||||||
nC18 | 0.528 ± 0.068 | 0.370 ± 0.018 | 1.084 ± 0.188 | 1.170 ± 0.257 | ||||||||||
Pr (nC19) | 0.382 ± 0.103 | 0.326 ± 0.014 | 0.509 ± 0.161 | 0.474 ± 0.268 | ||||||||||
Ph (nC20) | 0.681 ± 0.305 | 0.235 ± 0.230 | 0.947 ± 0.226 | 0.813 ± 0.341 | ||||||||||
ΣAliphatics (n-C10-C32) | 9.764 ± 0.171 | 3.789 ± 0.058 | 10.253 ± 0.140 | 9.527 ± 0.142 | ||||||||||
Pr/Ph | 0.562 | 1.390 | 0.537 | 0.582 | ||||||||||
nC17/Pr | 0.812 | 0.490 | 0.977 | 1.037 | ||||||||||
nC18/Ph | 0.776 | 1.577 | 1.145 | 1.438 | ||||||||||
ΣTotal HC | 11.050 ± 0.111 | 5.859 ± 0.006 | 21.386 ± 0.002 | 11.471 ± 0.068 |
Aliphatics (n-C10–C32) | Sampling site | |||||||||||||
A | B | C | D | |||||||||||
nC17 | 0.310 ± 0.130 | 0.160 ± 0.068 | 0.497 ± 0.332 | 0.491 ± 0.299 | ||||||||||
nC18 | 0.528 ± 0.068 | 0.370 ± 0.018 | 1.084 ± 0.188 | 1.170 ± 0.257 | ||||||||||
Pr (nC19) | 0.382 ± 0.103 | 0.326 ± 0.014 | 0.509 ± 0.161 | 0.474 ± 0.268 | ||||||||||
Ph (nC20) | 0.681 ± 0.305 | 0.235 ± 0.230 | 0.947 ± 0.226 | 0.813 ± 0.341 | ||||||||||
ΣAliphatics (n-C10-C32) | 9.764 ± 0.171 | 3.789 ± 0.058 | 10.253 ± 0.140 | 9.527 ± 0.142 | ||||||||||
Pr/Ph | 0.562 | 1.390 | 0.537 | 0.582 | ||||||||||
nC17/Pr | 0.812 | 0.490 | 0.977 | 1.037 | ||||||||||
nC18/Ph | 0.776 | 1.577 | 1.145 | 1.438 | ||||||||||
ΣTotal HC | 11.050 ± 0.111 | 5.859 ± 0.006 | 21.386 ± 0.002 | 11.471 ± 0.068 |
Pr, pristine; Ph, phytane; Total HC, total hydrocarbons = sum of total aliphatics (n-C10-C32) + Σ16 PAHs.
Concentration of PAHs (ng g−1 dw) in four salt marsh sites of the estuarine system Ria de Aveiro (mean ± STD, n = 3)
PAH | Sampling site | |||||||||||||
A | B | C | D | |||||||||||
2- to 3-ring | ||||||||||||||
Naph | 0.207 ± 0.049 | 0.204 ± 0.091 | 0.279 ± 0.092 | 0.205 ± 0.059 | ||||||||||
Aceph | 0.049 ± 0.002 | 0.053 ± 0.004 | 0.121 ± 0.021 | 0.054 ± 0.004 | ||||||||||
Ace | 0.048 ± 0.004 | 0.046 ± 0.001 | 0.051 ± 0.002 | 0.045 ± 0.001 | ||||||||||
Flu | 0.061 ± 0.004 | 0.052 ± 0.003 | 0.111 ± 0.008 | 0.051 ± 0.004 | ||||||||||
Phe | 0.090 ± 0.012 | 0.120 ± 0.031 | 0.710 ± 0.193 | 0.091 ± 0.013 | ||||||||||
Ant | 0.061 ± 0.001 | 0.070 ± 0.011 | 0.370 ± 0.243 | 0.059 ± 0.002 | ||||||||||
4-ring | ||||||||||||||
Fluor | 0.081 ± 0.021 | 0.186 ± 0.128 | 1.502 ± 0.185 | 0.116 ± 0.039 | ||||||||||
Pyr | 0.071 ± 0.019 | 0.163 ± 0.105 | 1.155 ± 0.120 | 0.102 ± 0.031 | ||||||||||
BaA | 0.069 ± 0.015 | 0.128 ± 0.063 | 0.832 ± 0.217 | 0.118 ± 0.052 | ||||||||||
Chr | 0.056 ± 0.009 | 0.099 ± 0.060 | 0.474 ± 0.088 | 0.089 ± 0.024 | ||||||||||
5- to 6-ring | ||||||||||||||
BbF | 0.096 ± 0.039 | 0.188 ± 0.097 | 1.330 ± 0.257 | 0.228 ± 0.097 | ||||||||||
BkF | 0.066 ± 0.007 | 0.111 ± 0.046 | 0.307 ± 0.050 | 0.089 ± 0.020 | ||||||||||
BaP | 0.098 ± 0.022 | 0.268 ± 0.170 | 2.032 ± 0.551 | 0.288 ± 0.176 | ||||||||||
InP | 0.097 ± 0.029 | 0.167 ± 0.066 | 0.996 ± 0.184 | 0.183 ± 0.073 | ||||||||||
DahA | 0.064 ± 0.005 | 0.077 ± 0.008 | 0.161 ± 0.041 | 0.074 ± 0.009 | ||||||||||
BghiP | 0.073 ± 0.007 | 0.139 ± 0.051 | 0.702 ± 0.114 | 0.150 ± 0.062 | ||||||||||
Σ16 PAHs | 1.285 ± 0.014 | 2.071 ± 0.050 | 11.134 ± 0.136 | 1.944 ± 0.046 |
PAH | Sampling site | |||||||||||||
A | B | C | D | |||||||||||
2- to 3-ring | ||||||||||||||
Naph | 0.207 ± 0.049 | 0.204 ± 0.091 | 0.279 ± 0.092 | 0.205 ± 0.059 | ||||||||||
Aceph | 0.049 ± 0.002 | 0.053 ± 0.004 | 0.121 ± 0.021 | 0.054 ± 0.004 | ||||||||||
Ace | 0.048 ± 0.004 | 0.046 ± 0.001 | 0.051 ± 0.002 | 0.045 ± 0.001 | ||||||||||
Flu | 0.061 ± 0.004 | 0.052 ± 0.003 | 0.111 ± 0.008 | 0.051 ± 0.004 | ||||||||||
Phe | 0.090 ± 0.012 | 0.120 ± 0.031 | 0.710 ± 0.193 | 0.091 ± 0.013 | ||||||||||
Ant | 0.061 ± 0.001 | 0.070 ± 0.011 | 0.370 ± 0.243 | 0.059 ± 0.002 | ||||||||||
4-ring | ||||||||||||||
Fluor | 0.081 ± 0.021 | 0.186 ± 0.128 | 1.502 ± 0.185 | 0.116 ± 0.039 | ||||||||||
Pyr | 0.071 ± 0.019 | 0.163 ± 0.105 | 1.155 ± 0.120 | 0.102 ± 0.031 | ||||||||||
BaA | 0.069 ± 0.015 | 0.128 ± 0.063 | 0.832 ± 0.217 | 0.118 ± 0.052 | ||||||||||
Chr | 0.056 ± 0.009 | 0.099 ± 0.060 | 0.474 ± 0.088 | 0.089 ± 0.024 | ||||||||||
5- to 6-ring | ||||||||||||||
BbF | 0.096 ± 0.039 | 0.188 ± 0.097 | 1.330 ± 0.257 | 0.228 ± 0.097 | ||||||||||
BkF | 0.066 ± 0.007 | 0.111 ± 0.046 | 0.307 ± 0.050 | 0.089 ± 0.020 | ||||||||||
BaP | 0.098 ± 0.022 | 0.268 ± 0.170 | 2.032 ± 0.551 | 0.288 ± 0.176 | ||||||||||
InP | 0.097 ± 0.029 | 0.167 ± 0.066 | 0.996 ± 0.184 | 0.183 ± 0.073 | ||||||||||
DahA | 0.064 ± 0.005 | 0.077 ± 0.008 | 0.161 ± 0.041 | 0.074 ± 0.009 | ||||||||||
BghiP | 0.073 ± 0.007 | 0.139 ± 0.051 | 0.702 ± 0.114 | 0.150 ± 0.062 | ||||||||||
Σ16 PAHs | 1.285 ± 0.014 | 2.071 ± 0.050 | 11.134 ± 0.136 | 1.944 ± 0.046 |
Concentration of PAHs (ng g−1 dw) in four salt marsh sites of the estuarine system Ria de Aveiro (mean ± STD, n = 3)
PAH | Sampling site | |||||||||||||
A | B | C | D | |||||||||||
2- to 3-ring | ||||||||||||||
Naph | 0.207 ± 0.049 | 0.204 ± 0.091 | 0.279 ± 0.092 | 0.205 ± 0.059 | ||||||||||
Aceph | 0.049 ± 0.002 | 0.053 ± 0.004 | 0.121 ± 0.021 | 0.054 ± 0.004 | ||||||||||
Ace | 0.048 ± 0.004 | 0.046 ± 0.001 | 0.051 ± 0.002 | 0.045 ± 0.001 | ||||||||||
Flu | 0.061 ± 0.004 | 0.052 ± 0.003 | 0.111 ± 0.008 | 0.051 ± 0.004 | ||||||||||
Phe | 0.090 ± 0.012 | 0.120 ± 0.031 | 0.710 ± 0.193 | 0.091 ± 0.013 | ||||||||||
Ant | 0.061 ± 0.001 | 0.070 ± 0.011 | 0.370 ± 0.243 | 0.059 ± 0.002 | ||||||||||
4-ring | ||||||||||||||
Fluor | 0.081 ± 0.021 | 0.186 ± 0.128 | 1.502 ± 0.185 | 0.116 ± 0.039 | ||||||||||
Pyr | 0.071 ± 0.019 | 0.163 ± 0.105 | 1.155 ± 0.120 | 0.102 ± 0.031 | ||||||||||
BaA | 0.069 ± 0.015 | 0.128 ± 0.063 | 0.832 ± 0.217 | 0.118 ± 0.052 | ||||||||||
Chr | 0.056 ± 0.009 | 0.099 ± 0.060 | 0.474 ± 0.088 | 0.089 ± 0.024 | ||||||||||
5- to 6-ring | ||||||||||||||
BbF | 0.096 ± 0.039 | 0.188 ± 0.097 | 1.330 ± 0.257 | 0.228 ± 0.097 | ||||||||||
BkF | 0.066 ± 0.007 | 0.111 ± 0.046 | 0.307 ± 0.050 | 0.089 ± 0.020 | ||||||||||
BaP | 0.098 ± 0.022 | 0.268 ± 0.170 | 2.032 ± 0.551 | 0.288 ± 0.176 | ||||||||||
InP | 0.097 ± 0.029 | 0.167 ± 0.066 | 0.996 ± 0.184 | 0.183 ± 0.073 | ||||||||||
DahA | 0.064 ± 0.005 | 0.077 ± 0.008 | 0.161 ± 0.041 | 0.074 ± 0.009 | ||||||||||
BghiP | 0.073 ± 0.007 | 0.139 ± 0.051 | 0.702 ± 0.114 | 0.150 ± 0.062 | ||||||||||
Σ16 PAHs | 1.285 ± 0.014 | 2.071 ± 0.050 | 11.134 ± 0.136 | 1.944 ± 0.046 |
PAH | Sampling site | |||||||||||||
A | B | C | D | |||||||||||
2- to 3-ring | ||||||||||||||
Naph | 0.207 ± 0.049 | 0.204 ± 0.091 | 0.279 ± 0.092 | 0.205 ± 0.059 | ||||||||||
Aceph | 0.049 ± 0.002 | 0.053 ± 0.004 | 0.121 ± 0.021 | 0.054 ± 0.004 | ||||||||||
Ace | 0.048 ± 0.004 | 0.046 ± 0.001 | 0.051 ± 0.002 | 0.045 ± 0.001 | ||||||||||
Flu | 0.061 ± 0.004 | 0.052 ± 0.003 | 0.111 ± 0.008 | 0.051 ± 0.004 | ||||||||||
Phe | 0.090 ± 0.012 | 0.120 ± 0.031 | 0.710 ± 0.193 | 0.091 ± 0.013 | ||||||||||
Ant | 0.061 ± 0.001 | 0.070 ± 0.011 | 0.370 ± 0.243 | 0.059 ± 0.002 | ||||||||||
4-ring | ||||||||||||||
Fluor | 0.081 ± 0.021 | 0.186 ± 0.128 | 1.502 ± 0.185 | 0.116 ± 0.039 | ||||||||||
Pyr | 0.071 ± 0.019 | 0.163 ± 0.105 | 1.155 ± 0.120 | 0.102 ± 0.031 | ||||||||||
BaA | 0.069 ± 0.015 | 0.128 ± 0.063 | 0.832 ± 0.217 | 0.118 ± 0.052 | ||||||||||
Chr | 0.056 ± 0.009 | 0.099 ± 0.060 | 0.474 ± 0.088 | 0.089 ± 0.024 | ||||||||||
5- to 6-ring | ||||||||||||||
BbF | 0.096 ± 0.039 | 0.188 ± 0.097 | 1.330 ± 0.257 | 0.228 ± 0.097 | ||||||||||
BkF | 0.066 ± 0.007 | 0.111 ± 0.046 | 0.307 ± 0.050 | 0.089 ± 0.020 | ||||||||||
BaP | 0.098 ± 0.022 | 0.268 ± 0.170 | 2.032 ± 0.551 | 0.288 ± 0.176 | ||||||||||
InP | 0.097 ± 0.029 | 0.167 ± 0.066 | 0.996 ± 0.184 | 0.183 ± 0.073 | ||||||||||
DahA | 0.064 ± 0.005 | 0.077 ± 0.008 | 0.161 ± 0.041 | 0.074 ± 0.009 | ||||||||||
BghiP | 0.073 ± 0.007 | 0.139 ± 0.051 | 0.702 ± 0.114 | 0.150 ± 0.062 | ||||||||||
Σ16 PAHs | 1.285 ± 0.014 | 2.071 ± 0.050 | 11.134 ± 0.136 | 1.944 ± 0.046 |
The localization of the sampling points may explain to some extent the differences in concentration of hydrocarbons obtained. Sites A and C are located in the Mira channel, one of the four main channels of the estuary system, whereas sites B and D are located in secondary channels. Another important feature of site C, the most contaminated site, is that it is located close to the Port of Aveiro and exposed to greater anthropogenic activity (recreational, navigation, urban runoff and shipping activity). Because hydrocarbons may have multiple origins, some aliphatic diagnostic indices were used to identify biogenic (terrestrial and marine) and/or anthropogenic sources. Pristane (C19) and phytane (C20) are common isoprenoids in coastal marine sediments and good indicators of petroleum contamination (Readman et al., 2002). The pristane to phytane ratios (Pr/Ph) of ≤ 1 reflect petroleum contamination and higher ratios indicate a biogenic source. In this study, the Pr/Ph ratios (Table 2) indicate that hydrocarbons in sediments are probably associated with petroleum contamination in sites A, C and D and are predominantly of biogenic origin at site B. Other aliphatic indicators, such as n-C17/Pr and n-C18/Ph ratios (Table 2), are useful as indicators of early microbial degradation (Díez et al., 2007). Generally, lower n-C17/Pr ratios (< 1) reflect the relative contribution of allochthonous and autochthonous hydrocarbons to the sediment (Mille et al., 2007). The calculated n-C18/Ph ratios in sediments from sites A and C were low (0.78–1.14), which indicates that microbial biodegradation of n-alkanes is an important process at these sites (Díez et al., 2007). The composition of the sediment PAH pool showed some differences between sites. There was a marked predominance of five- and six-ring PAHs at sites B, C and D but two- and three-ring PAHs were most abundant in sediment from site A (Table 3). This pattern may be related with the prevailing conditions at different estuarine sites, as high-molecular-weight (HMW) PAHs tend to predominate in sediments from marine and river environments (Yan et al., 2009; Guo et al., 2011; Commendatore et al., 2012; Gonul & Kucuksezgin, 2012). PAH molecular indices based on the ratios of selected PAH concentrations may help to infer the pyrogenic (originated from the combustion of fuels) or petrogenic (originated from petroleum) origin of the PAH pool (Budzinski et al., 1997). According to the diagnostic criteria (Table 4), PAHs found in the sediments of Ria de Aveiro are associated with fossil fuel combustion (pyrogenic sources). The ratio of LMW to HMW PAHs ranged from 0.173 to 0.669 (Table 4). Taken together with the Phe/Ant, Fluor/Pyr, Fluor/(Fluor + Pyr) and InP/(InP + BghiP) ratios (Luo et al., 2005), the results confirm that the PAH contamination of salt marsh sediments of Ria de Aveiro is typically related to chronic fossil fuel exposure.
LMW/HMW | Phe/Ant | Fluor/Pyr | Fluor/(Fluor + Pyr) | InP/(InP + BghiP) | ||||||||||||||||
Pyrolytic source | < 1 | < 10 | > 1 | > 0.5 | > 0.5 | |||||||||||||||
Petrogenic source | > 1 | > 15 | < 1 | < 0.5 | < 0.2 | |||||||||||||||
References | Soclo et al. (2000), Tam et al. (2001), Magi et al. (2002) | Budzinski et al. (1997), Baumard et al. (1998a, b) | Budzinski et al. (1997), Baumard et al. (1998a, b) | Gogou et al. (1998) | Yunker et al. (2002) | |||||||||||||||
Sampling site | ||||||||||||||||||||
A | 0.669 | 1.488 | 1.135 | 0.532 | 0.572 | |||||||||||||||
B | 0.358 | 1.716 | 1.140 | 0.533 | 0.546 | |||||||||||||||
C | 0.173 | 1.917 | 1.300 | 0.565 | 0.587 | |||||||||||||||
D | 0.351 | 1.540 | 1.137 | 0.532 | 0.549 |
LMW/HMW | Phe/Ant | Fluor/Pyr | Fluor/(Fluor + Pyr) | InP/(InP + BghiP) | ||||||||||||||||
Pyrolytic source | < 1 | < 10 | > 1 | > 0.5 | > 0.5 | |||||||||||||||
Petrogenic source | > 1 | > 15 | < 1 | < 0.5 | < 0.2 | |||||||||||||||
References | Soclo et al. (2000), Tam et al. (2001), Magi et al. (2002) | Budzinski et al. (1997), Baumard et al. (1998a, b) | Budzinski et al. (1997), Baumard et al. (1998a, b) | Gogou et al. (1998) | Yunker et al. (2002) | |||||||||||||||
Sampling site | ||||||||||||||||||||
A | 0.669 | 1.488 | 1.135 | 0.532 | 0.572 | |||||||||||||||
B | 0.358 | 1.716 | 1.140 | 0.533 | 0.546 | |||||||||||||||
C | 0.173 | 1.917 | 1.300 | 0.565 | 0.587 | |||||||||||||||
D | 0.351 | 1.540 | 1.137 | 0.532 | 0.549 |
LMW, low molecular weight; HMW, high molecular-weight; Phe, phenanthrene; Ant, anthracene; Fluor, fluoranthene; Pyr, pyrene; InP, indeno(1,2,3-cd) pyrene; BghiP, benzo(g,h,i) perylene.
LMW/HMW | Phe/Ant | Fluor/Pyr | Fluor/(Fluor + Pyr) | InP/(InP + BghiP) | ||||||||||||||||
Pyrolytic source | < 1 | < 10 | > 1 | > 0.5 | > 0.5 | |||||||||||||||
Petrogenic source | > 1 | > 15 | < 1 | < 0.5 | < 0.2 | |||||||||||||||
References | Soclo et al. (2000), Tam et al. (2001), Magi et al. (2002) | Budzinski et al. (1997), Baumard et al. (1998a, b) | Budzinski et al. (1997), Baumard et al. (1998a, b) | Gogou et al. (1998) | Yunker et al. (2002) | |||||||||||||||
Sampling site | ||||||||||||||||||||
A | 0.669 | 1.488 | 1.135 | 0.532 | 0.572 | |||||||||||||||
B | 0.358 | 1.716 | 1.140 | 0.533 | 0.546 | |||||||||||||||
C | 0.173 | 1.917 | 1.300 | 0.565 | 0.587 | |||||||||||||||
D | 0.351 | 1.540 | 1.137 | 0.532 | 0.549 |
LMW/HMW | Phe/Ant | Fluor/Pyr | Fluor/(Fluor + Pyr) | InP/(InP + BghiP) | ||||||||||||||||
Pyrolytic source | < 1 | < 10 | > 1 | > 0.5 | > 0.5 | |||||||||||||||
Petrogenic source | > 1 | > 15 | < 1 | < 0.5 | < 0.2 | |||||||||||||||
References | Soclo et al. (2000), Tam et al. (2001), Magi et al. (2002) | Budzinski et al. (1997), Baumard et al. (1998a, b) | Budzinski et al. (1997), Baumard et al. (1998a, b) | Gogou et al. (1998) | Yunker et al. (2002) | |||||||||||||||
Sampling site | ||||||||||||||||||||
A | 0.669 | 1.488 | 1.135 | 0.532 | 0.572 | |||||||||||||||
B | 0.358 | 1.716 | 1.140 | 0.533 | 0.546 | |||||||||||||||
C | 0.173 | 1.917 | 1.300 | 0.565 | 0.587 | |||||||||||||||
D | 0.351 | 1.540 | 1.137 | 0.532 | 0.549 |
LMW, low molecular weight; HMW, high molecular-weight; Phe, phenanthrene; Ant, anthracene; Fluor, fluoranthene; Pyr, pyrene; InP, indeno(1,2,3-cd) pyrene; BghiP, benzo(g,h,i) perylene.
In a recent report, the effect and bioavailability of PAHs in three different marine sediments (muddy, sand and organic) were tested (Lindgren et al., 2014). Their results show that muddy sediment, although containing the highest total PAH concentrations after 60 days, had the lowest bioavailable concentration. On the other hand, sandy sediment contained the lowest total PAH concentrations but the highest bioavailability. In this study, the sandy sediment (site B) has the lowest total hydrocarbon concentration, related to the higher bioavailability characteristic of this type of sediment.
Site-related variation of the structure of microbial communities
DGGE fingerprinting analysis revealed a significant association between composition of bacterial communities and sampling site, in the microhabitats corresponding to the rhizosphere of H. portulacoides (adonisF3,15 = 5.07, P < 0.001, R2 = 0.559), the rhizosphere of S. perennis ssp. perennis (adonisF3,15 = 4.57, P < 0.001, R2 = 0.533) and bulk sediment (adonisF3,15 = 4.18, P < 0.001, R2 = 0.511). These differences can also be observed in the PCO ordinations present in Fig. 2. The first two PCO axes explained c. 50% of the variation, with samples of each sampling site clustering together for each microhabitat. In salt marsh sediments, the composition of the community in terms of the dominant microbial taxa is structured by local factors (Bowen et al., 2009) related to geographical localization, environmental conditions and pollutants (Córdova-Kreylos et al., 2006). Studies conducted in the Ria de Aveiro have suggested that environmental factors structure bacterial communities (Cleary et al., 2012; Oliveira et al., 2012). In this study, localization of the vegetation banks in the complex estuarine system and differential exposure to environmental contamination, together with different physicochemical characteristics and hydrodynamics of each sampling site, might have influenced bacterial composition. The geographical location of site C, close to the port of Aveiro, may explain the higher organic matter content and hydrocarbon concentration in the sediment, as compared with the other sites. Site B is located in a secondary channel, more sheltered from direct sources of contamination or intense anthropogenic activities. In this case, other physicochemical characteristics, and a different sediment texture (Table 1), were probably major determinants of bacterial composition.
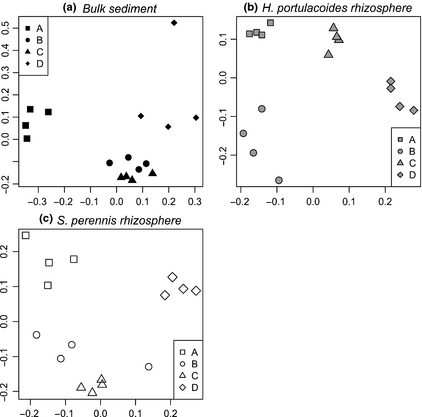
Ordination based on PCO of bacterial community profiles of different microhabitats (a, b, c) in each sampling site (A, B, C and D).
In this study, hydrocarbon concentrations were lower than reported in other salt marshes (Hwang et al., 2006; Watts et al., 2006; Ribeiro et al., 2011). Previous studies have, however, shown that relatively low levels of hydrocarbons in the sediment had an effect on the structural diversity of bacterial communities (Castle et al., 2006; Labbé et al., 2007).
Structure of bacterial communities in salt marsh sediments
The structure of sediment bacterial communities associated with H. portulacoides and S. perennis ssp. perennis roots and bulk salt marsh sediments were determined by 16S rRNA gene amplicons. Proteobacteria represented c. 60% of the total sequences in all samples but Actinobacteria were also well represented in rhizosphere samples (Fig. 3). Bacteroidetes were the third most dominant group, accounting, on average, for 8.3% of the sequences. These three phyla were also the most abundant groups detected in a study conducted in a coastal salt marsh during and after the influx of petroleum hydrocarbons following the Deepwater Horizon oil spill (Beazley et al., 2012). The phylum Proteobacteria is considered a dominant group in estuarine sediment samples (Bowen et al., 2012; Gomes et al., 2013, 2014). Within Proteobacteria, Alphaproteobacteria were the most dominant class in rhizosphere samples (F2,9 = 10.745, P = 0.004) whereas Gammaproteobacteria was the most abundant class in bulk sediment (Fig. 3). The distribution of Alphaproteobacteria in marine and freshwater environments is well documented and hydrocarbonoclastic Alphaproteobacteria have been detected in marine environments (Kim & Kwon, 2010; Newton et al., 2011). On the other hand, Gammaproteobacteria have been reported as abundant in coastal sediment, namely Atlantic port sediments (Gomes et al., 2013), eastern Mediterranean Sea sediments (Polymenakou et al., 2005), north-eastern Pacific sediments (Kouridaki et al., 2010) and South China Sea sediments (Zhu et al., 2013). In bulk sediment, Deltaproteobacteria was the second most dominant class (Fig. 3), immediately following Gammaproteobacteria. The high relative abundance of the order Desulfobacterales in bulk sediment (F2,9 = 4.585, P = 0.042) may be a result of more anaerobic conditions in bulk sediment, which in turn could select for specific guilds such as sulfate-reducing bacteria (SRB). These results are consistent with other studies conducted in the same estuarine system that revealed that the SRB order Desulfobacterales was the most abundant group in unvegetated sediment (Gomes et al., 2010a; Cleary et al., 2012). In these sediments, generally anoxic just below the surface and suboxic to oxic at the surface (Cunha et al., 2005; Santos et al., 2007), anaerobic metabolism such as fermentation and anaerobic respiration may represent the major pathways of organic matter oxidation. The high relative proportion of SRB in salt marsh sediments can be explained by the availability of fermentation-derived substrates directly utilized by SRB (Hines et al., 1999). Moreover, sulfate-reducing strains capable of growing and degrading hydrocarbons have been isolated from hydrocarbon-polluted marine sediment (Cravo-Laureau et al., 2004), and some strains were capable of oxidizing alkenes (Aeckersberg et al., 1991; Cravo-Laureau et al., 2004). SRB play critical roles in a variety of processes in coastal marine sediments such as organic matter turnover, biodegradation of pollutants, sequestration of metals, and sulfur and carbon cycles (Zhang et al., 2008). Also, recent studies have shown that the relative abundance of the order Desulfobacterales increases in marine sediments contaminated with OHs (Suárez-Suárez et al., 2011; Acosta-González et al., 2013). A phylogenetic tree of selected dominant OTUs (> 150 sequence reads) and their closest relatives was constructed (Fig. 4). The phylogenetic analysis revealed bacterial phylotypes closely related to the phyla Proteobacteria, Actinobacteria and Acidobacteria. Only one abundant OTU (18) was associated with bulk sediment. This OTU was classified as belonging to the phylum Acidobacteria and closely related to an uncultured bacterium (AF523900) isolated from forest wetland impacted by coal (Brofft et al., 2002). Most bacterial populations in both rhizospheres showed phylogenetic affiliation with ecotypes from a wide diversity of marine environments. Moreover, the majority of these ecotypes are related to hydrocarbon-impacted sediments (Fig. 4). A study performed after the Prestige oil spill revealed that members of the class Alphaproteobacteria and phylum Actinobacteria were the prevailing groups of bacteria in shoreline environments (Alonso-Gutiérrez et al., 2009). Also, a predominance of Gammaproteobacteria and Deltaproteobacteria in anaerobic bacterial communities of coastal sediments was reported after the Prestige oil spill (Acosta-González et al., 2013). The phylogenetic analysis showed that some OTUs associated with the S. perennis rhizosphere were closely related to gut bacteria (OTU 15) and uncultured Acidobacteria from coastal soils (OTU 45; Fig. 4a).
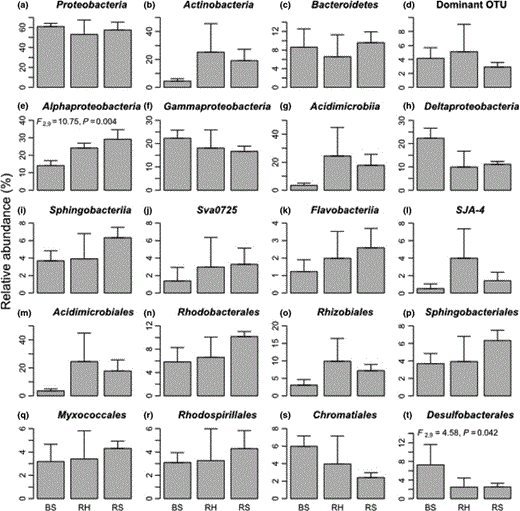
Relative abundance of the most well-represented bacterial taxa: three most abundant phyla (a, b, c), eight most abundant classes (e, f, g, h, i, j, k, l), the eight most abundant orders (m, n, o, p, q, r, s, t) and the most dominant OTU (d) in bulk sediments (BS), Halimione portulacoides rhizosphere (RH) and Sarcocornia perennis ssp. perennis rhizosphere (RS).
![Phylogenetic tree showing the Proteobacteria (a) and Actinobacteria–Acidobacteria–Bacteriodetes (b) clusters from bulk sediment and salt marsh rhizospheres of Halimione portulacoides and Sarcocornia perennis. Close relatives of the selected sequences were obtained by blast searches. H, microhabitat [rhizosphere samples from H. portulacoides (Hal) and S. perennis ssp. perennis (Sar)]; S, sampling site (A, B, C or D); RA, relative abundance of the respective OTU.](https://oup.silverchair-cdn.com/oup/backfile/Content_public/Journal/femsec/90/3/10.1111_1574-6941.12425/1/m_fem12425-fig-0004.gif?Expires=1749842925&Signature=tQbFqDtF~V0qjJE6I9xpFj5VZuFm93i39GYyEohUbuRQZ58LifO9buacHWOEyMOTt4FFzh2jmorSLFBTxrMMw021ouOdbdC~7pLaz1Uzc2uG7cQav3rF~AWn8SmbABSqrs3m7Xtj4S~GtQh0Ahd5WU0Lp~wF7q2evMli9bynnuRq0cN~69hYhQz020Zs0BoS8W1p6HPpm57JKIpMxrnMQzeiijKtb1dZigWMTU~oRLMWOgzJqKs7Rod4pE13DNdI7K7-5ZHTcmwmfwt0ak9--5gCKzVMAdvu1gRHs5xY4KAGzv926k9aiLoU5iNvjiDRL1EdvuOMrfNeetElShu2gQ__&Key-Pair-Id=APKAIE5G5CRDK6RD3PGA)
Phylogenetic tree showing the Proteobacteria (a) and Actinobacteria–Acidobacteria–Bacteriodetes (b) clusters from bulk sediment and salt marsh rhizospheres of Halimione portulacoides and Sarcocornia perennis. Close relatives of the selected sequences were obtained by blast searches. H, microhabitat [rhizosphere samples from H. portulacoides (Hal) and S. perennis ssp. perennis (Sar)]; S, sampling site (A, B, C or D); RA, relative abundance of the respective OTU.
Plant-related variation in the structure of bacterial communities
Overall analysis of the barcoded pyrosequencing data, comparing all rhizosphere samples against bulk sediment samples, showed significant differences between bacterial communities from bulk sediment and rhizospheres (adonisF2,11 = 2.09, P < 0.001, R2 = 0.317). Shannon indices indicate that bacterial communities of the S. perennis ssp. perennis rhizosphere were more diverse than H. portulacoides rhizosphere and bulk sediment (Supporting Information, Table S1). The PCO ordination of bacterial OTUs (Fig. 5) indicates that the two rhizospheres shared the most abundant OTUs (large grey circles; > 150 sequences). The rhizosphere effect can explain the higher number of OTUs in these samples, when compared with bulk sediment, and underlies an apparent convergent adaptation of rhizosphere communities. Plant–bacteria interactions, exerted through the release of exudates that create a unique physicochemical environment surrounding roots, explained the different shifts in bacterial community composition between rhizospheres and bulk sediment. The halophyte exudates provide oxygen and high-quality sources of carbon and energy for bacterial growth (Bagwell et al., 1998). Moreover, roots provide physical support and distinct chemical conditions (Singh et al., 2004; Mucha et al., 2010) and the presence of plants differentially promotes the development of some bacterial groups (Berg & Smalla, 2009; Mucha et al., 2011; Gomes et al., 2014).
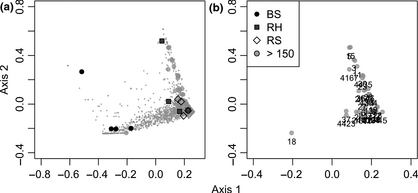
(a) Ordination based on PCO of pyrosequencing data. Samples are represented by symbols (BS, bulk sediment; RH, Halimione portulacoides rhizosphere; RS, Sarcocornia perennis ssp. perennis rhizosphere). OTUs are represented by circles and OTUs with > 150 sequences are represented by large circles. (b) OTU number of dominant 16S rRNA gene sequence reads (> 150 sequences).
As previously mentioned, Actinobacteria and Gammaproteobacteria were more abundant in rhizospheres than bulk sediment and the oxic conditions around the roots may explain a lower relative abundance of Desulfobacterales, and consequently Deltaproteobacteria, in relation to bulk sediment (Fig. 3). The orders Acidimicrobiales, Rhodobacterales, Rhizobiales, Sphingobacteriales and Rhodospirillales were also more abundant in rhizospheres than in bulk sediment (Fig. 3). The rhizosphere of H. portulacoides showed enrichment of Rhizobiales and Acidimicrobiales. Rhizobiales members are involved in atmospheric nitrogen fixation by plants and some members of this group have also been reported as degraders of aromatic hydrocarbon compounds (Baek et al., 2003). Acidimicrobiales members have been considered to be responsive to changes in soil pH (Lauber et al., 2009). Members of the phylum Actinobacteria capable of degrading hydrocarbons were isolated from Artic native plant species (Ferrera-Rodríguez et al., 2013). The rhizosphere of S. perennis ssp. perennis showed enrichment of Rhodobacterales, Sphingobacteriales and Rhodospirillales. Rhodobacterales have been associated with the degradation of aliphatic and LMW aromatic hydrocarbons (Harwati et al., 2007) and the association of Sphingobacteriales and Rhodospirillales members with PAH and petroleum degradation in soil has also been previously reported (Gomes et al., 2010b; Mao et al., 2012).
Phylogenetic analysis of the dominant selected OTUs (> 150 sequence reads) showed that 13 of these OTUs, predominant in rhizosphere samples, are closed related to ecotypes of OH-impacted sediments (Fig. 4). Six OTUs (5, 14, 19, 30, 4167 and 4435) were related to organisms assigned to the order Acidimicrobiales, which were previously reported from oil-polluted subtidal sediments (Acosta-González et al., 2013) as well as from fuel pollution-affected shoreline environments (Alonso-Gutiérrez et al., 2009). OTUs 19 and 14 were enriched in the rhizosphere of S. perennis ssp. perennis in the most contaminated sampling site (C), and OTUs 5, 30, 4167 and 4435 in the rhizosphere of H. portulacoides in sampling site A where the aliphatic hydrocarbon concentration is higher (Fig. 4b; Table 2). The other seven OTUs (7, 10, 20, 21, 24, 36 and 4104) were predominantly enriched in the rhizosphere of S. perennis ssp. perennis in site B, the second most polluted site (Fig. 4a; Table 3). These OTUs are phylogenetically closely related to proteobacterial orders that are known OH degraders. In this study, OTUs 10 and 24 were assigned to Myxococcales and Desulfobacteraceae, respectively. Both of these OTUs are affiliated to sulfate-reducing groups that may play an important role in hydrocarbon degradation in contaminated coastal sediments (Paisse et al., 2008; Acosta-González et al., 2013). Members of Xanthomonadales (OTU 21), Rhodospirillaceae (OTU 7) and Rhizobiales (OTU 20) that were detected in this study were associated with organisms related to either alkane degradation or oil-polluted sites (Viñas et al., 2005; Alonso-Gutiérrez et al., 2009; Patel et al., 2012). According to our phylogenetic analysis, OTU 2094 clustered in the family Rhodobacteraceae. Members of this family have a highly diverse metabolism and include hydrocarbonoclastic species from freshwater and marine environments (Chang et al., 2000; Brakstad & Lødeng, 2005). Two dominant OTUs (38 and 4104) showed close phylogenetic relationship to the genus Erythrobacter. Several marine OH-degrading stains related to Erythrobacter sp. were found to contain cytochrome P450 CYP153 and alkB genes (Wang & Qian, 2009). The alk genes code for an important enzyme involved in n-alkane environmental degradation, and CYP153A genes were detected in petroleum-contaminated soil, groundwater and coastal seawater (Kubota et al., 2005). Our phylogenetic analysis also showed a dominance of OTUs closely related to Actinobacteria in the H. portulacoides rhizosphere, members of which have a high DNA G + C content, are gram-positive and are physiologically diverse. In this study, the OTU sequences for the order Acidimicrobiales were mostly phylogenetically associated with uncultured Actinobacteria members. These observations mirrored results from uncultured (Militon et al., 2010) and culture-based (Pucci et al., 2000) approaches suggesting that Actinobacteria (Acidimicrobiales and Actinomycetales) might play a role in the bioremediation of alkane-contaminated sediments. Autochthonous strains with the capacity to degrade alkanes were isolated from crude-oil-polluted sites (Pucci et al., 2000). Moreover, it was observed that during bioremediation processes the active actinobacterial phylotypes increase (Militon et al., 2010). The abundance of sequences phylogenetically related to hydrocarbon-degrading organisms in the rhizosphere samples analysed in this study provides evidence that halophyte plant colonization is an important driver of the structure of hydrocarbonoclastic bacterial communities in salt marshes and demonstrates the potential of plant–bacteria interactions in processes of microbe-assisted phytoremediation of hydrocarbons in coastal ecosystems.
In silico metagenome analysis
The picrust algorithm was used to predict metagenome functional content based on the kegg classification to identify potential bacterial traits in different microhabitats. Figure 6 shows the relative gene count of selected KOs that might be involved in PAH degradation pathways (www.genome.jp/kegg-bin/show_pathway?ko00624). Significant differences from three selected KOs were observed. KOs K00449, K00517 and K00599 have a higher relative gene frequency in rhizosphere samples, but not K00492, a methyltransfarese involved in the final pathway of phenanthrene and pyrene degradation. K00492, oxidoreductase, is important in the initial steps of the phenanthrene degradation pathway. The protocatechuate 3,4-dioxygenase (K00449) produced by pcaH is important in the final steps of the metabolism of several PAHs (fluorene, anthracene and phenanthrene). K00517 is an oxidoreductase that acts in the initial step of anthracene degradation to 9,10-anthraquinone. These selected KOs are not exclusive of PAH degradation pathways but are involved in other metabolic pathways, in particular those related to the degradation of aromatic compounds (www.genome.jp/kegg/).
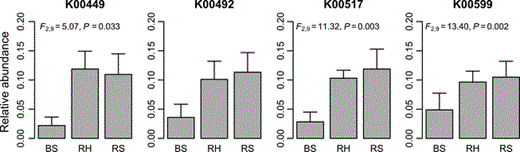
Relative gene count of selected KOs possibly involved in PAH degradation. Samples are represented by symbols (BS, bulk sediment; RH, Halimione portulacoides rhizosphere; RS, Sarcocornia perennis ssp. perennis rhizosphere). KOs enzyme terminology: K00449 - pcaH; K0042 - 1.14.13 (Oxidoreductases); K00517 - E1.14 (Oxidoreductases); K00599 - E2.1.1 (Transferases).
Despite the fact that the data obtained here were derived from an in silico metagenome analysis, the functional genetic information obtained still represents an important resource for the initial evaluation of the distribution of potential bacterial traits in different microhabitats.
Conclusions
Microbial communities in salt marsh sediments appear to respond to interplaying factors related to the physicochemical characteristics of the environment, sediment properties, plant colonization and pollution. The results of this study demonstrate that the structure of bacterial communities is probably affected by site-related factors, such as sediment properties and hydrocarbon contamination, and by plant-related factors.
The barcoded pyrosequencing approach used in this study provided a comprehensive overview of the rhizosphere bacterial communities associated with two important halophyte plants from temperate estuaries. The results indicate that root systems of H. portulacoides and S. perennis ssp. perennis appear to be able to exert a strong influence on bacterial composition. The orders Acidimicrobiales and Rhizobiales were both associated with the rhizospheres of the two halophytes whereas the order Desulfobacterales was associated with bulk sediment. In addition, in silico metagenome analysis revealed a possible higher number of genes involved in the process of PAH degradation in the rhizosphere of halophytic plants. This indicates that halophyte plant colonization could be an important driver of hydrocarbonoclastic bacteria community structure in salt marsh sediments, which can be exploited for in situ phytoremediation of OHs in salt marsh environments.
Acknowledgements
This study received financial support from CESAM research unit (Pest C/MAR/LA0017/2011), QOPNA research unit (PEst-C/QUI/UI0062/2013; FCOMP-01-0124-FEDER-037296), Portuguese Foundation for Science and Technology (FCT) project PTDC/AAC-CLI/107916/2008 (http://alfa.fct.mctes.pt) and COMPETES-(FCOMP-01-0124-FEDER-008657). Financial support to V.O. (SFRH/BD/46977/2008) was provided by FCT in the form of a PhD grant.
References
Author notes
Editor: Tillmann Lueders
CESAM
Pest C/MAR/LA0017/2011
QOPNA
PEst-C/QUI/UI0062/2013
FCOMP-01-0124-FEDER-037296
Portuguese Foundation for Science and Technology (FCT)
PTDC/AAC-CLI/107916/2008
COMPETES
FCOMP-01-0124-FEDER-008657
SFRH/BD/46977/2008