-
PDF
- Split View
-
Views
-
Cite
Cite
Riitta M. Nissinen, Minna K. Männistö, Jan Dirk van Elsas, Endophytic bacterial communities in three arctic plants from low arctic fell tundra are cold-adapted and host-plant specific, FEMS Microbiology Ecology, Volume 82, Issue 2, November 2012, Pages 510–522, https://doi.org/10.1111/j.1574-6941.2012.01464.x
- Share Icon Share
Abstract
Endophytic bacteria inhabit internal plant tissues, and have been isolated from a large diversity of plants, where they form nonpathogenic relationships with their hosts. This study combines molecular and culture-dependent approaches to characterize endophytic bacterial communities of three arcto-alpine plant species (Oxyria digyna, Diapensia lapponica and Juncus trifidus) sampled in the low Arctic (69°03′N). Analyses of a 325 bacterial endophyte isolates, as well as seven clone libraries, revealed a high diversity. In particular, members of the Actinobacteria, Bacteroidetes, Firmicutes, Acidobacteria, and Proteobacteria were found. The compositions of the endophytic bacterial communities were dependent on host-plant species as well as on snow cover at sampling sites. Several bacterial genera were found to be associated tightly with specific host-plant species. In particular, Sphingomonas spp. were characteristic for D. lapponica and O. digyna, and their phylogenetic grouping corresponded to the host plant. Most of the endophyte isolates grew well and retained activity at +4 °C, and isolate as well as clone library sequences were often highly similar to sequences from bacteria from cold environments. Taken together, this study shows that arctic plants harbour a diverse community of bacterial endophytes, a portion of which seems to be tightly associated with specific plant species.
Introduction
In all climates and ecosystems of the earth, plants are associated with diverse arrays of microbes in their phyllo-, rhizo- and endospheres. The relationship between plants and microbes vary from pathogenic to neutral, mutualistic and symbiotic. Endophytic bacteria inhabit the internal plant tissues where they form nonpathogenic, often mutualistic, relationships with their hosts (reviewed in Hardoim et al., 2008; Compant et al., 2010). Several studies on, primarily, agricultural plants suggest that endophytic communities may contribute to plant fitness and positively affect plant nutrient status, the ability to tolerate abiotic and biotic stressors and plant growth (Hardoim et al., 2008; Compant et al., 2010; Reinhold-Hurek & Hurek, 2011). However, as endophytic bacteria have been intensively studied only during the last decades, their overall diversities and interactions with their host plants are still poorly understood. Most published studies describe agricultural plants. The available studies on woody plants focus on species important for forestry or for phytoremediation, mainly Populus spp. (Van der Lelie et al., 2009; Gottel et al., 2011). To the best of our knowledge, there are currently no data on the bacterial endophyte diversity in the Arctic.
Arctic plants are challenged by several abiotic stressors in their environment, many of them being directly and/or indirectly linked to the prevailing low temperatures. The arctic growth season is very short, i.e. 1–4 months and solar radiation is low, with high annual fluctuation. Water stress, either in the form of drought or flooding, is common. However, arctic plants are highly adapted to their environment. In particular, they exhibit low optimal temperatures for photosynthesis and overproduction of cold-sensitive enzymes, allowing for growth rates to be similar to those in temperate regions (Chapin, 1983; Smallwood & Bowles, 2002; Margesin et al., 2007). As recycling of nutrients, especially nitrogen and phosphorus, in cold climates is very slow, the soil is often very poor in plant-accessible nutrients. Therefore, many arctic plant communities are nutrient-limited (Chapin, 1983).
Even though arctic region soils are under a severe weather regime, their microbial diversities can be high (Männistö et al., 2007), as also reported for the Canadian arctic tundra (Chu et al., 2010). Thus, Finnish arctic soils are dominated by members of Acidobacteria, Alpha-, Beta- and Gammaproteobacteria as well as Bacteroidetes and Actinobacteria (Männistö et al., 2007). However, we ignore to what extent plants in these regions contain associated bacteria and what determines the assembly rules that govern such communities. Hence, this study addressed the microbiota in three arctic plant species typical of the Kilpisjärvi fell tundra, i.e. Oxyria digyna, Juncus trifidus and Diapensia lapponica. Oxyria digyna is a nonmycorrhizal arcto-alpine species that is prevalent in snow patch communities, stream banks and rock slides. It is also a typical pioneer species in, for instance, glacier forefronts. Diapensia lapponica is a typical fjell top species. It is chionophobic and evergreen, and, when hardened, extremely cold-tolerant. Juncus trifidus is a monocotyledenous plant (rush) with wide distribution and ability to colonize various habitats. It is a typical high-elevation fell meadow species, often found growing adjacent to D. lapponica. The purpose of the study, incited by the underlying hypothesis, was to assess whether or not host-plant species or sampling site could be shown to be drivers of the endophytic bacterial communities across the three selected arctic plant species.
Materials and methods
Sampling sites, sampling and target plant species
Samples were taken in the Kilpisjärvi fell area, which extends into the Scandinavian mountain range and represents the low arctic–subarctic climate zone. The annual mean temperature is −2.3 °C, and the length of the growing season is about 100 days. The ground is covered with snow typically from mid-October to early-June. Temperature fluctuations are large: from −35 °C in winter to +30 °C in summer, and freeze-thaw cycles are frequent also during the growth season. The vegetation in the valleys consists mostly of boreal mountain birch forest. Above the tree line and on fells, typically an arcto-alpine flora dominates. Sampling was done in early July 2009 in the Kilpisjärvi area (69°′N; 20°50′E) in northern Fennoscandia. The daytime temperatures during the sampling were 5–10 °C. The sampling sites were located in the low- and mid-oroarctic zones above the tree line in fells Saana, Jehkas and Malla (Fig. 1). The sites vary in soil quality (pH, organic matter content), elevation and exposure, as well as prevailing snow coverage (Table 1). From each site, ten individual plants per species were collected. The sites were chosen to include at least two plant species, when possible (Table 1). Healthy-looking plants were collected with sterile spades and gloves. Spades were sterilized between different sampling sites and/or plant species. The material was stored at +2 °C and processed within 48 h after harvesting.
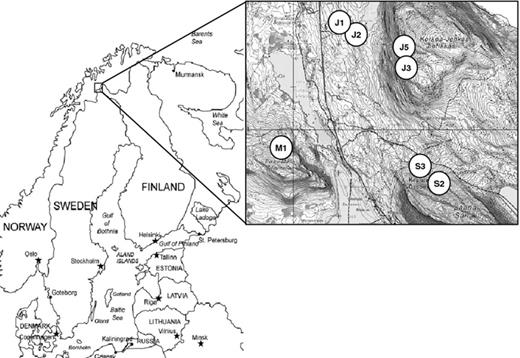
Location and sampling sites. For sampling site properties, refer to Table 1.
Sampling sites
Site | Elevation (m) | pH | Characteristics | Exposure | Sampled plant species |
Fell Saana | |||||
S2 | 754 | 4.9 | Snow patch, rock slide | Snow covered | Oxyria digyna, Diapensia lapponica |
S3 | 632 | 6.5 | Rocky stream bed | Snow covered | Oxyria digyna |
Fell Malla | |||||
M1 | 672 | 5.0 | Fell top | Windswept | Diapensia lapponica, Juncus trifidus |
Fell Jeahkas | |||||
J1 | 559 | 4.8 | Meadow, frost boil, | Snow covered | Diapensia lapponica |
J2 | 589 | 5.3 | Lakeside meadow | Snow covered | Juncus trifidus |
J3 | 898 | 6.1 | Fell top | Windswept | Diapensia lapponica, Juncus trifidus |
J5 | 810 | 6.0 | Rocky stream | Snow covered | Oxyria digyna |
Site | Elevation (m) | pH | Characteristics | Exposure | Sampled plant species |
Fell Saana | |||||
S2 | 754 | 4.9 | Snow patch, rock slide | Snow covered | Oxyria digyna, Diapensia lapponica |
S3 | 632 | 6.5 | Rocky stream bed | Snow covered | Oxyria digyna |
Fell Malla | |||||
M1 | 672 | 5.0 | Fell top | Windswept | Diapensia lapponica, Juncus trifidus |
Fell Jeahkas | |||||
J1 | 559 | 4.8 | Meadow, frost boil, | Snow covered | Diapensia lapponica |
J2 | 589 | 5.3 | Lakeside meadow | Snow covered | Juncus trifidus |
J3 | 898 | 6.1 | Fell top | Windswept | Diapensia lapponica, Juncus trifidus |
J5 | 810 | 6.0 | Rocky stream | Snow covered | Oxyria digyna |
Sampling sites
Site | Elevation (m) | pH | Characteristics | Exposure | Sampled plant species |
Fell Saana | |||||
S2 | 754 | 4.9 | Snow patch, rock slide | Snow covered | Oxyria digyna, Diapensia lapponica |
S3 | 632 | 6.5 | Rocky stream bed | Snow covered | Oxyria digyna |
Fell Malla | |||||
M1 | 672 | 5.0 | Fell top | Windswept | Diapensia lapponica, Juncus trifidus |
Fell Jeahkas | |||||
J1 | 559 | 4.8 | Meadow, frost boil, | Snow covered | Diapensia lapponica |
J2 | 589 | 5.3 | Lakeside meadow | Snow covered | Juncus trifidus |
J3 | 898 | 6.1 | Fell top | Windswept | Diapensia lapponica, Juncus trifidus |
J5 | 810 | 6.0 | Rocky stream | Snow covered | Oxyria digyna |
Site | Elevation (m) | pH | Characteristics | Exposure | Sampled plant species |
Fell Saana | |||||
S2 | 754 | 4.9 | Snow patch, rock slide | Snow covered | Oxyria digyna, Diapensia lapponica |
S3 | 632 | 6.5 | Rocky stream bed | Snow covered | Oxyria digyna |
Fell Malla | |||||
M1 | 672 | 5.0 | Fell top | Windswept | Diapensia lapponica, Juncus trifidus |
Fell Jeahkas | |||||
J1 | 559 | 4.8 | Meadow, frost boil, | Snow covered | Diapensia lapponica |
J2 | 589 | 5.3 | Lakeside meadow | Snow covered | Juncus trifidus |
J3 | 898 | 6.1 | Fell top | Windswept | Diapensia lapponica, Juncus trifidus |
J5 | 810 | 6.0 | Rocky stream | Snow covered | Oxyria digyna |
Endobacterial DNA isolation and bacterial cultures
The plant material was thoroughly washed with tap water, trimmed to remove dead tissue and surface-sterilized by immersion into 2% NaOCl (3 min), 2% sodium thiosulphate (3 min) and sterile distilled water (dH2O; 3 × 3 min). Sterility checks were performed by tissue-blotting surface-sterilized (intact) plant samples on R2A plates, and checking the plates after 2–7 days after plating. Samples with no bacterial growth (all samples but one) were considered successfully sterilized, and only these were included in further study. The samples were divided in two equal portions, one used for molecular analyses, and the other for bacterial isolation. The material for the DNA-based analyses was snap-frozen and stored at −80 °C prior to analysis. For bacterial isolation, the tissues were homogenized by hammering in sterile stomacher bags in sterile potassium phosphate buffer (5 mM, pH 6.5, 5 mL buffer per g plant tissue). The supernatant was used for serial dilution plating on R2A solid medium (Reasoner & Geldreich, 1985; pH adjusted to 6.5; Difco). The plates were incubated either at +23 or +4 °C and screened for colonies at 2, 4, 7, 14 and 21 days after plating, thereafter, all plates were further incubated at +10 °C, and screened once more 6 weeks after plating. In the same way, bacteria were also isolated from pooled samples of surface-sterilized seeds of D. lapponica (22 isolates) and J. trifidus (46), purified and identified as described above.
Culturable bacterial densities were estimated by dilution plating from samples from sites M1, J1, J2, J5, S2 and S3. Individual colonies were picked to produce pure cultures, which were preserved in liquid R2 medium (Reasoner & Geldreich, 1985) with 50% glycerol at −80 °C. Isolates were compared and grouped by BOX-PCR, then presumptively identified by amplification and subsequent sequencing of bacterial 16S rRNA genes with primer 1492R (Lane et al., 1991) and assigned to genera using the closest relative by sequence alignment with the Ribosomal Database Project (RDP) release 9 (http://rdp.cme.msu.edu).
Testing for phenotypes
All bacterial isolates were individually screened for hydrolysis of cellulose and casein, and for solubilization of phosphate by indicator plate assays as described in Nissinen et al. (2001). Cellulase and caseinase assays were performed on M9 minimal salts medium solidified with 1.5% agar and supplemented with either 0.5% carboxymethyl cellulose (Sigma-Aldrich) or with 0.2% skim milk powder, respectively. Ability to solubilize mineral phosphate was tested on National Botanical Research Institute's phosphate growth medium (Nautiyal, 1999). Bacterial isolates were plated on solid media and incubated for 7 days at ambient temperature. The ability of individual isolates to hydrolyze protein or solubilize phosphate was visible as a clear halo around bacterial colonies. The cellulase activity was visible after staining with Congo Red as described in Nissinen et al. (2001). The potential for chitin hydrolysis was screened at ambient temperature (~ 20–23 °C) and on ice (+0 to +2 °C) as described in Howard et al. (2003).
DNA isolation
For isolation of endobacterial community DNA, a method modified from Ikeda et al. (2009) was used to minimize the interference of plant plastid signal as follows: The frozen tissue samples were homogenized with liquid nitrogen, mortar and pestle and transferred to 5 mL potassium phosphate buffer (10 mM, pH 6.5). After spinning down the plant cell material for 5 min at 500 g, the supernatant was collected and pelleted for 30 min at 12 000 g at 4 °C. The supernatant was discarded and the pellet was re-suspended in BCE buffer (50 mM Tris–HCl [pH7.5], 1% Triton X-100, 2 mM mercaptoethanol; Ikeda et al., 2009), incubated for 30 min at room temperature (RT), pelleted for 30 min at 12 000 g at 4 °C, thereafter the supernatant was discarded and the pellet frozen at −20 °C. The bacterial pellet, together with a small portion of sterile 0.1 mm glass beads and 750 μL DNA-extraction buffer (0.2 M Tris-HCL pH 8.5, 0.025 M EDTA pH 8.0, 1% SDS, Sessitsch et al., 2012), was shaken 3 × 40 s using a bead-beater. A 0.1 mg mL−1 proteinase K, and 1 mg mL−1 lysozyme was added, and samples were further incubated for 1 h at 60 °C. An equal volume of Tris- buffered phenol (pH 8.0) was added, mixed manually and centrifuged for 5 min at 100 000 g. The aqueous phase was recovered and the step was repeated with chloroform/isoamylalcohol (24 : 1). The aqueous phase was treated with 0.5 vol 1 M NaCl and 0.6 vol isopropanol, and DNA was precipitated by 15 min incubaction on ice and 15 000 g for 15 min. The DNA pellet was washed with 100% ethanol and the air-dried pellet was rehydrated in 50 μL of TE buffer (0.1 M Tris-HCl, 0.1 M EDTA) and stored at −20 °C. DNA concentration was checked by electrophoresis.
16S rRNA gene amplification, bacterial identification and phylogenetic analyses
The endobacterial 16S rRNA genes were amplified using primer pair 799F–1492R, as primer 799R is reported to exclude plant chloroplasts (Chelius & Triplett, 2001). Amplification from O. digyna extracted DNA was unsuccessful, as it resulted in very weak bacterial signals in comparison to plant organelle amplicons. Thus, the molecular analyses were conducted with D. lapponica and J. trifidus samples only. Clone libraries were constructed from D. lapponica samples M1U, J1U, J3U and S2U and from J. trifidus samples M1Ju, J3Ju and J2Ju (Table 1). The PCR products were resolved on 1% agarose gels, and the endobacterial bands were excised and cloned using the pGEM-T cloning vector kit (Promega). Following transformation and colony growth, 96 clones from each library were sequenced with primer 1492R. After chimeras were removed by Bellerophon (Huber et al., 2004), the sequences were aligned, identified and assigned with closest phylogenetic neighbour using Sequalign and Classify at RDP release 9 (http://rdp.cme.msu.edu/, Cole et al., 2009)) and blast-n at NCBI (http://blast.ncbi.nlm.nih.gov). Aligned sequences were trimmed and analysed with mega 5 (Tamura et al., 2011). The phylogenetic trees were visualized with Interactive Tree of Life -online tool at http://itol.embl.de.
Statistical analysis and sequence submission
Canonical correspondence analysis (CCA) was used to correlate bacterial community composition (expressed as abundance of bacterial genera in each sample) with host-plant species, sampling site and sampling site properties (snow covered/exposed and pH). Significance of these correlations was assessed with the Monte Carlo permutation test (449 permutations). CCA analysis and visualization were performed with the canoco package 4.5.
Sequences have been submitted to European Nucleotide Archive under accession numbers HE814625–HE815460.
Results
Culturable bacterial communities
Endophytic bacterial population densities
The CFU levels ranged between 2.2 × 104 and 4.6 × 104 CFU (g fw)−1 in J. trifidus, between 3.5 × 105 and 1.9 × 106 CFU (g fw)−1 in D. lapponica and 2.2 × 104 and 7.2 × 105 CFU (g fw)−1 in O. digyna. There were no significant differences in the bacterial counts between plates incubated at +20 °C and at +4 °C (data not shown). Thus, most of the isolates can be described as psychrotolerant. Fifty per cent of all isolates were pigmented and yellow and orange pigments were common, whereas purple, pink and red colonies were also detected.
Isolates
In total, 451 isolates representing the endophytic bacterial communities of D. lapponica, J. trifidus and O. digyna from seven sampling sites were purified and grouped by BOX-PCR, and representatives of each group were sequenced with respect to their partial 16S rRNA gene and analysed. Most of the thus analysed isolates originated from O. digyna (158), followed by D. lapponica (110) and J. trifidus (54).
Overall, the isolates represented seven taxonomic divisions: Actinobacteria, Bacteroidetes, Firmicutes, Acidobacteria and α-, β- and γ-Proteobacteria. The most common phyla were Proteobacteria and Actinobacteria (28.6%, 28.6% and 23.3% for α- and β-Proteobacteria and Actinobacteria, respectively) (Fig. 2). In total, 58 bacterial genera were identified across all isolates. Oxyria digyna had the highest diversity, with 41 bacterial genera whereas 19 genera were present each in J. trifidus and in D. lapponica. The most frequently encountered genera were Burkholderia and Sphingomonas (15% and 12% respectively, of the total). Burkholderia spp. dominated the D. lapponica and J. trifidus isolates (28% and 31%, respectively), followed by Sphingomonas and Variovorax spp. in D. lapponica, and by Rhodanobacter and Bradyrhizobium spp. in J. trifidus. In O. digyna, Sphingomonas species represented 13% of the total isolates, followed by Agreia, Mucilaginibacter, Pseudomonas and Rhizobium.
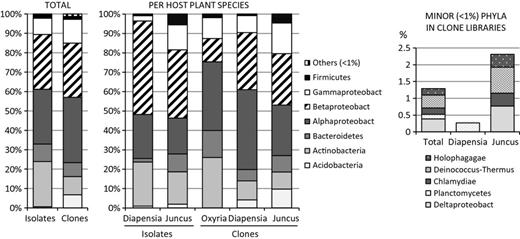
Phylogenetic distribution of endophytic bacteria at phyla/class level expressed as relative abundance in combined isolate library as well as clone library and per host-plant species in isolate and clone libraries. Distribution of minor phyla (< 1% of total) in clone libraries are shown separately. Bacteria were classified based on 16S rRNA gene data with RDP classifier (http://rdp.cme.msu.edu/)
Interestingly, the majority of the isolates were closely related to bacteria that had previously been described either from cold environments or from plants: 40.1% of the closest relatives in public databases were from glaciers, snow, ice, arctic or antarctic or high alpine soils or groundwater. For example, Sphingomonas sp. isolates M1U29 and many others were closely (99%) related to isolate PMX-3 (DQ314734) obtained from endonival plants (Sheng et al., 2011). A 38.6% of closest matches were from plant-associated bacteria, isolated from phyllo-, endo- or rhizospheres. Several of the isolates had less than 97% similarity to any cultured organisms in public databases. These thus may represent new species, including Sphingomonas sp. isolates J1U14, S2H36 and M1Ju36 (Fig. 5) and isolates M1U48 and J1U25, which showed less than 94% identity with type strains of Methylobacterium spp., as well as other Rhizobiales (Supporting Information, Fig. S3).
The endophytic bacterial diversities in the D. lapponica and J. trifidus seeds were clearly lower than in the respective vegetative tissues. The 68 isolates analysed represented ten bacterial genera, which were spread across the Firmicutes, Actinobacteria and Alpha-, Beta- and Gammaproteobacteria. Pseudomonas and Burkholderia were most common genera, with 25 and 19 isolates, respectively.
Clone libraries
To understand the endophytic colonization of Arctic plants at the total community level, seven clone libraries were generated (five sampling sites; four from D. lapponica, samples M1U, J1U, J3U and S2U, and three from J. trifidus, samples M1Ju, J3Ju and J2Ju; Table 1). In total, 620 clones were analysed. In general, these clone libraries were dominated by Alpha- and Betaproteobacteria (34% and 28% of the total clones, respectively), followed by Gammaproteobacteria and Actinobacteria (Fig. 2). The taxon richness in these clone libraries was higher than that in the isolate collections, as sequences from Deltaproteobacteria, Holophagae, Deinococcus-Thermus, Chlamydiae and Planctomycetes were also present.
At the level of genus, 47 bacterial genera, next to 14 unclassified taxa, and 52 genera next to 19 unclassified ones were present in the D. lapponica and J. trifidus libraries, respectively. The most frequent genera in D. lapponica were Sphingomonas, Collimonas and Burkholderia and in J. trifidus Burkholderia, Acidobacterium group 1 and Mucilaginibacter.
The endophytic bacterial community composition is host-plant specific
Several bacterial genera, for example Burkholderia, Sphingomonas, Mucilaginibacter and Micrococcus, were detected in all three plant species studied. However, bacterial genera were not evenly distributed among the sampled plant species. For example, of the 48 Burkholderia isolates, only one was from O. digyna. Likewise, out of a total of 39 Sphingomonas isolates and clone sequences only two were retrieved from J. trifidus (Figs 3 and 5). Moreover, Rhodanobacter spp. were only isolated from J. trifidus.

Distribution of major bacterial genera (a) in isolate libraries of Diapensia lapponica, Juncus trifidus and Oxyria digyna, (b) in clone libraries of D. lapponica and J. trifidus. Numbers in Y-axes indicate numbers of isolates or clones per bacterial genus. Only genera above 3% are shown. Distribution of all bacterial genera in isolate libraries by plant host and site are shown in supplemental Fig. S2.
CCA was then used to analyse the relationship of bacterial community composition with plant species, sampling site and sampling site properties. For both the isolate collections and the clone libraries, the endophytic bacterial community compositions clearly correlated with host-plant species, although sampling site, in particular snow cover, also affected the community compositions (Fig. 4).
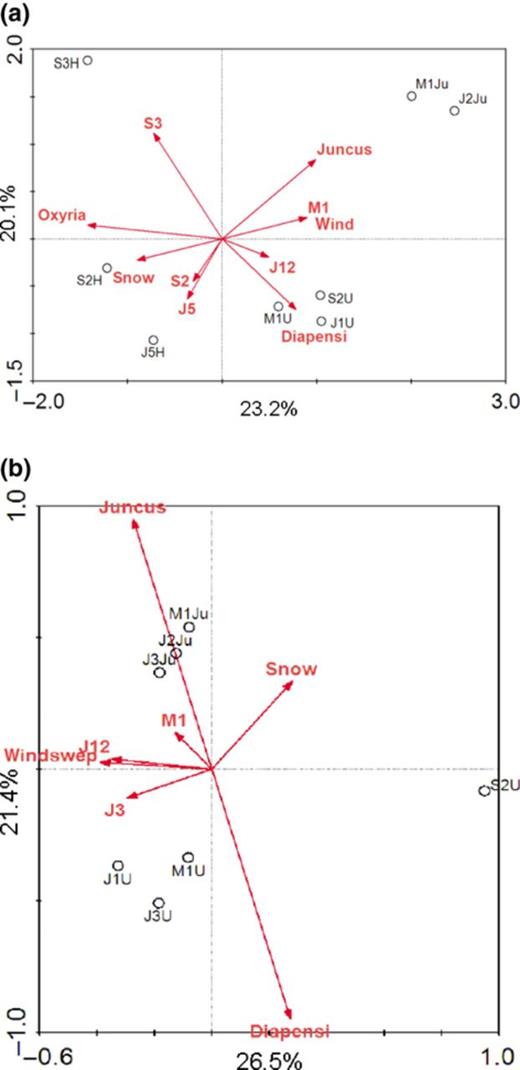
CCA ordination of endophyte community composition in (a) isolate libraries and (b) clone libraries. Samples are labelled with dipartite code indicating sampling site (J12, J3, J5, S2, S3, M1; see Fig. 1 for sampling sites) and sampled plant species (U = Diapensia lapponica, Ju = Juncus trifidus, H = Oxyria digyna). Per cent variation explained is indicated in the axes.
Endophytic Sphingomonas spp. from D. lapponica and O. digyna are host-plant specific
As Sphingomonas and Burkholderia comprised the dominating genera in our isolate collections and clone libraries, we performed phylogenetic analyses of partial 16S rRNA gene sequences of these groups to further investigate their affiliation with their host plants. The alignment of Sphingomonas spp. 16S rRNA gene sequences revealed clustering in two main groups according to host-plant species. The Sphingomonas isolates closely related to Sphingomonas sp. MSCB (EF103200) had all, but one been isolated from O. digyna (Fig. 5). Likewise, the Sphingomonas spp. closely related to Sphingomonas sp. Pmxh (DQ314734) were all, with one exception, provenient from D. lapponica samples. These host-specific Sphingomonas spp. were isolated from their respective hosts from all sampling sites, and, in case of D. lapponica, also from the seeds (Fig. 5). The tight clustering of Sphingomonas spp. was also seen when the clone sequences were included in the alignment: all the Sphingomonas clone library sequences clustered either with D. lapponica-specific Sphingomonas sp. isolate sequences, or formed a separate group (Fig. 5).

Phylogenetic alignment of partial 16S rRNA gene sequences of Sphingomonas spp. from isolate and clone libraries from Diapensia lapponica, Juncus trifidus and Oxyria digyna, and sequences of the most similar sequences from public databases. The evolutionary history was inferred using the Maximum Likelihood method based on the Tamura-Nei model (Tamura & Nei, 1993), with a total of 641 positions in the final dataset sequences. Evolutionary analyses were conducted in mega5 (Tamura et al., 2011), and phylogenetic tree was visualized with Interactive Tree of Life - online tool (http://itol.embl.de). Isolate samples are coded with tripartite code indicating sampling site (M1, J1, J2, J3, J5, S2, S3, see Fig. 1 for sampling site locations), plant species (U = D. lapponica; Ju = J. trifidus; H = O. digyna), followed by isolate number. Clone library sequences kk2, kk3, kk4, kk5 are from D. lapponica sampled in M1, S2, J3 and J1, respectively. No Sphingomonas sp. sequences were detected in J. trifidus clone libraries.
In contrast, the major phylogenetic group of Burkholderia 16S rRNA sequences from isolate collections and clone libraries were distributed evenly in J. trifidus and D. lapponica samples. This major group of Burkholderia spp. was highly similar (98–99%) to Burkholderia sordidicola plant growth promoting isolate BLN20 (GQ181055). A smaller group of Burkholderia spp., clustering with Burkholderia phytofirmans and Burkholderia bryophila, was predominantly present in D. lapponica (Fig. 6).
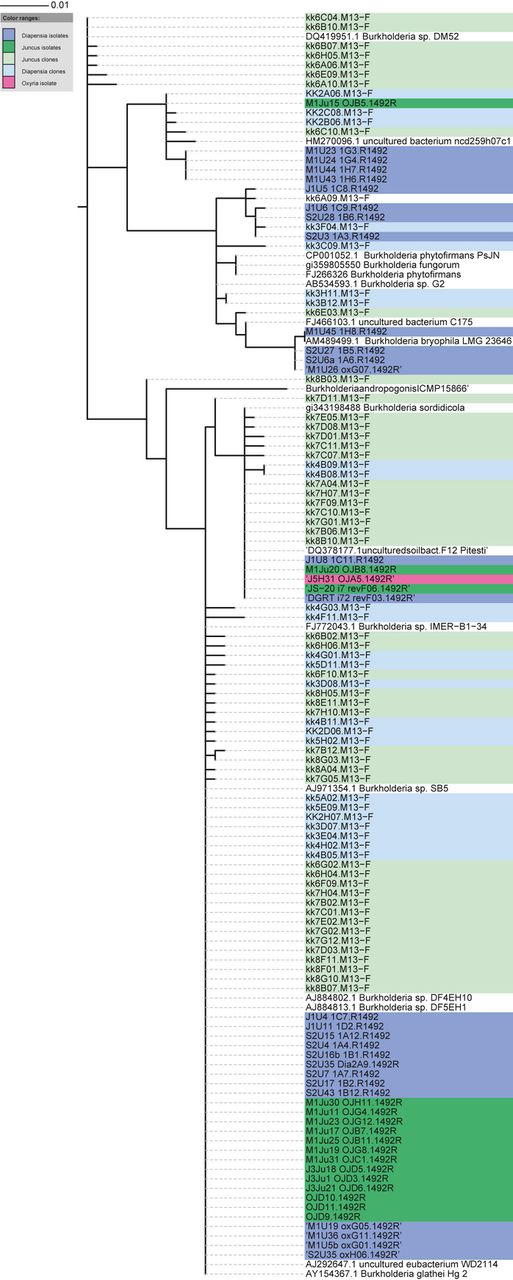
Phylogenetic alignment of partial 16S rRNA gene sequences of Burkholderia spp. from isolate and clone libraries from Diapensia lapponica, Juncus trifidus and Oxyria digyna, and sequences of the closest cultivated relatives from public databases. The evolutionary history was inferred using the Maximum Likelihood method based on the Tamura-Nei model (Tamura & Nei, 1993), with a total of 657 positions in the final dataset sequences. Evolutionary analyses were conducted in mega5 (Tamura et al., 2011), and the phylogenetic tree was visualized with Interactive Tree of Life -online tool (http://itol.embl.de). Isolate samples are noted with tripartite code indicating sampling site (M1, J1, J2, J3, J5, S2, S3, see Fig. 1 and Table 1 for sampling sites), plant species (U = D. lapponica; Ju = J. trifidus; H = O. digyna) and isolate number. Clone library sequences kk2, kk3, kk4, kk5 are from D. lapponica sampled in M1, S2, J3 and J1, respectively and kk6, kk7 and kk8 from J. trifidus sampled in J2, J3 and M1, respectively.
Functional characterization of isolates
All endophyte isolates in the collection were individually tested for their abilities to solubilize mineral phosphate and to degrade chitin, cellulose or protein. Fifty-eight per cent of the isolates, predominantly in the genera Burkholderia, Frondihabitans, Pseudomonas, Sphingomonas and Variovorax were able to solubilize mineral phosphate. Proportionally most phosphate solubilization positive isolates were detected in D. lapponica isolate collection (86% of the isolates), followed by J. trifidus (46%) and O. digyna (35%). The ability to solubilize phosphate was also very common in the isolates from D. lapponica and J. trifidus seeds, 88% of these showed activity.
Furthermore, 29% of the isolates, mainly from the genera Mucilaginibacter, Sphingomonas, Rhizobium and Pedobacter, showed chitinolytic activity. Seventeen per cent and 19% of the isolates showed proteolytic or cellulolytic activity, respectively. The genera Pseudomonas, Micrococcus and Collimonas were common among the protease-positive isolates. Practically all cellulose-positive isolates were from O. digyna, typically representing the bacterial genera Agreia, Sphingomonas and Rhizobium.
The phylogenetic divergence of Spingomonas sp. affiliated with either D. lapponica or O. digyna was also reflected in the phenotypic profiles: isolates from O. digyna were positive for cellulase activity and several of them revealed high chitinase activity. In contrast, D. lapponica-associated Sphingomonas spp. had no cellulolytic activity and weak or no chitinolytic activity.
The isolates positive for chitinase, protease or phosphate solubilization at RT were retested at +0 to 4 °C. Fifty-four per cent (25/46) of chitinolytic strains retained their activity at +0 °C, and 54% (14/26) and 67% (19/28) of isolates showed activity at 4 °C for proteinase and phosphate solubilization, respectively. Bacterial growth and, thus, development of haloes (indicating activity) was clearly slow at 4 °C, and only visible 14 d.p.i, as compared to 7 d.p.i at RT.
Discussion
In this article, we describe the endophytic bacterial communities from three plant species from the European low arctic field tundra. We detected, in total, 58 genera in five phyla in our isolate collection and 73 genera in 12 phyla in the clone libraries. This richness is comparable to that described with similar approaches for temperate and warm climate plants like soybean, rice, potato and poplar (Zinniel et al., 2002; Sessitsch et al., 2004; Ulrich et al., 2008; Ikeda et al., 2009). In addition to the current study, there are two reports of endophytic bacterial communities in cold climate plants. Reiter & Sessitsch (2006) described the endophyte diversity in Crocus albiflora from alpine forest zones in Austria, with 17 genera in three phyla and 38 OTUs in six divisions in their isolate and clone collections, respectively. Sheng et al. (2011) isolated endophytic bacteria from 11 plant species of high altitude alpine tundra, and discovered 12 bacterial genera representing three bacterial phyla. Taken together, the data from this study and these articles indicate that endophytic bacterial communities are no less diverse in Arctic ecosystems than in temperate region plants, corresponding to studies of arctic soil bacterial communities (Neufeld & Mohn, 2005; Chu et al., 2010).
The endophyte populations in the Kilpisjärvi fell plants were dominated by Alpha- and Betaproteobacteria and Actinobacteria, with the bacterial genera Burkholderia and Sphingomonas being most abundant in both the isolate collections and clone libraries. These resemble endophyte communities described from temperate climate, e.g. sugarcane (Mendes et al., 2007), soybean (Kuklinsky-Sobral et al., 2004; Ikeda et al., 2009) and potato (Garbeva et al., 2001; Sessitsch et al., 2004). In contrast, the endophytic bacterial communities in two climate studies were dominated by Actinobacteria (genera Clavibacter, Agreia and Rhodococcus sp.), followed by Gamma- and Alphaproteobacteria (Pseudomonas and Sphingomonas) (Sheng et al., 2011), or by Firmicutes and Gammaproteobacteria (Reiter & Sessitsch, 2006). Thus, this limited data set does not support an idea of any phylogenetic trend in endophytic bacterial communities in cold climates.
Interestingly, 40% of the sequences in public databases that were most similar to our endophyte isolate sequences were from glaciers, snow, ice, Arctic, Antarctic or high-alpine soils or groundwater. For example, the major group of Sphingomonas sp. from D. lapponica (isolate M1U29 and others) were 99% similar to isolate PMX-3 obtained from endonival plants (Sheng et al., 2011; DQ314734). Also, Sheng et al. (2011) reported that 46% of their isolates from subnival plants were highly similar to bacteria from other cold climates, and also the endophyte community of Crocus albiflorus from an alpine forest zone contained several endobacteria closely related to Pseudomonas and Sphingomonas sp. from cold biomes (Reiter & Sessitsch, 2006). Taken together, these data suggest the presence of sets of specific cold-adapted plant-associated bacteria across different habitats.
Many of the isolates in this study were also closely related to soil bacteria that had been isolated previously from the same area. For example, Mucilaginibacter sp. isolate M1Ju16 from J. trifidus was closely related to M. frigoritolerans FT22 that was isolated from Kilpisjärvi soil after experimental freeze-thaw treatment (Männistö et al., 2010). Mucilaginibacter and the closely related Pedobacter spp. have also been isolated from soil and lichen samples from Northern Finland, and were characterized by their abilities to grow at temperatures down to −5 °C (Männistö & Haggblom, 2006). The abundance of these organisms in the tundra ecosystem is likely linked to their cold tolerance. Likewise, Acidobacterium isolate J1U23 isolated in this study was related to the genus Terriglobus. This taxon is also an abundant inhabitant of tundra soil and has been isolated from windswept tundra fell soil (Männistö et al., 2011).
The ‘cold trend’ in phylogenetic affiliation of our isolate and clone sequences was in accordance with the phenotypes of our isolates: all isolates were psychrotolerant and over half of the tested isolates retained enzymatic activities at +0 to 4 °C. Moreover, 50% of the isolates were pigmented. Similar features were also detected in endophytic bacteria isolated from endonival plants by Sheng et al. (2011), who reported over 67% and 70% of isolates being psychrotolerant and pigmented, respectively. Pigmented strains were also common among isolates from the Kilpisjärvi tundra soil (Männistö & Haggblom, 2006). Besides providing protection against ultraviolet radiation, pigments are presumed to play a role in cold adaptation, e.g. by modulating membrane fluidity (Strand et al., 1997; Jagannadham et al., 2000).
The diversity in our isolate collection was relatively high, including isolates of Acidobacteria, Rhodanobacterium and Bradyrhizobium and representatives of unclassified Rhizobiales, none of which, to our knowledge, has previously been described in endophyte communities with culture-dependent approaches. This high isolate diversity was achieved using a combination of relatively low nutrient agar (R2A at pH 6.5), long incubation times and low temperatures (up to 8 weeks, of which 6 weeks at +10 °C).
As expected, our clone libraries contained higher proportions of Acidobacteria, and other rarely cultured bacterial genera, than the isolate collection. However, some clone libraries (e.g. S2U clone library) also contained higher proportions of Collimonas spp. and other supposedly fast-growing organisms than our isolate collection from the same samples. Thus, differences between our isolate and clone libraries could be explained, in addition to culturability, by surface sterilization by sodium hypochlorite treatment, a method that is currently used in most endophyte work (Hallman et al., 2006). Although chlorite ions are very efficient in killing bacteria, (as indicated by the lack of bacterial growth in our negative control plates) this treatment might not be sufficient to eliminate all surface-bound DNA from the rhizoplane. Thus, clone libraries might contain signals from remnant rhizoplane (and phylloplane) populations.
Diapensia lapponica is a mycorrhizal plant species, and has a tight relationship with ericoid mycorrhizal fungi (Read, 2001). Even though we removed as much fungal hyphae as possible, our D. lapponica root samples most likely contain, in addition to plant tissues, mycorrhizal tissue and thus, endofungal or fungal-associated bacteria. This applies to all endomycorrhizal plant species, and should always be considered when studying (endo)mycorrhizal plant species and analysing bacteria from plant endoroots. For example, Burkholderia spp., Collimonas spp. and Variovorax spp. were commonly detected in D. lapponica samples. All these genera contain species known to have a tight association with fungi (Boersma et al., 2010; Leveu et al., 2010).
Host-plant specificity
In our study, the endophyte community composition was divergent between different plant species (Fig. 4), and several major endobacterial genera were distributed in a host-plant species specific manner (Fig. 3). Plant-associated bacterial communities have been shown to depend on the host-plant species and cultivar in vascular plants (Hartmann et al., 2009; Andreote et al., 2010; Hardoim et al., 2011), as well as in bryophytes (Opelt & Berg, 2004; Bragina et al., 2012). As endophytic bacterial populations have been largely considered to be an endocompetent subset of rhizospheric populations (Hardoim et al., 2008; Compant et al., 2010), and as plant species differ in their requirements for optimal growth and thus colonize different sites, differences in the community composition of endophytes between different plant species could be explained by differences in the soil microbiota at these sites.
In our study, differences in the endobacterial communities between O. digyna and the other two plant species could be partially explained by the different habitats favoured by the plants, mainly differences in snow cover (Table 1), and pH, resulting in different soil communities.
However, we found no indication that the endophyte communities would closely follow any variation in the soil bacterial communities, as similar taxa were detected in each plant species growing in soil with wide pH differences and snow cover (Fig. 3, Table 1), and as the endophyte community composition was more dependent on host-plant species than on sampling site (Fig. 4). Further, J. trifidus and D. lapponica harboured clearly different endophyte communities, even though the plants were sampled from the same sites (J12, M1), and sometimes adjacently growing plants were sampled. Taken together, the different endophyte population structures in these three plant species could not be explained by differences in soil bacterial composition on their growth sites. The dependence on host-plant species was visible also at species level, as exemplified by the tight association of specific Sphingomonas spp. either with O. digyna or D. lapponica (Fig. 5). The functional characterization performed by us also suggests functional divergence between the two Sphingomonas groups in this study, at least in their abilities to degrade chitin and cellulose. This would suggest different roles of these bacteria in O. digyna and D. lapponica. Further studies incorporating bacterium-plant interactions, metabolic profiling and gene expression studies are needed to address this issue.
Acknowledgements
This study was funded by an EU 7th framework Marie Curie actions IEF fellowship for R. Nissinen. The field work was funded by the Finnish Cultural Foundation. The authors thank Rauni Partanen and the other staff of Kilpisjärvi Biological Center of University of Helsinki for valuable assistance with the sampling, Pablo Hardoim for reading the manuscript, and Elise Biersma and Patricia Zeinstra from University of Groningen for their excellent help with the seed isolates.
References
Supporting Information
Additional Supporting Information may be found in the online version of the article:
Author notes
Editor: Nina Gunde-Cimerman