-
PDF
- Split View
-
Views
-
Cite
Cite
Maider Iglesias-Carrasco, Beatriz Taboada, Miguel Lozano, Pau Carazo, Roberto Garcia-Roa, Eduardo Rodriguez-Exposito, Francisco Garcia-Gonzalez, Sexual selection buffers the negative consequences of population fragmentation on adaptive plastic responses to increasing temperatures, Evolution, Volume 78, Issue 1, January 2024, Pages 86–97, https://doi.org/10.1093/evolut/qpad193
- Share Icon Share
Abstract
Whether sexual selection facilitates or hampers the ability to plastically respond to novel environments might depend on population structure, via its effects on sexual interactions and associated fitness payoffs. Using experimentally evolved lines of the seed beetle Callosobruchus maculatus, we tested whether individuals evolving under different sexual selection (monogamy vs. polygamy) and population spatial structure (metapopulation vs. undivided populations) treatments differed in their response across developmental thermal conditions (control, hot, or stressful) in a range of fitness and fitness-associated traits. We found that individuals from subdivided populations had lower lifetime reproductive success at hot temperatures, but only in lines evolving under relaxed sexual selection, revealing a complex interaction between sexual selection, population structure, and thermal environmental stress on fitness. We also found an effect of population structure on several traits, including fertility and adult emergence success, under exposure to high thermal conditions. Finally, we found a strong negative effect of hot and stressful temperatures on fitness and associated traits. Our results show that population structure can exacerbate the impact of a warming climate, potentially leading to declines in population viability, but that sexual selection can buffer the negative influence of population subdivision on adaptation to warm temperatures.
Introduction
Determining the factors facilitating species and population persistence in the face of rapid environmental change is a central question in evolutionary ecology and conservation biology. Sexual selection has been proposed as an important moderator of the ability of individuals to cope with a changing environment. For instance, theoretical and empirical studies suggest that sexual selection can accelerate local adaptation and improve population fitness (Agrawal, 2001; Cally et al., 2019; Dugand et al., 2019; Lumley et al., 2015; Parrett et al., 2019; Rowe & Houle, 1996; Siller, 2001; Tomkins et al., 2004; Whitlock, 2000). Since the expression of sexually selected traits is typically condition-dependent, sexual selection is expected to favor the rapid spread of beneficial alleles (Baur & Berger, 2020; Cotton et al., 2006; Parrett et al., 2022), thereby promoting adaptation in the face of environmental change (Lorch et al., 2003; Plesnar-Bielak et al., 2012). In addition, sexual selection can buffer the direct impact of environmental change by promoting plastic responses to maintain/increase the reproductive output of populations under novel conditions (reviewed by Fox et al., 2019).
However, sexual selection can also hamper adaptive plastic responses in novel conditions, increasing the probability of extinction due to the costs of sexual conflict, or due to the interaction between sexual selection and other ecological and demographic factors. On the one hand, sexual conflict can lead to depressed population viability in novel environmental conditions via two mechanisms. First, genetic conflict arising from suboptimal trait expression in one sex due to opposing selection on the same trait in the other sex (Chippindale et al., 2001; Kokko & Brooks, 2003; Long et al., 2012; Rankin & Kokko, 2006). Second, due to conflict over traits that are beneficial for one sex but not for the other, such as male harm to females (Flintham & Savolainen, 2023; Gomez-Llano et al., 2023). The two types of costs are likely to interact and to be impacted by environmental conditions. On the other hand, whether sexual selection hinders adaptation to novel environments might depend on ecological factors, including population spatial structure (Eldakar et al., 2009; Evans & Garcia-Gonzalez, 2016; Perry & Rowe, 2018; Rodriguez-Exposito & Garcia-Gonzalez, 2021). Theoretical and empirical studies exploring the potential interaction between sexual selection and population subdivision in determining adaptation are scant, yet recent studies are highlighting the possibility that spatial structure modulates sexual selection and sexual conflict (McDonald, 2023). For example, metapopulations, where subpopulations of different sizes are connected with each other via the migration of some individuals, have been suggested to be critical in shaping the evolution of mating systems by softening selection (Li Richter & Hollis, 2021; Rodriguez-Exposito & Garcia-Gonzalez, 2021; Wallace, 1975; Yasui & Garcia-Gonzalez, 2016), including reducing the number and the chances of encountering high-quality mates that convey direct or genetic benefits. In addition, fragmented unconnected populations are more likely to become extinct than large or highly connected populations due to isolation, reduced population size, and the increased risk of stochastic genetic and demographic events (Cushman, 2006; Johansson et al., 2007; Zayed et al., 2005). Sexual selection could increase such negative effects due to the stronger reduction in the effective population size associated with paternity biases toward the most attractive/competitive males in sexually reproducing populations/species, which can lead to inbreeding, drift, the fixation of maladaptive alleles, and ultimately extinction in small populations (Keller & Waller, 2002; Saccheri et al., 1998). In this regard, theoretical models have shown that, while sexual selection can result in faster adaptation rates in large populations, it can lead to population extinction due to increased population stochasticity in small populations (Martínez-Ruiz & Knell, 2017). In contrast, a high number of interacting individuals in large populations is predicted to increase sexual conflict and its negative effects on female fitness (Gay et al., 2009, 2010), which could hamper the adaptation ability of such populations to novel conditions. For instance, male harm can decrease population growth and even lead populations to extinction (Flintham & Savolainen, 2023; Gomez-Llano et al., 2023; Le Galliard et al., 2005), and such conflict can be expected to be higher in populations with large numbers of individuals interacting. Interestingly, however, under maladaptation or harsh environmental conditions male and female interests should converge along the axis of viability selection, with genetic conflict being expected to be reduced in populations facing abrupt environmental changes (Connallon & Hall, 2016, 2018; Long et al., 2012). This could, to some extent, reduce the negative effects of conflict over mating. Such complexity and contrasting predictions illustrate the need to empirically test how complex population structure, similar to that observed in the wild, moderates the effect of sexual selection in facilitating or hampering population responses to environmental change.
Temperature is a key environmental factor influencing the biology of organisms (Abram et al., 2017; García-Roa et al., 2020; Noble et al., 2018; Nowakowski et al., 2018; Olsson et al., 2011) and is predicted to impact species viability due to climate change. Temperature changes due to anthropogenic climate change are expected to range from slow and gradual increases across several generations to more abrupt and extreme heat events affecting a specific moment of the life cycle of organisms (Jentsch et al., 2007). Although each type of temperature variation is likely to affect populations in different ways, all may lead to plasticity in reproductive traits that affect fitness, with likely negative consequences for population viability (Loisel et al., 2019; Parratt et al., 2021; Sanghvi et al., 2022; van Heerwaarden & Sgrò, 2021). Whether and how temperature affects population dynamics (Cobben et al., 2011; Duncan et al., 2015; Pärn et al., 2011) or the strength, opportunity, and direction of sexual selection and conflict is attracting increased interest (e.g., García-Roa et al., 2019, 2020; Londoño-Nieto et al., 2023). In the meantime, we are just beginning to understand how population structure and sexual selection can independently influence the ability of populations to respond to varying or increased temperatures. For example, in the Indian meal moth, Plodia interpunctella, sexual selection can increase adaptation rates to a gradual increase in temperature (Parrett & Knell, 2018), while in the fruit fly, Drosophila melanogaster, thermal adaptation happens under precopulatory, but not under postcopulatory, sexual selection (Gómez-Llano et al., 2021). Similarly, metapopulations have been predicted to show different responses in relation to colonization, adaptation, and extinction to changing temperatures, compared to panmictic populations (O’Connor et al., 2012). However, to our knowledge, whether population structure and sexual selection synergistically interact to regulate the ability of populations to plastically respond to altered temperature conditions has been thus far unexplored.
Here, we used an experimental evolution study to expose populations of the seed beetle Callosobruchus maculatus evolving under different intensities of sexual selection and sexual conflict (monogamous vs. polygamous conditions) and different levels of population spatial structure (population subdivision with deme connectivity—that is, metapopulation structure—vs. absence of population spatial structure) to a variety of temperature regimes (control, hot, and stressful). Our aim was to explore whether and how sexual selection and population structure interact to moderate the fitness consequences of altered developmental temperatures, both in terms of moderate increases that persist during the full preadult development period and in terms of mirroring a severe heat event where temperatures rise abruptly during a brief period. Based on previous studies, the alteration of the environment during development time in seed beetles is presumed to have potentially important effects on reproduction (Sanghvi et al., 2022). We measured how altered development temperature regimes affect plastic phenotypic responses in fitness (i.e., in this study measured as focal pair fitness and female lifetime reproductive success; hereafter “LRS”) and underlying fitness-related traits such as larval development time (in both males and females), adult emergence success (i.e., egg-to-adult viability), fertility and lifespan, to then investigate the interplay between sexual selection and population structure in moderating such responses.
Methods
Model system and experimental lines
The seed beetle (C. maculatus) is a pest of stored legumes. The life cycle begins with the female laying eggs on host beans. After hatching, the larva burrows into the bean to feed and, after 21–29 days of full pre-adult development time (depending on environmental conditions), the adult emerges. In this study, we used the 104th generation of a set of selection lines established in 2014 at the Doñana Biological Station and maintained in mung beans (Vigna radiata) at 29 °C, 40% relative humidity, and a 12L: 12D cycle. The experimental evolution setup is explained in detail elsewhere (Canal et al., 2022; Rodriguez-Exposito & Garcia-Gonzalez, 2021). Briefly, the selection experiment consisted of the factorial combination of two selection treatments each containing two levels, namely, mating system selection treatment (monogamy or relaxed sexual selection vs. polygamy or intense sexual selection/conflict) and metapopulation structure (population subdivision—mirroring metapopulation dynamics with moderate gene flow—vs. lack of spatial structure—large panmictic population) (Figure 1). This design resulted in four selection regimes (resulting from the factorial 2 × 2 design). Each selection regime has four replicates, which amounts to 16 selection lines in total (Canal et al., 2022; Rodriguez-Exposito & Garcia-Gonzalez, 2021). All 16 populations underwent two generations of common garden breeding (under polygamy + no spatial structure conditions) before extracting the focal individuals that were allocated to the different temperature treatments (see below). This removes the possibility for any influence of potential parental or grandparental effects associated with the breeding protocol on the results, hence ensuring that the effects seen can be attributed to genetic divergence due to the evolutionary history of individuals according to their selection regime.
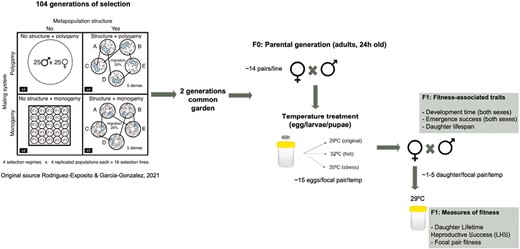
Diagram showing the setup of the selection experiment and the outline of the experimental design. The scheme represents the protocol followed for each of the 16 selection lines.
Experimental design and procedures
To test whether sexual selection, population structure, and their interaction facilitate or hamper the ability of individuals to exhibit adaptive plastic responses in fitness and a variety of fitness-associated traits to quick alterations of the environment, we exposed one generation of developing eggs and larvae to three different temperature regimes: 29 °C (control), 32 °C (hot), or 35 °C including a severe heat period of 72 hr at 39 ± 2 °C a week before the predicted emerging time (stressful) (see Martinossi-Allibert et al., 2016) for stressful conditions for the species.
Around 10 days before the expected emergence time of the adults of the second common garden generation (F0), and to obtain virgins, we isolated ~ 100 individual eggs (one single egg/bean) per selection line in Eppendorf tubes with pin-holes in the cap for airflow. Tubes were monitored daily for adult emergence. The day of adult emergence was recorded, and individuals were sexed and provided with a random identification number blind to the experimental evolution treatment. Animals were maintained in their tubes for another 24 hr to attain sexual maturity. After that, 14 females/lines were randomly selected and placed individually in Eppendorf tubes, each containing a randomly chosen male of the same age and same selection line, to copulate (hereafter we will refer to each of these female/male pairs as “focal pair”). We ensured that copulation took place by checking that males mounted and inserted the aedeagus into the female and remained in this position for around three minutes. Once copulation ended, females were individually placed in a 30-ml plastic container filled with ad libitum beans (approx. 80 beans). After 48 hr, females were removed from the container and the beans with a single egg laid on them (C. maculatus females typically lay a single egg per bean when uninfested beans are available) were individually placed in Eppendorfs. For each focal pair, we isolated around 45 beans with individual eggs, which were randomly allocated to the three temperature treatments (mean ± SD = 14.027 ± 1.875 eggs per focal pair and temperature level, hereafter “temperature-by-focal pair batch”). After a week, we checked the Eppendorfs daily for the presence of emerged adults (F1), and we recorded the day of adult emergence (see Figure 1 for experimental protocol).
On this generation of emerged adults (F1), we measured:
- Development time: number of days elapsed from the mother’s copulation to beetle emergence. Although females were housed with the beans for 48 hr, we considered that first day as the starting point for all samples; but note we cannot completely rule out an error of up to 1 day in this measure.
- Emergence success: number of eggs that successfully completed the development and emerged as adults in each batch. Both development time and emergence success were checked every day for 10 additional days after the last individual emergence. Once the 10 days had elapsed, we considered the development unsuccessful, and the beans were removed from their containers and frozen.
To explore whether the evolutionary regimes moderated the individual fitness of animals exposed to increased temperatures, we measured the LRS of a sample of the daughters (F1 emerged from the three temperature treatments). We randomly selected 1–5 daughters per focal pair and temperature treatment (mean ± SD = 2.626 ± 1.417), provided a random identification number, and housed the animals in their corresponding individual Eppendorf tubes and temperature treatment for 24 hr to attain sexual maturity. The variation in the number of daughters was due to the number of beetles that emerged at each temperature, which limited the availability of daughters (see Results). About 24 hr after emergence, we added a non-sibling male of the same age, selection line, and temperature treatment to the daughter's Eppendorf for mating. Once the copulation ended, daughters were housed in a container with ad libitum (c. 80–90) mung beans at 29 °C to lay eggs. We measured single-mated daughter LRS due to its feasibility and following previous studies in this species, but note that since males and daughters came from the same evolutionary regime, it is likely that the male contributed to some extent to the daughter's LRS. We placed all the daughters at the original temperature since the aim of the experiment was to explore how an increase in temperature during development, rather than during the adult stage, affected fitness.
Daughter survival was monitored daily, and lifespan was recorded as the number of days each daughter survived after mating, which took place when they were 1 day old. Once they died, the daughters were removed from the container. The containers with the beans were kept in a climatic chamber (29 °C) for 28 days after the death of the daughter to ensure the emergence of all offspring produced by her—even the offspring from eggs laid in the latest days of life of the daughter. The beans were then frozen for at least a week before counting the number of emerged offspring (F2) as a measure of the LRS of the daughters (from F1). Females had an average lifespan of ~8 days so, considering this lifespan and the time needed for larval development, our approach ensures that individuals from the next generation have not copulated and produced new adults before freezing the vials, avoiding the inclusion of individuals from the next generation in the measures of LRS. The only identification for each daughter and egg container was a random number (from 1 to n), so all measures were taken blindly to the selection line and the temperature treatment.
Statistical analyses
We run general and generalized linear mixed models (LMM and GLMM) using the packages lme4 and glmmTMB to assess the effect that the manipulation of sexual selection opportunity/intensity and population structure have on the ability of individuals to cope with rising temperatures. All models included sexual selection (polygamy vs. monogamy), population structure (subdivided vs. undivided), and temperature (control 29 °C, hot 32 °C, and stress 35 °C with severe heat event) and their three-way interaction as fixed factors. Both selection line and focal pair identity—for variables with more than a data point per focal pair—(F0 generation female–male pair) were added as random factors in all models. In addition, we crossed line identity with temperature and repeated the models (results of models can be found in the Supplementary Information file, Supplementary Tables S1–S4, Supplementary Figures S1–S4) by fitting line identity as random intercept and random slopes to avoid type I error inflation (Arnqvist, 2020). In most cases, results from both analyses were qualitatively identical, but note that when they did not do so, we noted so and proceeded to interpret the results with caution.
When the three-way interactions were nonsignificant, we re-ran the models to explore the two-way interactions between the three fixed factors. If the two-way interactions were nonsignificant we then simplified the models and removed the interactions to interpret the main effects. For consistency when reporting the results, we first report significant effects (independently of whether these stem from interactions or main effects) and then nonsignificant results for each of the variables analyzed. We tested the statistical estimates of the model variables using the summary function in the lme4 package. The p-values were extracted from models run with maximum likelihood and the parameter estimates from models using restricted maximum likelihood.
Females and males in C. maculatus differ in several aspects of their life history (Canal et al., 2022; Hallsson & Björklund, 2012), including development time (Iglesias-Carrasco et al., 2020a) (in our study development time of males [i.e., sons] was significantly shorter than that of females [i.e., daughters, p < .001]). Therefore, we explored the effect of the evolutionary regime and temperature on development time independently for each sex. Even though we ran GLMM with Poisson and negative binomial errors, the best-performing models were LMMs with Gaussian error structure after log-transforming development time.
Emergence success was analyzed in two steps. First, we explored whether temperature treatment led to a different number of temperature-by-focal pair egg batches with successful emergence (i.e., the groups of eggs laid by a mother and housed at each temperature). To do this, we created a binomial variable where 0 represents those batches in which none of the eggs emerged as adults, and 1 represents those in which at least one of the eggs led to an adult. In a second step, we explored how evolutionary regimes and temperature variation affected the batches that showed successful emergence (i.e., any batch with at least one successfully emerged adult). In this case, we run a GLMM with a binomial model using the cbind function to explore emergence success (number of adults emerged; number of eggs resulting in no adult emergence). We checked for overdispersion of residuals to ensure the suitability of the models.
We explored the effects of sexual selection, population structure, and temperature treatment on daughter fertility, LRS, and focal pair fitness in various steps.
First, we tested whether temperature affected the fertility of daughters using a Binomial model (0 representing infertile daughters, i.e., with no offspring; 1 representing fertile daughters, i.e., producing at least one adult offspring). Since the number of daughters available for testing fertility was low in the stressful temperature (see Results), we did not have enough statistical power to inspect a three-way interaction. Therefore, we only explored the effects of two-way interactions between temperature and population structure, and temperature and sexual selection, on fertility.
Second, we focused on fertile daughters to explore whether the combination of selection regimes and temperature affected the number of adult offspring produced along the daughter's lifespan (LRS). Since the number of fertile daughters in the stressful temperature group was even lower than those available for the tests of fertility (see first paragraph in Results), which resulted in a lack of replication for some evolutionary line replicates, we discarded this temperature level from the main model exploring the effects of temperature on LRS. We ran a LMM with a Gaussian error distribution.
In order to explore the effect of the stressful temperature on LRS, we calculated the mean LRS in each of the temperature, sexual selection, and population structure combinations and ran a Kruskal–Wallis model with temperature as an explanatory factor.
Lastly, we calculated focal pair fitness (e.g., each of the female–male pair copulated in F0) as a compound measure of fitness by multiplying the mean LRS of the daughters from each focal pair by the relative emergence of the corresponding focal pair. Again, we discarded the stressful temperature from the analyses due to the low sample size. We used an LMM with Gaussian error distribution.
We also explored the effect of the evolutionary regimes and temperature on the lifespan of the daughters used for LRS measurements. We explored the fit of models with different model error distributions, but again the best-performing models were those in which the log-transformed variable was modeled using an LMM with Gaussian error.
Results
Throughout the experiment, the sample size fell to different extents across temperatures. While we started the experiment with a similar number of eggs reared at the three temperature treatments (ncontrol = 2,095; nhot = 2,030; nstress = 2,005), the temperature had a very strong effect on the number of emerged adults overall (ncontrol = 1,607 [79%]; nhot = 1,444 [71%]; nstress = 265 [13%]). Among the emerged daughters, 365, 396, and 74 were mated in the control, hot, and stressful, treatments, of which, 14 (4%), 40 (10%), and 12 (16%), respectively, were nonfertile and did not lead to adult offspring.
Daughter development time was affected by the three-way interaction between sexual selection, population structure, and temperature. In monogamous lines, under control and hot temperatures, there was no effect of population structure on the development time of daughters (estimate ± SE = 0.001 ± 0.012, t-value = 0.121, p = .909), while daughters reared at stressful temperatures took longer to emerge when evolving in undivided populations than when in subdivided populations, or compared to control females (estimate ± SE = 0.088 ± 0.027, t-value = 3.303, p = 0.001, Figure 2A, Supplementary Figure S1 per line). In polygamous lines, in contrast, the development time of daughters was similar in subdivided and undivided evolutionary regimes in the three temperature treatments (all p-values > .075). However, the three-way interaction was only marginally significant when the line replicate was crossed with temperature in the model (p = .057, Supplementary Table S1). Son development time was affected by the two-way interaction between sexual selection and population structure (estimate ± SE = 0.068 ± 0.034, t-value = 1.988, p = .037, Figure 2B, Supplementary Table S1), but no effect of temperature was observed (i.e., no three-way interaction).
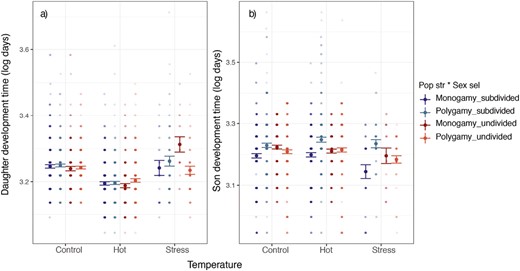
Effect of temperature and the evolutionary regimes (combination of sexual selection and population structure selection treatments) on the development time of (A) daughters and (B) sons. Control = 29 °C; hot = 32 °C; stress = 35 °C ± 72 hr severe heat event. Figure shows means and SEs.
We found that the number of temperature-by-focal pair egg batches that produced at least one offspring was lower when housed at stressful temperatures than when housed at the control and hot temperatures (infertile batches: ncontrol = 1 out of 147 [0%]; nhot = 1 out of 148 [0%]; nstress = 84 out of 142 [59%]; estimate ± SE = −5.633 ± 1.054, z-value = −5.345, p < .001). For batches with successful emergence, we found a statistically significant two-way interaction between population structure and temperature treatment on emergence success. In general, batches in subdivided populations had a lower proportion of emerged individuals in the control and hot temperatures, but an increased emergence success in the stressful temperature (estimate ± SE = 0.480 ± 0.189, z-value = 2.537, p = .011), compared to batches from undivided populations (Figure 3, Supplementary Figure S2 per line, Supplementary Table S2). We note the high variance and small sample size in the stressful temperature treatment, which could potentially affect the estimation of emergence success, so we interpret the two-way interaction with caution. We did not find a significant effect on the emergence of either the three-way interaction between sexual selection, population structure, and temperature, not in the other two-way interactions or main effects.
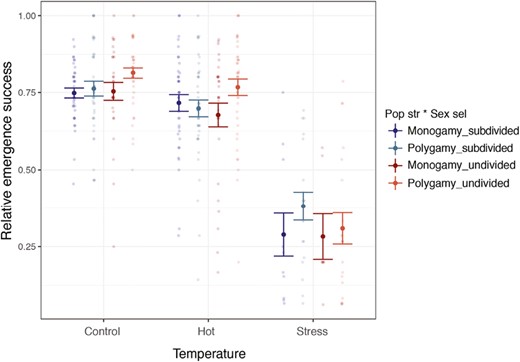
Effect of temperature and the evolutionary regimes on adult emergence success in each temperature-by-focal pair batch. This figure shows the proportion of emerged individuals (i.e., emergence success). Nevertheless, the model used for analyses was performed using a binomial error distribution (cbind function of emerged/nonemerged individuals per batch; see Methods section for more details). Control = 29 °C; hot = 32 °C; stress = 35 °C ± 72 hr severe heat event. Figure shows mean and SE.
There was a statistically significant two-way interaction between population structure and temperature in the number of infertile daughters. In subdivided populations, infertility was higher in both the control and hot temperatures than in the stressful temperature (estimate ± SE = 2.562 ± 1.015, z-value = 2.524, p = .012), while there were no differences in undivided evolutionary regimes. We did not find a significant two-way interaction between sexual selection and temperature treatment on daughters’ fertility (p > .823). However, the reduced model showed that fertility was higher in polygamy than in monogamy (p = .029), while lower in hot and stressful temperatures than in the control (both p-values < .029).
We found a statistically significant three-way interaction between sexual selection, population structure, and temperature on fitness measured as daughter LRS (Figure 4A, Supplementary Figure S3A per line, Supplementary Table S3). In monogamy, daughters from subdivided populations had lower LRS when reared in hot than in control temperatures (estimate ± SE = −7.836 ± 3.027, t-value = −2.589, p = .010). In the polygamous lines, in contrast, there was no effect of the two-way interaction between population structure and temperature on daughter LRS (p = .191). Nonetheless, the three-way interaction was marginally significant when line identity was crossed with temperature (p = .054, Supplementary Table S3), so we interpret this result with caution.
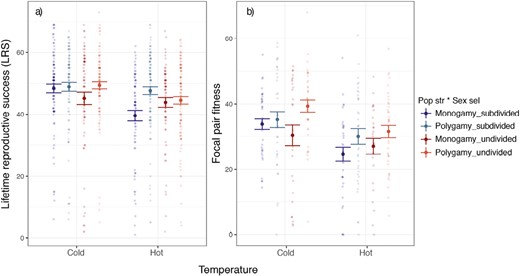
Fitness results. Effect of temperature and the evolutionary regimes (combination of sexual selection and population structure selection treatments) on (A) number of adult individuals produced by each daughter during her entire lifespan (i.e., lifetime reproductive success) and (B) focal pair fitness, calculated as the product of the mean LRS of the daughters from each focal pair (i.e., each of the female/male pairs of F0) by the relative emergence of the corresponding focal pair batch. Control = 29 °C; hot = 32 °C. Figure shows means and SEs.
Kruskal–Wallis analyses showed that independently of evolutionary history, daughters reared at stressful temperatures had, on average, lower LRS than those at control temperatures (KW X2 = 7.654, df = 2, p = .022). We re-ran an ad hoc model to ask if sexual selection hampers/facilitates adaptation to different temperatures in undivided populations. However, we did not find any effect of the two-way interaction between temperature and the sexual selection treatment on LRS (p = .079).
We found that, in general, focal pair fitness was lower in monogamy than in polygamy (estimate ± SE = −6.564 ± 2.666, t-value = −2.462, p = .026), and in the hot temperature than in the control (estimate ± SE = −6.527 ± 1.458, t-value = −4.476, p < .001, Figure 4B, Supplementary Figure S3B per line, Supplementary Table S3). However, we did not find significant two-way interactions between sexual selection, population structure, and temperature in moderating focal pair fitness responses.
The daughter's lifespan was affected by the three-way interaction between sexual selection, population structure, and temperature (Figure 5, Supplementary Figure S4 per line, Supplementary Table S4). In polygamous lines, daughters from divided lines lived longer when reared at stressful temperatures than when they were reared at control and hot temperatures (estimate ± SE = 0.117 ± 0.053, t-value = 2.186, p = .029). In monogamous lines, in contrast, temperature and population structure interaction did not affect the daughter's lifespan (p-value > .099). Again, this interaction was marginally significant when line identity was crossed with temperature (p = .057, Supplementary Table S4).
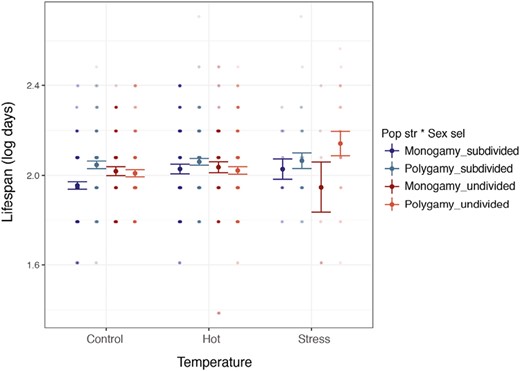
Effect of temperature and the evolutionary regimes (combination of sexual selection and population structure selection treatments) on daughters’ lifespan. Control = 29 °C; hot = 32 °C; stress = 35 °C ± 72 hr severe heat event. Figure shows means and SEs.
Discussion
Sexual selection is expected to promote adaptive plastic responses to novel environmental conditions, but it can also hamper adaptation due to sexual conflict and sexually antagonistic selection (Bonduriansky & Chenoweth, 2009; Cally et al., 2019; Cox & Calsbeek, 2009; Parrett et al., 2019). Nevertheless, studies have traditionally focused on examining the role of sexual selection in maintaining population viability using relatively simple ecological/population/demographic scenarios. This limited focus is starting to broaden, as we are starting to realize that ecological and demographic factors can lead to changes in the intensity of sexual selection and sexual conflict (Martínez-Ruiz & Knell, 2017; McDonald, 2023; Perry & Rowe, 2018; Rodriguez-Exposito & Garcia-Gonzalez, 2021), which might potentially affect the capacity of populations to respond to novel conditions. Here, we explored whether a specific ecological and demographic factor, population structure, moderated the effect of sexual selection in promoting adaptive plastic responses to increased temperatures in fitness and other fitness-associated traits in the seed beetle C. maculatus. As expected, increased temperatures had strong detrimental effects on fitness traits—LRS and focal pair fitness—overall. Interestingly, however, population structure and sexual selection interacted to shape such responses in complex and trait-specific ways. Population structure exacerbated the negative impact of warm temperatures, but sexual selection seemed to buffer the negative influence of population subdivision on plastic adaptive responses. Below we discuss each result in detail, but our findings generally highlight the importance of considering population structure in studies exploring the potential for sexual selection to promote/hamper adaptation.
The interplay between sexual selection and population structure
Previous studies have focused on studying the potential for sexual selection as an evolutionary force facilitating or hampering plastic adaptive responses to novel environmental conditions (e.g., Cally et al., 2019; Candolin & Heuschele, 2008; Doherty et al., 2003; Martinossi-Allibert et al., 2018; Servedio & Boughman, 2017), with mixed results. The results in our study seemed to provide no support for the notion that sexual selection in isolation moderates the plastic phenotypic responses in fitness and fitness-associated traits to increased temperatures, which would add to similar findings revealing neutral effects of sexual selection when individuals face novel conditions (Candolin & Heuschele, 2008; Holland, 2002; Martinossi-Allibert et al., 2019; Rundle et al., 2006). Importantly, however, we found that, when considering population structure, sexual selection influenced the response to increased temperatures in daughter fitness in terms of LRS. Specifically, our results show that daughters from populations evolving under relaxed sexual selection (monogamy) and metapopulation structure exhibited reduced LRS when exposed to increasing temperatures, while there was no effect of population structure on fitness in populations that had evolved under strong sexual selection (polygamous selection lines). Although there is some scope for fecundity selection to differ between our polygamous and monogamous lines (see Canal et al., 2022), we can largely rule out LRS variation in our study to be the consequence of fecundity selection rather than sexual selection. This is because (a) during the maintenance of the lines all females were treated in the same way and we did not promote directional selection towards the more fecund females, and (b) a recent study using the same lines (authors, unpublished) did not find differences in female fecundity (i.e., number of eggs laid) between females from polygamous and monogamous lines. Interestingly though, that same study found that the male sexual selection regime influenced female fecundity through male effects, such that standardized females from outside the selection experiment mated to males from the polygamous evolutionary lines were more fecund than standardized females mating with males from monogamous lines. These lines of evidence suggest that potential variation in fecundity is the result of sexual selection on males, for example, if sexually selected males convey larger direct benefits to females or impose higher costs to females through the stimulation of enhanced oviposition rates. It could also be that sexual selection could somehow intensify male mate choice on larger, more fecund females, which would likewise imply fecundity selection as a by-product of sexual selection. Nevertheless, as mentioned, there is no evidence for differences in female fecundity between polygamous and monogamous lines, which argues against the role of direct fecundity selection on female LRS.
Therefore, our findings suggest that sexual selection can buffer the negative consequences of metapopulation structure when individuals are exposed to an environmental challenge, by allowing surviving individuals to maintain a high reproductive output. Strikingly, although we found that focal pair fitness was lower in monogamy than in polygamy and in the hot temperature than in the control, we did not find any interaction between sexual selection and temperature, suggesting that sexual selection does not buffer the negative effects of increased temperatures on focal pair fitness. Since our measure of focal pair fitness is the combination of adult emergence and LRS, this suggests that high temperatures could affect population viability by reducing egg-to-adult viability in a specific generation. However, the results from daughter LRS show that sexual selection could help buffer, to some extent, this negative effect, with polygamous populations recovering quicker than monogamous ones due to higher female reproductive output. Nonetheless, an open question is whether such rescue by sexual selection would be enough to ensure the viability of metapopulations exposed to stressful conditions in the wild. A key area for future research would be the study of whether sexual selection is able to rescue small populations living in marginal habitats, with limited ability to adapt (e.g., if they receive most immigrants from larger populations, impeding local adaptation). Simulation studies have already suggested that sexual selection may delay the extinction of small demes (Yasui & Garcia-Gonzalez, 2016), but theoretical, field, and comparative studies (for example, comparing long-term population dynamics of sexually selected vs. monogamous species) are needed. Likewise, a promising future research avenue in this context is to explore the effect of thermal stress on male reproductive traits (in addition to female LRS), since females and males are known to respond in different ways to stress (e.g., Breedveld et al., 2023; Martinossi-Allibert et al., 2016).
Our results also suggest that population subdivision alone constrains the ability to plastically respond to novel environments—an idea evidenced by the significant influence that population structure has in moderating how a range of fitness-associated traits, and not only fitness itself (i.e., LRS and focal pair fitness), responded to temperature. In general, individuals from subdivided lines (i.e., metapopulations) showed lower LRS, as well as reduced emergence success when exposed to increased temperatures than those from undivided lines. One possible explanation is that exposure to novel developmental thermal conditions in subdivided populations leads to potential maladaptive plastic changes in the allocation of resources to different life-history traits. Because investing in reproduction often comes at a cost of survival (Hammers et al., 2013; Kirkwood & Rose, 1991; Stearns, 1989), the longer lifespan of daughters developed under increased temperatures might result from reduced reproductive effort. However, we cannot discard that the lower LRS in daughters from the hot conditions is the result of changes in the timing of egg-laying along the life of individuals. In seed beetles, eggs laid later during the lifetime of females had lower emergence success than those laid early during their lives (Iglesias-Carrasco et al., 2018a, 2018b). Since daughters in hot thermal conditions live longer, they might have delayed their egg-laying schedules, with detrimental effects on adult emergence. Another explanation for the reduced performance observed in subdivided populations is the softness of selection (Li Richter & Hollis, 2021), and the potential higher accumulation of mutations associated with it. We can discard that any detrimental effect of population subdivision observed is due to differences in effective population size and ensuing inbreeding or drift. Previous studies using these lines have thoroughly explored whether the evolutionary lines differ in their effective population sizes (Canal et al., 2022; Rodriguez-Exposito & Garcia-Gonzalez, 2021). Such tests inform of a negligible difference in the effective population size of polygamous populations compared to monogamous populations, with a slightly lower effective population size for polygamous lines and with overlapping coefficient intervals between the estimates of both evolutionary regimes. Furthermore, additional lines of evidence suggest that there is limited scope for inbreeding and drift effects (discussed in Rodriguez-Exposito & Garcia-Gonzalez, 2021). Interestingly, however, our study suggests that the negative fitness effect of evolving in subdivided populations is in part compensated by polygamy. All in all, our study shows that population structure is a key factor to consider when studying adaptation to novel environments, and that sexual selection may modulate adaptation when there is population spatial structuring.
Thermal conditions
Exposure to increased temperatures, especially to the stressful treatment, had strong detrimental effects in both LRS and in a range of other fitness-associated traits measured, including emergence success and fertility. These results are in accordance with increasing field and laboratory evidence showing that exposure to high temperatures leads to reduced reproductive success and important fitness costs in several taxa, via the alteration of sexually selected traits and behavior, reduced gamete quality and quantity, and increased infertility (Breedveld et al., 2023; García-Roa et al., 2020; Hurley et al., 2018; Iglesias-Carrasco et al., 2020b; Leith et al., 2021; Martinet et al., 2021; Parratt et al., 2021; Paxton et al., 2016; Walsh et al., 2019; Wild et al., 2019). In addition, our results corroborate that fertility thermal limits are lower than critical thermal limits for survival (van Heerwaarden & Sgrò, 2021). More specifically, we find that adult females show lower fertility and LRS, but longer lifespans, when reared at hot and, in particular, stressful temperatures. Our results suggest that a relatively small increase of three degrees in developmental temperature can significantly impact the fertility of a population. These findings add to a growing body of research on thermal fertility limits (Walsh et al., 2019), and to evidence of the critical consequences of thermal limits on population viability and species survival in the face of anthropogenic climate change (Martinet et al., 2021; Parratt et al., 2021). Interestingly, although our finding that female fertility was reduced when exposed to high temperatures agrees with recent studies in C. maculatus (Baur et al., 2022, 2023), such studies also found that sexual conflict moderates the effect of thermal stress on female fertility. The fact that we did not find similar moderating effects might be the result of the methodology used (e.g., females housed with males during the whole life vs. females only housed with males during copulation), the duration and intensity of the heat event, as well as in the timing in the life cycle at the moment of exposure to the different temperature regimes. Hence, since in many species thermal sensitivity differs between sexes, life-cycle stages, and the type of thermal environment that individuals are exposed to (Baur et al., 2022; Sales et al., 2021), it may be expected that the moderating effect of sexual selection and population structure on fertility would differ depending on these factors.
In our study, we found that rearing temperature affected the development time of females, but not of males. The effect was especially obvious in monogamous lines, where daughters from undivided populations took the longest to emerge when exposed to stressful temperatures. The different responses between males and females could be the result of females being more affected by stressful conditions due to the costs of producing larger bodies or due to the increased physiological costs related to longer exposure times to stressful conditions during development (Benito & González-Solís, 2007; Iglesias-Carrasco et al., 2020a; Wikelski & Thom, 2000). Irrespective of the underlying reason, a mismatch in emergence time between females and males can lead to altered sexual interactions and fitness costs, with far-reaching consequences for sexual selection dynamics (including interspecific interactions such as sexual tactics or female mate choice [Boullis et al., 2016; Candolin, 2019; García-Roa et al., 2020]). Males of seed beetles emerge earlier than females (Arnqvist & Tuda, 2010; Iglesias-Carrasco et al., 2020a), which is presumed to be linked to increased fertilization opportunities associated with the advantage of encountering virgin females eager to mate (Rönn et al., 2008) and the high early-life fecundity and female reproductive returns observed in the species (Fricke et al., 2006; Sanghvi et al., 2022). Instead, in several insects, females benefit from longer development times, due to the accumulation of resources for reproduction during the larval stage (Moller et al., 1989; Šešlija & Tucić, 2003). Future experimental studies could provide valuable insight by examining how the emergence time mismatch resulting from elevated temperatures affects the lifetime reproductive success of females and males. In addition, it would be interesting to investigate the possible association between such mismatches and variations in the intensity of sexual conflict, which could subsequently lead to changes in the evolutionary trajectories of populations under different thermal regimes.
In conclusion, our study suggests that sexual selection could, to some extent, be beneficial in fragmented populations with an inherent lower capacity to adapt to novel environmental conditions. Some of the mixed results found in the literature regarding the effect of sexual selection on the adaptation ability of populations to novel environments could be due to effects stemming from variation in population structuring. Broadly, our study underscores that it is critical to consider the ecological and demographical characteristics linked to populations’ evolutionary history if we aim to understand how animal populations will respond to different anthropogenic challenges such as climate change.
Data availability
Data collected during this experiment have been uploaded in DRYAD, https://doi.org/10.5061/dryad.sj3tx96b3.
Author contributions
M.I.C., P.C., R.G.R., and F.G.G. conceived and designed the study; M.I.C., B.T., and M.L. collected the data; E.R.-E. and M.L. maintained the selection lines; M.I.C. analyzed the data and drafted the first version of the manuscript with input from F.G.G.; all authors contributed critically to the drafts and gave final approval for publication.
Funding
This work was supported by the Andalusian Government to M.I.C., and the Spanish Ministry of Economy and Competitiveness (grants CGL2016-76173-P and PID2019-105547GB-I00—AEI/10.13039/501100011033 to F.G.G.; co-funded by the European Regional Development Fund), and a grant from the Spanish Research Council-CSIC (201730I034 to F.G.G.).
Conflict of interest:
The authors have no conflict of interest to declare.
Acknowledgments
We thank Sara Tripodi for her help with the maintenance of selection lines during the earlier generations, Ana Ruiz for her help collecting the data, and the laboratory of climatic chambers at the Estación Biológica de Doñana.
References
Author notes
Shared authorship