-
PDF
- Split View
-
Views
-
Cite
Cite
Alessio N. De Nardo, Jeannine Roy, Sonja H. Sbilordo, Stefan Lüpold, Condition-dependent interaction between mating success and competitive fertilization success in Drosophila melanogaster, Evolution, Volume 75, Issue 8, 1 August 2021, Pages 2014–2026, https://doi.org/10.1111/evo.14228
- Share Icon Share
Abstract
Dietary restriction during development can affect adult body size and condition. In many species, larger (high-condition) males gain higher mating success through male-male competition and female choice, and female condition can affect the extent of both female mate choice and male investment in courtship or ejaculates. However, few studies have examined the joint effects and interplay of male and female condition during both the pre- and the postcopulatory phases of sexual selection. We therefore manipulated the larval diet of male and female Drosophila melanogaster to study how body size variation in both sexes biases competitive outcomes at different reproductive stages, from mating to paternity. We did not find a difference in mate preference or mating latency between females of different conditions, nor any interaction between male and female conditions. However, large males were more successful in gaining matings, but only when in direct competition, whereas mating latencies were shorter for low-condition males in noncompetitive settings. Small males also transferred more sperm to nonvirgin females, displaced a larger proportion of resident sperm, and achieved higher paternity shares per mating than large males. In agreement with existing theory, we suggest that small males might partially compensate for their low mating success by strategically investing in larger sperm numbers and potentially other, unmeasured ejaculate traits, when they do have a mating opportunity.
Sexual selection theory assumes that females typically invest more per offspring and are more selective when it comes to mates, whereas males become mate limited and compete for available mating opportunities (reviewed in Andersson 1994; Kokko et al. 2006). Traits and behaviors associated with females sampling and choosing mates, and with males gaining access to mating opportunities through competition or courtship, often incur substantial costs (Pomiankowski 1987; Reynolds and Gross 1990; Andersson 1994; Cotton et al. 2006b; Rosenthal 2017). Further costs through enhanced investment in fitness traits can arise whenever females mate with multiple males, whose sperm will compete for fertilization (Parker 1970). Although females may grow costly sperm-storage organs that allow them to differentially store or use sperm from competing males (Miller and Pitnick 2003; Ward et al. 2008), males can increase their competitive fertilization success by transferring larger and higher-quality ejaculates (Snook 2005; Simmons and Fitzpatrick 2012; Fitzpatrick and Lüpold 2014). However, spermatogenesis and ejaculates are now widely recognized to be costly (Dewsbury 1982; Van Voorhies 1992; Olsson et al. 1997; Thomsen et al. 2006; Lüpold et al. 2016). Theory therefore predicts that males should allocate their ejaculates strategically (Wedell et al. 2002; Parker and Pizzari 2010). This prediction finds empirical support, with males of many species adjusting their sperm numbers or costly seminal fluid components to the perceived risk and intensity of sperm competition (reviewed in Kelly and Jennions 2011).
The total investment in fitness-related traits is often determined by the phenotypic condition (e.g., body size, age, nutritional, or infection state) of individuals, with high-condition individuals being able to sustain greater costs of investing in sexual traits, and thus gaining greater reproductive success, than individuals of poor condition (Nur and Hasson 1984; Rowe and Houle 1996; Cotton et al. 2004). Consequently, condition should affect reproductive decisions and strategic allocation between costly traits to enhance the investment in those traits with the greatest marginal fitness benefits. Condition dependence of reproductive traits and behaviors is taxonomically widespread for both sexes. For males, condition-dependent expression may include secondary sexual characters (Andersson 1994; Cotton et al. 2004; Emlen 2014) and courtship or calling effort (Galeotti et al. 2005; Judge et al. 2008; Engqvist 2009) or ejaculate/spermatophore size and quality (McGraw et al. 2007; Lehmann and Lehmann 2009; Perry and Rowe 2010; Kahrl and Cox 2015; Macartney et al. 2019). For females, condition has been shown to affect their choosiness between or sexual responsiveness to males (Syriatowicz and Brooks 2004; Wilgers and Hebets 2012; Lerch et al. 2013; Judge et al. 2014; Kelly 2018), or their remating behavior (Schäfer and Uhl 2005; Perry et al. 2009).
Studies examining the fitness consequences of condition typically do so for one of the sexes and/or during either the pre- or postmating episode of selection. However, male reproductive success, for example, is determined by the combination of mating success and competitive fertilization success, with potentially antagonistic interactions between these episodes of selection (e.g., Danielsson 2001). Further, both episodes are influenced by the males’ own competitiveness and any female preferences or biases, which themselves can be condition dependent (e.g., Cotton et al. 2006a). Finally, males may invest differentially in courtship or ejaculates depending on the condition (i.e., breeding value) of the female, and the degree of differential allocation could vary with male condition (Kelly and Jennions 2011). It thus seems important to test how males of varying condition gain mating and fertilization success, and how female condition might influence either of these processes.
A useful system to study the joint effects of male and female condition on different reproductive stages is Drosophila melanogaster. In this species, both sexes mate multiply (Imhof et al. 1998), leading to selection on any male trait that enhances either mating success or competitive fertilization success, or any female trait that gives the female some control over these processes. Multiple mating and the associated behaviors and physiological changes can be costly by shortening lifespan and reducing immune function in both males (Partridge and Farquhar 1983; Cordts and Partridge 1996; McKean and Nunney 2001) and females (Fowler and Partridge 1989; Chapman et al. 1995; Kamimura 2007; Short et al. 2012). Additionally, male mating costs include the enhanced ejaculate investment in response to sperm competition (Pitnick 1996; Linklater et al. 2007; Sirot et al. 2009; Lüpold et al. 2011). These costs might explain why males themselves are choosy (Byrne and Rice 2006; Friberg 2006) and strategically allocate their ejaculate components (Wigby et al. 2009; Lüpold et al. 2011, 2020; Sirot et al. 2011; Garbaczewska et al. 2013), or why males reared on a low-protein diet transfer fewer sperm (McGraw et al. 2007), thereby suffering a disadvantage in competitive fertilization (Amitin and Pitnick 2007; McGraw et al. 2007; Fricke et al. 2008). For females, the potential costs of mate choice (e.g., extended mate assessment sensu Jennions and Petrie 1997; Cotton et al. 2006b) and cryptic female choice (e.g., length of the seminal receptacle; Miller and Pitnick 2002, 2003) could also be considerable, resulting in condition-dependent expression of the traits and processes associated with female choice. For example, low-condition females might be less choosy (Cotton et al. 2006b; Judge et al. 2014) or less able to bias sperm storage and utilization toward preferred males.
Using D. melanogaster as a model organism, we studied the joint effects of male and female condition on male mating success (competitive and noncompetitive) and sperm competitive ability (i.e., sperm transfer, sperm displacement), as well as female fecundity, mate choice, and cryptic female choice. We manipulated the food of developing larvae to systematically vary adult body size, and subjected small and large flies to different experimental mating scenarios to tease apart the contributions of male and female size to different reproductive processes. In D. melanogaster, body size is often used as a proxy for condition due to its sensitivity to environmental conditions during development (e.g., Partridge et al. 1994; Tu and Tatar 2003) and because it is a good predictor of both female fecundity (Byrne and Rice 2006) and male mating success (Partridge and Farquhar 1983; Partridge et al. 1987a,b).
We tested four main predictions about separate effects of male and female condition/size on reproductive outcomes, and two predictions about their interactions. Our four univariate predictions were (1) that male mating success (competitive and noncompetitive) should increase with body size, as demonstrated previously (Ewing 1961, 1964; Partridge and Farquhar 1983; Partridge et al. 1987a,b; Morimoto et al. 2016; Baxter et al. 2018); (2) that high-condition (i.e., large) males should achieve greater paternity shares by affording to produce more competitive ejaculates than their low-condition (small) counterparts (Amitin and Pitnick 2007; McGraw et al. 2007; Fricke et al. 2008); (3) that large, high-condition females should invest more time in mate assessment by waiting longer to start mating (Jennions and Petrie 1997; Cotton et al. 2006b); and (4) that females should bias sperm ejection (i.e., a mechanism of cryptic female choice; Manier et al. 2010; Lüpold et al. 2013) to predominantly retain the sperm of large males, because these are preferred (Dukas 2005) .
Further, our two predictions for the interactions were (5) that if females can choose between mates of different sizes, large, high-condition females should have a stronger propensity to choose a larger male (or discriminate against a smaller one) than would small, low-condition females, because they can better afford to invest time and energy in mate sampling, and (6) that if cryptic female choice is costly (e.g., Miller and Pitnick 2002, 2003), large females should be able to have a stronger influence on competitive fertilization success than small females, manifested by a stronger bias in fertilization success in favor of their preferred mate, possibly mediated by sperm × female interactions (Miller and Pitnick 2002; Lüpold et al. 2020).
Material and Methods
EXPERIMENTAL SUBJECTS
The experiments were conducted using the LHm strain of Drosophila melanogaster. All flies used were genetically modified to express either red (RFP) or green fluorescent protein (GFP) in their sperm heads (Manier et al. 2010). The GFP flies also ubiquitously expressed GFP, permitting paternity assignment at the organismal level following competitive matings (Lüpold et al. 2012, 2013). All fly cultures were maintained under stable conditions of 24°C, 60% humidity, and a 12h:12h light:dark cycle.
To enhance the genetically determined variation in body size without manipulating levels of larval competition (and thus socially cued anticipatory plasticity in reproductive investment; Kasumovic and Brooks 2011), groups of 40 first-instar larvae were transferred to vials with either 12 ml of standard fly food (high-yeast treatment, henceforth “H”; see recipe in Table S1) or 6 ml of fly food containing only 25% of the normal yeast concentration to reduce the protein content (low-yeast treatment, henceforth “L”). Within 8 h of emergence, adult flies were collected under ice anesthesia (Barron 2000; Champion de Crespigny and Wedell 2008) and kept in groups of either 10–15 virgin females or 15–20 sexually naïve males, respectively, in vials with standard fly food.
SEQUENTIAL MATING
To study the univariate and interactive effects of male and female condition on pre- and postmating sexual selection, females of high or low condition were sequentially mated to two males that contrasted in both condition and sperm-tag color.
In a fully factorial design (Fig. 1), 3- to 5-day-old H and L females were mated to either a H or L male (each either GFP or RFP, 3- to 5-day old) and, 2 days later, to a male of the opposite treatment and sperm-tag color. All pairs that did not remate were given additional opportunities on the two subsequent days. Flies that did not remate after three opportunities, or that received sperm from only one male (i.e., no fertilized eggs before remating or no offspring/sperm detected from first or second male), were excluded. Successful double mating was recorded for 83% of high- and 73% of low-condition females (χ2 = 14.05, df = 1, P < 0.001). However, remating rates did not differ significantly between females that were mated to a low- (80% remating) or a high-condition first male (76% remating; χ2 = 2.37, df = 1, P = 0.12).
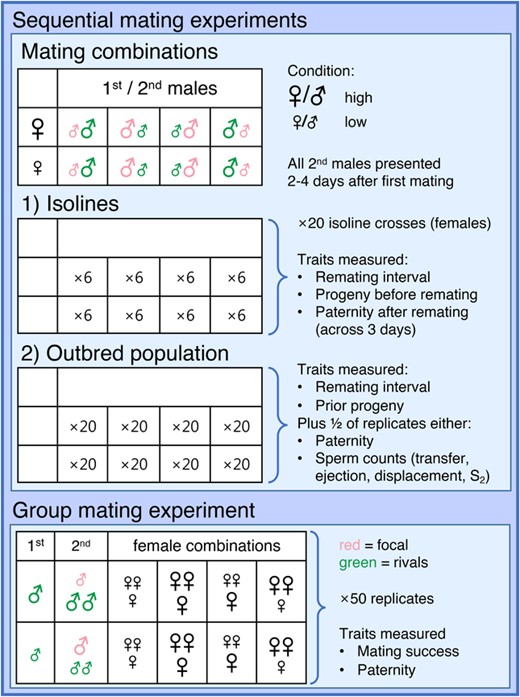
Illustration of experimental design for the two sequential mating experiments (top panels) and the group mating experiment (bottom panel). The different panels indicate the mating combinations, replication, and traits measured for each combination. The general experimental design for the sequential matings applied to both the isoline and population experiments, with the corresponding cells below thus only indicating the different levels of replication (for each of the 20 female isolines or in total, respectively).
This experiment was conducted twice using different fly populations (Fig. 1). In the first round, the experimental flies were generated by pairwise crosses between independent isogenic lines (henceforth “isolines”) of the same sperm-tag color (for details, see Lüpold et al. 2012, 2013). This approach created heterozygous, but genetically identical flies, allowing us to expose the same genotypes to different experimental conditions while minimizing inbreeding effects compared to pure isolines. Experimental females of both larval conditions were derived from each of 20 genetically independent RFP crosses, and the males from one GFP and one RFP cross, respectively. Standardized male genotypes were used because the focus of this first experimental design was on the female genotypic and condition-dependent effects. In six replicates per female line, treatment combination, and order of male genotypes (i.e., sperm color), females were sequentially mated to two males and then allowed to oviposit in fresh vials for three days. Immediately after mating (males) or oviposition (females), experimental flies were frozen for later measurements of thorax length as a proxy of body size. The offspring of the oviposition vials were reared for paternity assignment.
In a second round, the general procedure was repeated, but this time using experimental flies from outbred populations of either sperm-tag color instead of isolines (Fig. 1). Half of the replicate trials were treated as above and used for paternity assignment; in the other half, females were transferred to individual wells of 24-well cell-culture plates immediately after their second mating, covered with a coverslip and held in place using dots of rubber cement in two corners. These females were checked every 10 min for up to 5 h for the ejection of the copulatory plug and a mass containing both displaced first-male and excess second-male sperm (Manier et al. 2010; Lüpold et al. 2013). This sperm ejection marks the end of the sperm displacement phase, and both its timing and extent have been shown to affect relative sperm storage (i.e., proportion of all stored sperm derived from the second male, S2) and competitive fertilization success among males (Lüpold et al. 2013, 2020). The females and ejected masses were frozen separately for later quantification of sperm stored in the female reproductive tract or ejected under an Olympus BX50 fluorescent microscope with a GFP/RFP dual filter.
GROUP MATING
Because the two sequential mating scenarios precluded premating male-male competition and female choice among rival males, interactions between males and females of contrasting condition were also examined in a group setting. Given that males with GFP-tagged sperm also ubiquitously express GFP (whereas the RFP had no external marker), mating success and P2 of a focal RFP male relative to his GFP competitors could be easily determined based on the presence and proportion of non-GFP offspring, respectively.
To examine the effect of premating sexual selection in mating trials, as well as P2 in case of successful mating by the focal male, flies of the same two outbred populations were used as in the second round of the sequential-mating experiment, but this time mated in groups (Fig. 1). Two to 3 days after eclosion, groups of approximately 50 virgin RFP females and 75 GFP males were combined in bottles in a fully factorial design with respect to their larval treatment. All four combinations of male and female conditions were replicated across three bottles (i.e., N = 150 female per combination). After 6 h, when females were considered to have mated given the surplus of males, the flies were separated by sex and mating treatment. Males were kept in vials of 15–20 individuals of the same mating treatment. By contrast, females were housed in groups of three in four different combinations between female larval treatments (3H, 3L, 2H/1L, or 2L/1H) for each first-male treatment (H or L). Each of these eight different combinations was replicated across N = 50 vials.
Three days after these initial matings, two rival GFP-males of the same larval treatment as the females first mates and one focal RFP-male of the opposite larval treatment were added to each vial of mated females. All matings were observed, and their start and end times were recorded. After the third mating in a vial, the females were separated and allowed to lay eggs in individual vials for 3 days. Groups with fewer than three matings during the 6-h observation period were excluded from the analysis. The percentage of groups with three matings did not vary in response to different female compositions (χ2 = 1.8, df = 3, P = 0.61) but depended on the composition of the three males, with 29% of groups with two low-condition rivals and 45% of groups with two high-condition rivals achieving three matings (χ2 = 10.9, df = 1, P < 0.001). For those with three matings, we explicitly assumed that each female mated once (given the typical remating interval of 2–4 days). After eclosion of the adult offspring, the presence of focal-male (RFP) offspring provided information on his mating success and, if he mated, on his paternity share (P2). Due to this indirect assessment, we cannot exclude the possibility that some focal matings could have been missed if they did not result in any offspring, but these cases are rare.
STATISTICAL ANALYSES
All statistical analyses were performed in R version 3.6.2 (R Core Team 2019). All graphical representations were created using the ggplot2 package (Wickham 2016). The effects were extracted from the models using the effects package (Fox and Hong 2009). The continuous predictor variables were scaled and centered in all analyses and the sperm-tag color of the males was accounted for in all models. Experiments were conducted in single blocks; hence, no block effects were included in our statistical models.
The effect of larval treatments on body size was analyzed in a linear mixed-effects model (LMM) using the lme4 package (Bates et al. 2014). For mating latency, we conducted a Cox's proportional hazard analysis, using the survival package (Therneau 2020). The remating interval was analyzed using an ordinal logistic regression model as implemented in the ordinal package (Christensen 2019), with the remating day coded as an ordered factor.
Finally, all count and proportion data were analyzed using generalized linear mixed-effect models (GLMM) using the lme4 package, with an observation-level random effect (OLRE) to account for overdispersion (e.g., Harrison 2015). Generally, all predictors that were a priori hypothesized to be biologically relevant were kept in the final model, even if nonsignificant. Only interactions with P-values >0.1 were removed if their exclusion improved model fit based on likelihood ratio tests. Where necessary, the mixed-effects models were optimized using the optimx package (Nash 2014).
Results
SEQUENTIAL MATING WITH ISOLINES
The low-yeast larval food treatments resulted in significantly smaller body sizes (thorax length), but to a varying degree between the genotypes both in males (treatment least-squares mean ± SE = 0.90 ± 0.002 vs. 0.97 ± 0.002 mm; treatment: F1,836 = 789.75, P < 0.001; color: F1,836 = 12.27, P < 0.001; interaction: F1,836 = 7.48, P < 0.01; Fig. S1) and females (1.00 ± 0.002 vs. 1.11 ± 0.002 mm; treatment: F1,828 = 1442.35, P < 0.001; isoline: F19,828 = 3.78, P < 0.001; interaction: F19,828 = 1.96, P < 0.01; Fig. S1). As thorax length varied substantially within treatments, we performed our analyses twice, once using thorax lengths and once using larval treatment as predictors. In most analyses, both approaches revealed similar results. For simplicity, and because the continuous body size variation tended to explain more of the variance, we focus our results and discussion on the analyses based on thorax length (except where results differ between models) but report all results for the larval treatments in the Supporting Information tables.
To test the effects of male and female body size on the latency to mating (at the first mating), a Cox's proportional hazard analysis was performed with male size/treatment, female size/treatment, and the color (i.e., genotype) of the male as predictors. Neither male nor female size had a significant effect on mating latency (both P > 0.10; Table S2), but in the treatment model low-condition males mated significantly faster (hazard ratio = 1.2, z = 2.58, P < 0.01; Table S3) than high-condition males with a hazard ratio of 1.2, which means a 20% higher chance of mating in the next minute for low- compared to high-condition males. In both models, the RFP-males mated faster than the GFP-males (z > 5.2, P < 0.001).
Next, the effects of female and male condition on the remating interval were tested in an ordinal logistic mixed-effect model (N = 592) with female line as a random effect (N = 20, variance = 0.073) and controlling for the mating order between RFP and GFP males. The possible remating days (2–4 days) were treated as ordinal factor levels. None of the predictors in the body size model had a significant effect on the remating interval (Table S4), but in the treatment model (N = 732) low-condition females had a longer remating interval than high-condition females (z = 2.36, P = 0.018) and the remating interval was longer for females that had mated with a low-condition male during their first mating (z = 2.53, P = 0.011; Table S5).
By contrast, large females produced offspring at a higher rate than small females, in that a Poisson GLMM (N = 733) revealed a significant increase in number of progenies produced between sequential matings with female size (z = 12.13, P < 0.001), while controlling for the effect of varying remating intervals (z = 11.88, P < 0.001). However, in the same model there was no significant effect of first-male size (z = −1.29, P = 0.20; Tables S6 and S7 for treatment model).
Finally, a binomial GLMM (N = 447) on second-male paternity (P2), with female line (N = 20) as a random effect, indicated that smaller second males gained relatively higher paternity shares than larger second males (z = −2.79, P < 0.01; Fig. 2) and that P2 was higher where first males were larger (z = 1.99, P = 0.045; Table S8). The same pattern was revealed in the treatment model (Table S9). Note that these analyses included only observations for which successful sperm transfer by both males was confirmed (i.e., progeny before remating and/or mixed paternity thereafter).
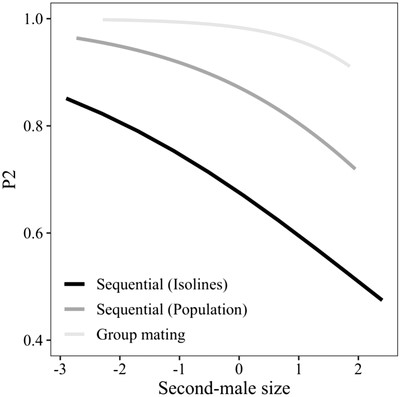
The effect of second-male body size (scaled and centered) on second-male paternity (P2) in three independent experiments.
SEQUENTIAL MATING WITH OUTBRED POPULATIONS
As in the isoline experiment, larvae developing in high-yeast medium resulted in significantly larger adult flies than those reared under low-yeast conditions for both males (0.90 ± 0.002 vs. 0.95 ± 0.002 mm; treatment: F1,713 = 322.06, P < 0.001; color: F1,713 = 12.27, P < 0.001; interaction: F1,713 = 3.35, P = 0.07) and females (1.02 ± 0.003 vs. 1.125 ± 0.003 mm; treatment: F1,326 = 741.44, P < 0.001; Fig. S1).
Our first analysis explored the size effects on the number of sperm transferred by the second male to mate, controlling for the number of first-male sperm still residing inside the female reproductive tract at remating. In a GLMM (N = 95), the amount of sperm transferred was negatively associated with the size of the second male (z = −1.99, P = 0.046; Fig. 3), and positively with the number of first-male sperm still in storage (z = 3.25, P < 0.001), with no effect of female size (z = 0.59, P = 0.55; Table S10). These results were confirmed by the model with larval treatment as a predictor, except that there was no effect of male treatment on the number of sperm transferred (z = 0.49, P = 0.62; Table S11).
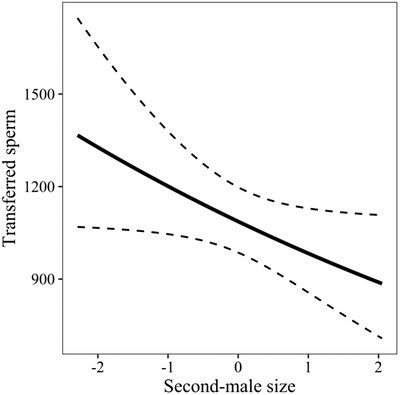
The effect of second-male body size (scaled and centered) on the amount of transferred sperm. The dashed lines indicate the 95% confidence interval.
Next, in a binomial GLMM (N = 95), a higher proportion of the first male's resident sperm was displaced and ejected by large females than smaller females (z = −2.25, P = 0.025). Females also ejected a higher proportion of the first male's sperm when the second male was large (z = −1.97, P = 0.049) and when the second male transferred more sperm (z = 4.81, P < 0.001). Further, albeit nonstatistically significant, a weak interaction between the second males’ size and sperm transferred (z = 1.83, P = 0.067; Table S12) suggested that the proportion of rival sperm displaced was independent of the number of sperm transferred in the case of small second males, but contingent on sperm transfer for large second males (Fig. S2). Except for the number of sperm transferred, none of the predictors had an effect on sperm displacement in the treatment model (all P > 0.1, N = 95; Table S13).
After female sperm ejection, a binomial GLMM (N = 97) revealed that the resulting proportion of second-male sperm in storage (S2) was higher when the second male transferred relatively more sperm (z = 2.01, P = 0.044). However, S2 was lower in larger females (z = −2.39, P = 0.017) and in females with more of the first male's sperm still in storage (z = –6.19, P < 0.001; Table S14, treatment model in Table S15). Further, S2 in the seminal receptacle (N = 97), which reflects the primary source of sperm for fertilization in D. melanogaster (Manier et al. 2010, 2013), was also lower in larger females (z = –1.91, P = 0.056) and when more resident sperm were still present (z = −4.90, P < 0.001). However, the number of sperm transferred by the second male had no effect (z = 0.59, P = 0.56; Table S16, treatment model in Table S17).
Finally, similar to the experiment using isoline crosses, P2 was inversely related with second-male body size (z = −2.11, P = 0.03; Fig. 1 and Table S18). These results were consistent in the model with larval treatments as predictors, except for an additional effect of second-male sperm-tag color (Table S19).
GROUP MATING WITH OUTBRED POPULATIONS
To assess male mating success, each of the three females per vial was scored as having mated with the focal male or not. In a binomial GLMM (N = 353) with an OLRE (variance = 1.1 × 10−10, standard deviation [SD] = 1.0 × 10−5), focal males from the low-yeast treatment (i.e., smaller than their rivals) had a significantly lower mating success compared to their larger high-yeast counterparts (z = –4.29, P < 0.001; Fig. 4).
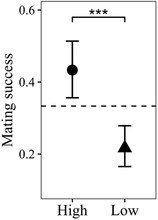
The larval treatment has a significant effect on male mating success. The dashed line indicates the expected mating success for each male if matings were randomly distributed between the three males (i.e., if size did not have any influence on mating success).
Similarly, in a model using the measured body sizes of focal and rival males, as well as those of the females, focal-male mating success was negatively associated with the mean rival-male size (z = −2.53, P = 0.011). However, focal-male size did not significantly predict focal-male mating success in this model (z = 0.95, P = 0.34), possibly due to the presence of two rival males, whose sizes had a stronger influence on the outcome than the size of the focal male.
Among the females that did remate with the focal male (N = 183 across 119 different vials), P2 was analyzed in the group mating context using a binomial GLMM (N = 183) with the vial ID (variance = 0.46, SD = 0.68) and an OLRE (variance = 12.28, SD = 3.5) as random effects. As the approach in this experiment differed from that of the noncompetitive experiments, the thorax length of the first male was unknown, and all remating intervals were 3 days. Thus, only focal-male and female size were included as predictors and found to be negatively associated with P2, albeit not significantly so for female size (focal-male size: z = −3.03, P = 0.002, Fig. 2; female size: z = −1.79, P = 0.07). In the treatment model, only the male treatment was considered, as that of the individual females could not be unequivocally determined after the mating trials due to overlapping size ranges. In accordance with the body size model, here low-condition males also achieved a higher P2 (z = 7.35, P = 0.0018).
Discussion
We investigated the effects of larval food restriction and body size in males and females on various aspects of sexual selection in D. melanogaster and found that (1) large males had higher mating success than small males, but low-condition males mated faster than high-condition males; (2) low-condition males delayed female remating for longer than high-condition males, whereas small males transferred more sperm to nonvirgin females and displaced a larger proportion of resident sperm from previously mated females, leading to higher P2. Overall, small, low-condition males were more competitive in postmating sexual selection. (3) Female size had no effect on mating latency, but low-condition females remated later than high-condition females. (4) Females ejected more first-male sperm when the second male was large, which might indicate a cryptic preference. Further, (5) female condition did not influence the strength of the preference for males of a certain condition and there was no evidence for size-assortative mating; and (6) large females did not eject a higher proportion of small-male sperm than small females did. In fact, large females ejected a lower proportion of first-male sperm than small females, but there was no interaction with male condition.
Our results do not support the prediction that low-condition females allocate fewer resources to mate choice (i.e., they did not initiate mating earlier) or have a weaker preference for high-quality males (i.e., no size-assortative mating was found). However, this lack of differential female preference might be a consequence of the experimental setup and does not necessarily imply a lack of condition-dependent female mate choice altogether. Differential female preferences would be predicted particularly when mate choice is costly (Jennions and Petrie 1997; Cotton et al. 2006b). However, our data suggest that competitive mating success might be primarily explained by male-male competition, as a large-male mating advantage was only shown in a competitive context. If true, selection on, and thus female investments in, premating female choice might be comparatively less important than premating male-male competition or postmating female biases, thereby limiting the scope for condition dependence of premating female mate choice. Consistent with this last prediction, we documented a relatively smaller bias in S2 in large females compared to small females. A similar result has been found at least in one experimental block in Amitin and Pitnick's (2007) study and been indirectly suggested by Lüpold et al.’s (2020) documented interactions between the sizes of females and their sperm-storage organs as well as the competing males’ ejaculate parameters. If larger females have a relatively larger seminal receptacle (Amitin and Pitnick 2007; Lüpold et al. 2016), then they might be able to retain relatively more sperm of the first male, thereby making it more difficult for the last male to displace these sperm despite transferring more sperm in response (Lüpold et al. 2012, 2020).
Absent any interactive effects between male and female size/condition, our study revealed that focal males that were larger than their two standard competitors on average gained approximately 43% of all matings, thus 30% more than the 33% success rate predicted by chance. Consistently, at 22%, the mating success was 33% below random when focal males were smaller than their competitors. This mating advantage of large males is consistent with laboratory and field observations of most Drosophilid species studied so far (reviewed in Pavkovic-Lucic and Kekic 2013) and investigations of a diversity of other taxa (Andersson 1994; Andersson and Iwasa 1996). In D. melanogaster specifically, this advantage can manifest itself by larger males physically dominating smaller ones in aggressive interactions (Dow and Schilcher 1975; Partridge and Farquhar 1983; Baxter et al. 2018), and by delivering more and louder courtship song (Partridge et al. 1987a).
The substantial large-male mating advantage observed under competitive conditions was absent in no-choice trials, in that large males did not initiate mating faster than small males as reported previously (Partridge and Farquhar 1983; Turiegano et al. 2013). Because male Drosophila are unable to force copulations and females thus ultimately determine if and when copulation occurs with a particular male (Spieth 1974; Eberhard 2002), mating latency is often used as a proxy of female preference for a given male (Spieth 1974; Grillet et al. 2006; Hosken et al. 2008; Sharma et al. 2010). To the extent that variation in mating latency indeed reflects female preferences, the lack of a difference between males of different sizes would imply that large males may not have been perceived by females as more attractive. Surprisingly, however, we found that males of the low-yeast treatment mated faster than males of the high-yeast treatment, which suggests that treatment effects other than body size appear to contribute to mating latency. We did not quantify courtship behavior or cuticular hydrocarbons, but these are two variables that could contribute to this difference. If so, our findings across competitive and noncompetitive conditions would suggest that size-dependent mating success might primarily be the result of male-male competition rather than female choice, or that the female preference for larger males becomes more reliable and pronounced when potential mates can be compared directly (e.g., Hoikkala and Aspi 1993; also see Jennions and Petrie 1997). Because the single males presented in our noncompetitive experiments were the first males encountered by our females after sexual maturation, their size could not be assessed relative to that of other males in the population, whereas males in the group vials were directly comparable (Dougherty and Shuker 2015), in addition to the absence versus presence of male interactions themselves. In contrast, low-yeast males were more successful in no-choice scenarios, where no competition between males occurred and females might have been more receptive to a low-yeast male. It is possible that such males are less harmful to females (Pitnick and Garcia-Gonzalez 2002), although it remains unclear whether the risk of male harm can be predicted by the female through other cues than body size.
Although large males secured more matings under competitive conditions, small males achieved higher competitive fertilization success per mating (measured by P2) in all three experiments of our study. If differences in mating success result from winning or losing in social interactions, our findings are broadly consistent with Filice and Dukas's (2019) recent report that males losing in an aggressive encounter subsequently gain higher postmating success, at least as the first of two sequential mates. It seems likely that male D. melanogaster balance their ejaculate investment between their current and anticipated future mating opportunities (Pitnick 1991). Given their disadvantage in acquiring mates, small males would benefit by making the most of any mating obtained, whereas large males should invest less per copulation to partition their sperm and seminal-fluid reserves across their expectedly more abundant mating opportunities (Tazzyman et al. 2009).
Our results of all three experiments deviate from Amitin and Pitnick's (2007) observation of a consistent large-male advantage on P2 when experimentally manipulating male size by larval density compared to nutritional quality in our study (i.e., primarily protein restriction by reducing yeast concentration). The reason for this discrepancy remains unclear, but it seems plausible that adult-male physiology and ejaculate production are differentially affected between food quantity or accumulation of toxic waste via crowding (Botella et al. 1985) and food quality during larval development (in addition to different contexts of larval social interactions between these experiments). For example, varying protein:carbohydrate ratios in the diet greatly influence how insects allocate their resources between reproduction and somatic maintenance (e.g., Lee et al. 2008; Maklakov et al. 2008; Lee 2015) or between pre- and postmating traits (e.g., Morimoto and Wigby 2016). Similarly, ejaculate traits can also vary in their sensitivity to the quantity versus quality of the food consumed by the male (Bunning et al. 2015). If these differences in the experimental manipulation of male and female condition affect the composition of ejaculates or female sperm storage and utilization, then the complex interactions between ejaculate traits within and between competing males, and the morphology and physiology of the female reproductive tract, could ultimately also alter paternity patterns between males (Lüpold et al. 2020).
In our study, the small-male advantage in competitive fertilization was primarily mediated by transferring more sperm and thus displacing a greater proportion of the first male's sperm still residing in the female reproductive tract at remating. However, it appears that small and large males might also differ to some extent in their sperm-displacement ability beyond transferring more sperm. A weak interaction between second-male size and sperm transfer indicated that apart from very large ejaculates, the sperm of small males displaced a higher proportion of first-male resident sperm than those of large males, irrespective of the number of sperm transferred. This interaction suggests that small and large males not only invest differentially in sperm numbers per copulation but potentially also in other, unmeasured ejaculate traits such as sperm motility, sperm viability, or seminal-fluid components that facilitate sperm displacement (e.g., Wigby et al. 2016). Differential investment in seminal-fluid components could also be an explanation for the finding that females first mating with a low-yeast male remated later than those that first mated with a high-yeast male. This also indicates that males might differentially allocate specific components of their seminal fluids, depending on their condition, the mating status of the female, and most likely other factors (e.g., see Wigby et al. 2016). In future experiments it would thus be interesting to examine how the ejaculate composition varies with male size and possibly with the current sperm-displacement ability and anticipated mating potential (e.g., Perry and Rowe 2010).
Antagonistic effects between mating success and competitive fertilization success have also been documented in the water strider, Gerris lacustris (Danielsson 2001), and are indirectly implied in several other species based on differential investments between traits under pre- and postmating sexual selection, respectively. For example, ejaculate characteristics thought to be important in competitive fertilization are negatively associated with quantitative secondary sexual traits such as male body size (Danielsson 2001; Klaus et al. 2011), the expression of sexual weaponry (Kelly 2008; Yamane et al. 2010), or male attractiveness (Evans 2010; Simmons et al. 2010; Engqvist 2011), but also with discrete states such as male social rank (Rudolfsen et al. 2006), prior competitive experience (Pizzari et al. 2007; Filice and Dukas 2019), or alternative mating strategies (Simmons et al. 1999; Vladić and Järvi 2001; Locatello et al. 2007). Negative associations between pre- and postmating sexual traits are further widely reported across species, with several comparative studies documenting evolutionary trade-offs between testes size and traits under premating sexual selection such as the degree of sexual size dimorphism (Fitzpatrick et al. 2012; Dines et al. 2015; Kahrl et al. 2016), weapon or body size (Poulin and Morand 2000; Lüpold et al. 2017), or ornamentation (Lüpold et al. 2019). Yet, at both the intra- and interspecific levels, such negative covariance between pre- and postmating sexual traits or fitness outcomes is far from universal, as positive or no relationships are almost equally widespread (Mautz et al. 2013; Lüpold et al. 2014; Evans and Garcia-Gonzalez 2016). Reasons for these mixed results include differential patterns of resource acquisition and allocation, male ability to monopolize females, or general mating opportunities, all of which can ultimately be influenced by social and ecological variables such as population density and structure, or food availability (Lüpold et al. 2014; Evans and Garcia-Gonzalez 2016; Simmons et al. 2017).
In conclusion, ejaculate investment in D. melanogaster is plastic in response to many different factors, including female reproductive potential and mating status (Lüpold et al. 2011; Sirot et al. 2011), perceived sperm competition risk (Bretman et al. 2009; Hopkins et al. 2019), or the male's own condition (Wigby et al. 2016). As described in this study, male size does not necessarily adequately predict sperm transfer, as large males may transfer more sperm to virgin females (McGraw et al. 2007), but small males transfer more sperm to nonvirgin females (this study). In accordance with theoretical models (Tazzyman et al. 2009), we suggest that small males invest more in certain components of their ejaculate (quality or quantity) to compensate for their low expected future mating potential. Differences in seminal fluid allocation in response to female mating status have been shown in D. melanogaster (Sirot et al. 2011) and might explain the large proportions of sperm displaced by small males, regardless of the amount of sperm they transferred, as well as the higher fertilization success of small males.
Studies such as ours help us understand the dynamics of pre- and postmating sexual selection and its associated consequences for the evolution of primary and secondary sexual traits, and trade-offs between them. Future research could address the condition dependence of sperm motility or viability, or identify candidate seminal-fluid proteins that are transferred at higher concentration by small males, with potential consequences for the sperm displacement or fertilization ability of the male. It might further be interesting to explore the effects of the social context (e.g., operational sex ratio, population density, or rival competitiveness) on how males tailor their ejaculates and females use them.
AUTHOR CONTRIBUTIONS
ANDN and SL developed the experiment. ANDN, JR, SHS, and SL conducted the experiment and collected the data. ANDN analyzed the data. ANDN and SL wrote this article.
ACKNOWLEDGMENTS
We thank V. Zeender and J. Perdigón Ferreira for their help during the experiment, and two anonymous reviewers and M. Dean for their constructive comments. This project was supported by the Swiss National Science Foundation (PP00P3_170669 to SL).
CONFLICT OF INTEREST
The authors declare no conflict of interest.
DATA ARCHIVING
Data are deposited on the Dryad Data Repository: https://doi.org/10.5061/dryad.9w0vt4bfc (De Nardo et al. 2021).
LITERATURE CITED
———.
———.
———.
———.
———.
———.
Associate Editor: M. Dean
Handling Editor: T. Chapman
Author notes
This article corresponds to V. P. Narayan and Y. Wang. 2021. Digest: Does size matter? Condition-dependent sexual selection in Drosophila melanogaster. Evolution. https://doi.org/10.1111/evo.14294