-
PDF
- Split View
-
Views
-
Cite
Cite
Veerle M Darras, Deiodinases: How Nonmammalian Research Helped Shape Our Present View, Endocrinology, Volume 162, Issue 6, June 2021, bqab039, https://doi.org/10.1210/endocr/bqab039
- Share Icon Share
Abstract
Iodothyronine deiodinases are enzymes capable of activating and inactivating thyroid hormones (THs) and have an important role in regulating TH action in tissues throughout the body. Three types of deiodinases (D1, D2, and D3) were originally defined based on their biochemical characteristics. Cloning of the first complementary DNAs in the 1990s (Dio1 in rat and dio2 and dio3 in frog) allowed to confirm the existence of 3 distinct enzymes. Over the years, increasing genomic information revealed that deiodinases are present in all chordates, vertebrates, and nonvertebrates and that they can even be found in some mollusks and annelids, pointing to an ancient origin. Research in nonmammalian models has substantially broadened our understanding of deiodinases. In relation to their structure, we discovered for instance that biochemical properties such as inhibition by 6-propyl-2-thiouracil, stimulation by dithiothreitol, and temperature optimum are subject to variation. Data from fish, amphibians, and birds were key in shifting our view on the relative importance of activating and inactivating deiodination pathways and in showing the impact of D2 and D3 not only in local but also whole body T3 availability. They also led to the discovery of new local functions such as the acute reciprocal changes in D2 and D3 in hypothalamic tanycytes upon photostimulation, involved in seasonal rhythmicity. With the present possibilities for rapid and precise gene silencing in any species of interest, comparative research will certainly further contribute to a better understanding of the importance of deiodinases for adequate TH action, also in humans.
Iodothyronine deiodinases are enzymes that are present throughout the body and are essential in the metabolism of thyroid hormones (THs). They either activate 3,5,3’,5’-tetraiodothyronine (T4), the major hormone secreted by the thyroid gland, to the more active 3,5,3’-triiodothyronine (T3) by outer ring deiodination (ORD) or inactivate T4 and T3 by inner ring deiodination (IRD) to 3,3’,5’-triiodothyronine (rT3) and 3,3’-diiodothyronine (3,3’-T2), respectively. Vertebrates typically possess 3 types of deiodinases: deiodinase type 1 (D1) with both ORD and IRD activity and low substrate affinity (high Km), deiodinase type 2 (D2) with only ORD activity and high substrate affinity (low Km), and deiodinase type 3 (D3) with only IRD activity and high substrate affinity (1,2). Within a given species, these deiodinases show a highly dynamic spatiotemporal expression pattern. In combination with differences in expression of transmembrane TH transporters, this allows different tissues and even specific cell types to regulate intracellular TH levels at least in part independently from the levels present in the general circulation (2,3). Although biochemical proof for the existence of 3 different types of deiodinases was originally obtained by in vitro studies on mammalian (mostly rat) tissues in the late 1970s and early 1980s (see, eg, (4)), much about what we presently know about their structure and function was discovered first in nonmammalian vertebrates. Over the years, studies in fish, amphibians, birds, a few reptiles, and, more recently, a number of invertebrates have provided essential windows for our view on deiodinases, their origin, evolution, and function. The present review aims to highlight a few of these findings.
Evolution of Deiodinases
Structure and function of vertebrate deiodinases
Vertebrate deiodinases are integral membrane proteins, which normally function as homodimers. The hydrophobic transmembrane region of the enzymes is located in the N-terminal domain. Their catalytic center is oriented toward the cytoplasm and contains the rare amino acid selenocysteine (Sec), which is essential for their catalytic activity. Since Sec is encoded by UGA that normally functions as a stop codon, their messenger RNAs (mRNAs) contain a Sec insertion sequence element in the 3’-UTR (2).
Before any deiodinase was cloned, biochemical studies in a variety of species already indicated the presence of TH deiodinating enzymes in both mammals and nonmammalian vertebrates. While these studies showed important similarities, they also suggested some functional variability, especially in fish (eg, (5,6)). The first deiodinase sequence information became available with the cloning of Dio1 cDNA from rat liver in 1991 (7). The first cDNAs for dio2 and dio3 were identified in frogs with the cloning of dio3 cDNA from Xenopus laevis tadpole tails in 1994 (8) and dio2 cDNA from bullfrog Rana catesbeiana skin and hindlimbs in 1995 (9). By now, cDNA sequences are available for 1 or more deiodinases in almost 200 vertebrate species (10), and for some species, the complete gene structure has also been elucidated. Until a few years ago, this sequence information suggested that all vertebrate dio1 and dio2 genes consist of multiple exons while dio3 genes contain no introns, a characteristic that is rather unusual in the eukaryotic kingdom (11). In 2014, however, a multi-exon dio3 gene was identified in zebrafish (12). Teleosts, the “modern” bony fishes (Fig. 1), underwent a whole-genome duplication during evolution, and as a result, they express 2 paralogs of many genes, including dio3. Both paralogs encode functional D3 enzymes but in contrast to the dio3a gene, the dio3b gene contains a large intron separating its open reading frame from its Sec insertion sequence element (12,13). It remains to be studied if this characteristic is specific for zebrafish dio3 or whether it is also found in other, related teleosts.
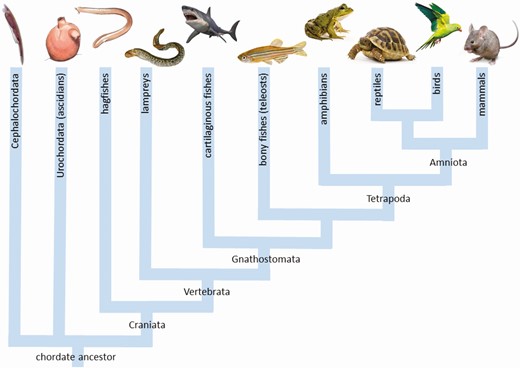
Phylogenetic tree showing the evolutionary relationship between the different groups of chordates mentioned in the text.
The large number of sequences available allowed researchers to construct comprehensive phylogenetic trees, showing that the D1 cluster has the most sequence variation and suggesting that D1 is the oldest vertebrate deiodinase, while the D2 and D3 paralogs appeared more recently (11). A well-known example of functional variability of D1 is its sensitivity to inhibition by the thyrostatic drug 6-propyl-2-thiouracil (PTU). This characteristic has long been considered an important criterion to distinguish PTU-sensitive D1 activity from the PTU-insensitive D2 and D3 activities. This was one of the main reasons why it was originally thought that amphibians and fish do not possess D1, as addition of PTU did not inhibit deiodination in these species. However, a study in bullfrog tadpoles in 1988 already mentioned the presence of a high Km ORD activity in the gut during prometamorphosis (14), and biochemical characterization of the ORD activity in tilapia kidney and liver in 1993 showed the presence of 2 different enzymes: a high Km (µM) enzyme with substrate preference rT3 > T4 and a low Km (nM) enzyme with substrate preference T4 > rT3, resembling mammalian D1 and D2 activity, respectively. The high Km enzyme was, however, insensitive to inhibition by PTU (15). Subsequent cloning of dio1 from tilapia and killifish (16,17) as well as Xenopus (18) confirmed that both fish and amphibians express a PTU-resistant D1. Sequence comparison with PTU-sensitive D1s from mammals and birds showed the presence of a Pro residue instead of a Ser residue 2 positions downstream of the Sec in the catalytic site, similar to the situation in known PTU-insensitive D2 and D3 enzymes. Site-directed mutagenesis in Xenopus D1 (Pro132Ser) restored PTU sensitivity, suggesting that this amino acid is essential for PTU sensitivity (18). However, it also increased Km and maximum velocity for ORD of T4. Further comparative work in killifish and sea bream revealed that rather than a change of 1 specific amino acid, a combination of changes resulting in an increase in substrate turnover rate are probably determinative in the efficiency of PTU to block D1 activity (17,19).
Another surprising finding in teleosts was related to the efficiency of dithiothreitol (DTT) to stimulate deiodinase activity. As the nature of the natural cofactor for deiodinase activity remains elusive, this reducing agent is typically used as cofactor in in vitro kinetic tests for all three types of deiodinases. However, in 2005 a cDNA was cloned for sea bream dio1, coding for a D1 enzyme that proved to be inhibited by DTT (19). This apparently was also the case for rT3 ORD activity in killifish and in the flatfish Solea senegalensis (20,21), but not in several other species including tilapia, African catfish, rainbow trout, and turbot, where all deiodinases were stimulated by DTT (22). A later study in common carp, where DTT potently inhibited ORD in kidney homogenates but stimulated ORD in liver homogenates, continued to investigate this problem. Although the exact reason for the discrepancies in DTT action between and even within species remains unclear, the study at least suggests that the present view of DTT as a strictly reducing agent of a selenyl-iodide intermediate of the enzyme may be too simple and that the endogenous cofactor may have different sites of action on the deiodinase protein (23).
Comparative studies in tissue homogenates of exothermic vertebrates also revealed variability in the temperature optimum of catalytic activities between species. In general, the optimum tends to be lower (20°C-30°C vs 30°C-37°C) in exothermic species living at a low ambient temperature, such as trout, turbot, and red drum, although it may also vary between tissues (22,24). Optima can apparently also shift with acclimation to different rearing temperatures as found in red drum (24), pointing to shifts in the expression of different isozymes, but this was not the case in a study in rainbow trout (25). Interestingly, ORD activity in liver of the turtle Trachemys scripta and the lizard Sceloporus grammicus remained fairly constant over the range of 18°C to 37°C and 15°C to 42°C, respectively (26, 27), suggesting that deiodinases in reptiles are well adapted to a broad range in body temperature.
Deiodinases in invertebrates
Over the years an increasing number of papers have reported on the presence of deiodinases in invertebrate chordates (Fig. 1). One of the first reports, from 1989, showed in vivo deiodination of T4 in the ascidian Phallusia mammillata (28). Twelve years later an in vitro study in different tissues of hagfish showed the presence of T4 ORD, T4 IRD, and T3 IRD activities (29). Interestingly, both ORD and IRD of T4 in hagfish were inhibited by PTU, suggesting this primitive chordate, situated in the evolutionary tree just before the transition to vertebrates (Fig. 1), expresses a D1-like deiodinase that is PTU-sensitive. This adds additional complexity to the question as to why D1 in teleost fish and amphibians is resistant to PTU inhibition.
More recently, the rapid increase in sequence information allowed to identify deiodinase cDNAs in several invertebrate chordates, including both cephalochordates and urochordates (3). Full cloning and characterization of some of these sequences revealed a few puzzling observations. The ascidian Halocynthia roretzi expresses a Sec-containing deiodinase that shows a mix of mammalian D1 and D2 characteristics: a high Km for ORD of rT3 and T4 and ping-pong kinetics, but negligible IRD activity and resistance to PTU (30). Partial sequences available from several other ascidian species suggest that urochordates possess at least 2 deiodinase homologs (30). In the cephalochordate Branchiostoma floridae, at least 3 deiodinase homologs have been identified. Two of them have Sec in their catalytic site, but a third instead has a Cys residue (31). This deiodinase does not deiodinate T4, T3, or rT3 but instead catalyzes IRD of 3,5,3’-triiodothyroacetic acid and 3,5,3’,5’-tetraiodothyroacetic acid. It has a low nM Km and is stimulated by DTT up to 10 mM. Taking into account that the ligand of the TH receptor (TR) in Branchiostoma is neither T3 nor T4 but 3,5,3’-triiodothyroacetic acid, this nonselenodeiodinase may be an important regulator of TH signaling in this species. The deiodinases cloned from Halocynthia and Branchiostoma both have a temperature optimum around 20°C-30°C while activity is strongly decreased at 37°C (30,31). In an evolutionary context, this could mean that the typical optimum around 37°C found in endothermic mammals and birds evolved later as an adaptation to their high body temperature.
Sequences for putative selenoproteins resembling iodothyronine deiodinases have in the meantime also been found in a wide variety of nonchordate invertebrates, including mollusks, annelids, and even sponges and sea anemones (32). So far, no iodothyronine deiodinases have been reported in arthropods and nematodes. This is in line with the fact that in these phyla no TR orthologs have been identified and hence classical TH signaling may be absent. In contrast, annelids and mollusks contain TR orthologs, and TH signaling, although not necessarily TR-mediated, has been demonstrated in some species (33). Three full-length deiodinase-like cDNAs have been cloned in mollusks: 1 from a scallop and 2 from the Pacific oyster. All 3 of them encode a selenoprotein (34,35). In vivo expression of the scallop deiodinase increased following stimulation of the immune system with lipopolysaccharide, while suppression of its expression by double-stranded RNA injection was followed by an increase in the T4/T3 ratio in the hemolymph (34). Exposure of Pacific oysters to T4 increased expression of both deiodinases (35). Changes in hemolymph T4 and T3 levels and in deiodinase mRNA expression were also observed in response to exposure to polybrominated diphenyl ether, a well-known disruptor of mammalian TH homeostasis, in the Manila clam Ruditapes philippinarum (36). This indicates that iodothyronine deiodinases may have a regulatory role under changing physiological conditions in mollusks and possibly also in some other phyla of nonchordate invertebrates where THs have been shown to influence development and life stage transitions.
Local and whole-body impact of D2 and D3
Based on the characteristics and tissue distribution of deiodinases in mammals, mainly studied in rodents, the original view on deiodinase action was that D1 in liver, kidneys, and thyroid was the enzyme responsible for regulating the T3 level in circulation, while D2 and D3 only acted locally to regulate intracellular T3 levels at the site of expression. Emphasis of the research was also mainly on the ORD pathway, producing T3 from T4. Less attention was given to the IRD pathway, responsible for T4 and T3 degradation, as D3 was thought to be almost absent in postnatal life. Research in nonmammalian vertebrates not only helped to discover some specific local actions of D2 and D3, but it also demonstrated their more general impact and was key in gradually shifting our view on the relative importance of the different deiodinases. It is now generally accepted also in mammals that D2 activity in various tissues contributes to circulating T3 levels and that D3 is expressed throughout life, where increased T3 degradation is essential to cope with challenges such as food restriction, infection, and tissue damage (1,37-40).
Deiodinases in development
Chicken embryos and amphibian tadpoles are 2 long-standing models in developmental biology, and their deiodination pathways have been studied already extensively in the 1980s and early 1990s. Data from chicken embryos showed that high Km D1-like activity in liver increased 2- to 3-fold during the last (third) week of embryonic development while low Km D3-like activity was highest around embryonic day 15 and 16 and decreased by more than 90 % during the last 3 days prior to hatching (Fig. 2). It was suggested that this rapid drop in hepatic IRD activity was the main reason for the concurrent sharp increase in plasma T3 (41,42). Following the cloning of chicken DIO1 and DIO3, these changes were confirmed at the level of mRNA expression (43). In the same period, studies in bullfrog tadpoles showed high IRD D3-like activity in liver of pre- and prometamorphic tadpoles that decreased to undetectable levels during metamorphic climax. ORD D2-like activity was absent from liver at all stages but present in gut and skin and increased significantly in skin during metamorphic climax. Rapid degradation of T3 by hepatic D3 was suggested to be the reason for the failure to demonstrate in vivo T4 to T3 conversion in tadpoles prior to metamorphic climax (44,45). Again the changes were confirmed later at the mRNA level when dio2 and dio3 expression could be quantified (46). In turn, investigations in fish showed that in several teleosts D1 activity was low or absent in the liver of euthyroid animals and D2 was the major enzyme regulating plasma T3 (15,22,47). These are just a few examples of how early data from nonmammals helped to challenge and subsequently change the old view on the relative impact of different deiodinases on the T3 level in circulation.
Throughout the years, nonmammalian vertebrate models have also contributed substantially to reveal the importance of divergent spatiotemporal expression of deiodinases to ensure the correct sequence in tissue development. A typical example is the metamorphosing tadpole where development of the limbs has to precede shrinkage of the tail to ensure continuous mobility. D2 and D3 activity and expression were analyzed in these tissues throughout metamorphosis (48). While D2 expression and activity peaked in hindlimbs during late pre- and prometamorphosis, levels only started to increase in the tail during metamorphic climax. D2 activity in forelimbs, which develop later than hindlimbs, was also low during prometamorphosis and only increased during metamorphic climax. Interestingly, D3 activity levels in these tissues varied more or less in parallel with those of D2. This may have been one of the first clear examples showing that although D2 and D3 have opposite activities, their expressions do not necessarily change in an opposite way. Instead, their combined increase in the same tissue indicated a potential for tight control of local T3 levels during important phases of differentiation (48).
Nonmammalian, externally developing model species have also been very helpful in demonstrating the presence of deiodinases and the need for TH signaling at early stages of embryonic development. Dio2 and dio3 mRNA of maternal origin were detected by whole mount in situ hybridization (WISH) in Xenopus embryos at the 2-cell stage and disappeared during gastrulation. Subsequent embryonic expression of all 3 deiodinases was shown by WISH and quantitative polymerase chain reaction around the onset of neurulation (49,50). Exposure to T3 starting at the tailbud stage increased expression of dio2 and dio3, indicating their responsiveness to thyroid status (51). In zebrafish, dio1 and dio2 mRNA of maternal and later embryonic origin was visualized by WISH from the 2-cell stage throughout embryonic development. Both deiodinases were colocalized at first, but their localization started to diverge around 24 h postfertilization. Dio3 mRNA was first detected at the 6-somite stage (12 h postfertilization) (52). Dio1 and dio2 expression was also confirmed by quantitative polymerase chain reaction from the first stage studied (8 h postfertilization) (53). Because of the convenience of rapid and external development, these species are now also increasingly used in high-throughput screening of potentially endocrine disrupting compounds, where expression of deiodinases is 1 of the biomarkers for harmful interaction with thyroid function (54,55). The relatively large chicken embryo, in turn, has been very useful to study the dynamic and cell-specific expression pattern of deiodinases in the early developing brain, highlighting their role in regulating T3 availability in structures such as the brain barriers (Fig. 3), the cerebellum, and the retina (56-60).
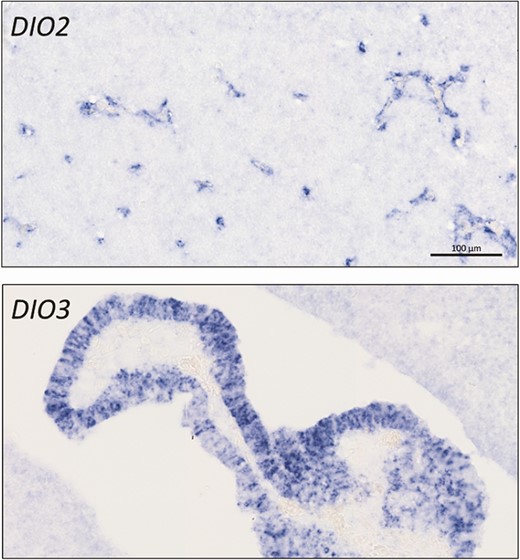
Expression of deiodinases at the brain barriers in 8-day-old chicken embryos. In situ hybridization shows DIO2 expression in the developing capillaries of the blood-brain barrier while DIO3 is highly expressed in the developing choroid plexus at the blood-cerebrospinal fluid barrier. Scale bar 100 µm for both pictures.
One of the obvious ways to investigate deiodinase function is to silence their expression. While transgenic mice deficient in D1, D2, or D3 became available between 2001 and 2006 (61-63), methodological limitations delayed deiodinase knockout in other species for many years. The first alternative deiodinase knockout vertebrate, a D2-deficient zebrafish, was finally reported in 2016 (64). Ten years earlier, however, knockdown by antisense morpholinos already allowed researchers to efficiently block expression of 1 or more deiodinases in zebrafish throughout embryonic and early larval development. This revealed, among other things, that dio2 knockdown disrupted normal development but that dio1 knockdown was only detrimental when T3 availability was already low due to combined dio2 knockdown (65,66). It also proved that both D3 paralogs are active in zebrafish but that dio3b is the predominant one (13). In agreement with the observations in deiodinase knockout mice, dio3 knockdown was more detrimental for zebrafish development than knockdown of the TH-activating deiodinases (Fig. 4) (67,68). Nevertheless, recent results in dio2 knockout zebrafish showed that D2 is not only needed for normal development and that permanent D2 deficiency also has long-lasting effects, including disruption of male and female reproduction and changes in glucose homeostasis (64,69,70).
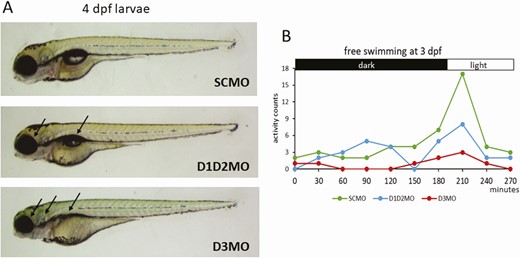
Knockdown of the TH activating (D1+D2) or the inactivating (D3) pathway in developing zebrafish by antisense morpholino (MO). Both knockdown conditions disturb normal development, but the impact of blocking D3 activity is more severe. (A) Larvae at 4 days postfertilization. Arrows point to impact on eye, ear and swim bladder development. (B) Free swimming behavior and reaction to a light pulse at 3 days postfertilization. Abbreviations: D1D2MO: combined injection of dio1- and dio2-blocking MOs, D3MO: injection of dio3b-blocking MO; SCMO: injection of standard control MO.
Deiodinases and seasonal rhythms
A clear and fairly recent example of a local action of deiodinases that was first discovered in nonmammals is the role of D2 and D3 in photoperiodism and seasonal reproduction. This time the model organism was Japanese quail. They are long-day breeders, and their photoperiodic clock is located in the mediobasal hypothalamus. It was found that even a short light pulse in the photo-inducible phase resulted within hours in a sharp increase in DIO2 expression and a sharp decrease in DIO3 expression in this brain area (71,72). It was shown that DIO2 and DIO3 are co-expressed in the tanycytes lining the third ventricle and that the reciprocal switch in D2 and D3 activity results in a local increase in T3 levels. This in turn induces morphological changes in the terminals of the gonadotropin-releasing hormone–producing cells in the median eminence, facilitating gonadotropin-releasing hormone release and hence stimulation of gonadotropin secretion from the pars distalis (73). Interestingly, the increase in DIO2 expression appears to be triggered by thyrotropin (TSH) that is produced locally within the pars tuberalis and binds to TSH receptors within the ependymal cells (74). The same mechanism has also been found in other birds including chickens and buntings (75-77). Although there are some differences in the location of the photosensitive structures and the molecules involved in transmitting light information, TSH signaling and reciprocal changes in hypothalamic D2 and D3 activity have in the meantime also been identified as crucial in seasonal rhythmicity in several mammals as well as some fish (for reviews, see (78-81)).
Conclusion
Research in nonmammalian species has contributed greatly to our present knowledge of the origin and evolution of iodothyronine deiodinases. Moreover, it has been essential in studying their function. Several findings in nonmammalian vertebrates have pointed to new roles that were later found to exist also in mammals, including humans. It is clear that comparative research has broadened our view on the impact of these important enzymes on TH action throughout life. With the present possibilities for rapid and precise gene silencing in any species of interest, it will also continue to do so in the future.
Acknowledgments
The author would like to thank Dr. P. Vancamp for his useful comments on the first draft of the manuscript.
Additional Information
Disclosures: The author has no conflicts of interest to disclose.
Data Availability
Data sharing is not applicable to this article as no data sets were generated or analyzed during the current study.