-
PDF
- Split View
-
Views
-
Cite
Cite
Ilaria Cellai, Vincenza Di Stasi, Paolo Comeglio, Elisa Maseroli, Tommaso Todisco, Chiara Corno, Sandra Filippi, Sarah Cipriani, Flavia Sorbi, Massimiliano Fambrini, Felice Petraglia, Irene Scavello, Giulia Rastrelli, Gabriele Acciai, Fabio Villanelli, Giovanna Danza, Erica Sarchielli, Giulia Guarnieri, Annamaria Morelli, Mario Maggi, Linda Vignozzi, Insight on the Intracrinology of Menopause: Androgen Production within the Human Vagina, Endocrinology, Volume 162, Issue 2, February 2021, bqaa219, https://doi.org/10.1210/endocr/bqaa219
- Share Icon Share
Abstract
In this study, we investigated steroidogenic gene mRNA expression in human vaginas and verified the ability of human vagina smooth muscle cells (hvSMCs) to synthesize androgens from upstream precursor dehydroepiandrosterone (DHEA). As a readout for androgen receptor (AR) activation, we evaluated the mRNA expression of various androgen-dependent markers. hvSMCs were isolated from vagina tissues of women undergoing surgery for benign gynecological diseases. In these cells, we evaluated mRNA expression of several steroidogenic enzymes and sex steroid receptors using real time reverse transcription-polymerase chain reaction. Androgen production was quantified with liquid chromatography tandem-mass spectrometry (LC-MS/MS).
In vaginal tissues, AR mRNA was significantly less expressed than estrogen receptor α, whereas in hvSMCs, its mRNA expression was higher than progestin and both estrogen receptors. In hvSMCs and in vaginal tissue, when compared to ovaries, the mRNA expression of proandrogenic steroidogenic enzymes (HSD3β1/β2, HSD17β3/β5), along with 5α-reductase isoforms and sulfotransferase, resulted as being more abundant. In addition, enzymes involved in androgen inactivation were less expressed than in the ovaries. The LC-MS/MS analysis revealed that, in hvSMCs, short-term DHEA supplementation increased Δ4-androstenedione levels in spent medium, while increasing testosterone and DHT secretion after longer incubation. Finally, androgenic signaling activation was evaluated through AR-dependent marker mRNA expression, after DHEA and T stimulation.
This study confirmed that the human vagina is an androgen-target organ with the ability to synthesize androgens, thus providing support for the use of androgens for local symptoms of genitourinary syndrome in menopause.
Sex steroids are hormones derived from cholesterol with fundamental roles in the development and functioning of female sexual organs, such as the vagina (1-5). Two main classes of sex steroids, namely androgens and estrogens, are secreted (the latter not in a significant amount) by the outer cortex of adrenal glands and, in a sexually dimorphic manner, also by the gonads, showing dramatic fluctuations throughout the different stages of life (6).
The human vagina is extremely sensitive to changes in the hormonal milieu throughout a person’s lifespan. Among sex steroids, estradiol (E2) has been historically considered to be the most important hormone for vaginal health. Therefore, a wide spectrum of genital and sexual symptoms (including dryness, burning, irritation, lack of lubrication, discomfort or pain, urgency, dysuria, and recurrent urinary tract infections), encompassed by the term “genitourinary syndrome of menopause” (GSM) (7), has been closely tied to the drop of estrogen level after menopause. More recently, the role of androgens in the development and treatment of the GSM has received increased attention (8). In fact, the fall in ovarian estrogen production at menopause is accompanied by a progressive, and ageing-dependent, decline in androgens (9). Nevertheless, to protect peripheral tissues from circulating hormonal imbalances, small amounts of estrogens and androgens are also produced in situ from inactive precursors by cell-specific sets of enzymes. This specific local production and activity of hormones, which differs from the classical processes, is defined as “intracrinology” (10). A peculiar intracellular enzymatic equipment—by which dehydroepiandrosterone (DHEA), an almost biologically inactive androgen, is locally converted into testosterone (T) and 17β-E2—has been evoked to protect several peripheral tissues, including the vagina (11), from hormonal deficiency. Indeed, adrenal DHEA, which is considered as the only source of sex steroids in menopausal women, starts to decline at about the age of 30 years, resulting, on average, in a 60% decrease after menopause. In peripheral tissues, a parallel 60% decrease in androgen biosynthesis from DHEA has therefore been hypothesized (11, 12). Consistent with this view, a novel approach aimed at treating GSM symptoms using intravaginal DHEA has been postulated.
Bertin and colleagues first demonstrated that the intracrinological enzymatic machinery able to produce androgens was present in the vagina of rodents and even in the cynomolgus monkey (Macaca fascicularis), the closest animal model to the human one (13). However, data on intracrinology in the human vagina are still lacking. Hence, addressing this hypothesis appears to be of particular relevance, not only to better understand the physiology of the female genitourinary tract, but also from a clinical perspective, to clarify the mechanisms of action of intravaginal DHEA.
The aim of the study was to investigate whether the human vagina possesses the proper enzymatic machinery to convert weak androgen precursors, such as DHEA, into active androgens, as already described in other species. We recently demonstrated that androgens are potent anti-inflammatory hormones in human vaginal smooth muscle cells (hvSMCs) (14), thus raising the urgent need to investigate the ability of these cells to produce their own androgens. We therefore aimed to examine if isolated hvSMCs maintained the ability to convert DHEA into Δ4-androstenedione and T. Moreover, we wanted to investigate the capability of DHEA, through its conversion into active androgens, to induce androgen-dependent signaling by androgen receptor (AR) stimulation on these cells. All steroid measurements were performed using an isotopic dilution liquid chromatography tandem mass spectrometry method (LC-MS/MS), the gold standard method for steroid quantification.
Material and Methods
Collection of samples during surgical procedure
Human vagina (n = 28 samples) and ovary (n = 18 samples) tissues were obtained, after informed consent, from postmenopausal women (age range 60-82 years) undergoing surgery for benign gynecological conditions. The study received approval from the Ethics Committee “Area Vasta Centro” (Azienda Ospedaliero Universitaria Careggi, Florence, Italy: HUMVAGDHT, Prot. N. 12903). Specifically, specimens from the upper third of the distal vagina, measuring approximately 3 × 1 cm, were collected.
Human vaginal smooth muscle cells culture
hvSMCs were isolated from vaginal tissue biopsies obtained from postmenopausal women. The collected tissue samples were first minced with a surgical knife and subsequently incubated with type IV bacterial collagenase (2 mg/mL; Worthington Biochemical Corporation, Lakewood, NJ) for 12 hours at 37°C. The samples were washed with phosphate-buffered saline and centrifuged at 500g for 10 minutes. Pellets obtained from centrifugation were collected and located in Dulbecco’s Modified Eagle’s Medium Ham F-12 culture medium (1:1; Sigma-Aldrich, St Louis, MO), deprived of phenol red, supplemented with fetal bovine serum 10% (Sigma-Aldrich), antibiotics (penicillin 100 IU/mL, streptomycin 100 mg/mL; Sigma-Aldrich), and amphotericin B (1 µg/mL; Sigma-Aldrich), and then kept at a steady temperature of 37°C with an H2O and CO2 percentage of 95% and 5%, respectively. Cells (hvSMCs) began to emerge after 48 hours and they had been used by the seventh or eighth passage.
Cells were seeded in 6-well plates (1.5 × 105 cells per well) with culture medium in them. After 24 to 48 hours, the cells were washed with phosphate-buffered saline, transferred to culture media in Dulbecco’s Modified Eagle’s Medium Ham F-12 culture medium and then serum deprived for 24 hours. Cells were thus stimulated for 24 and 48 hours, either with increasing doses of DHEA (from 1 nM to 30 nM) or with a single dose of T (0.6 nM), which correspond to the physiological concentrations reported in the blood of healthy women in early adulthood or in the postmenopausal phase (9). The experiments were also replicated with or without the nonsteroidal antiandrogen bicalutamide (1 µM). For every procedure, suspended cells were centrifuged, and culture media collected while cell pellets were stored at –80°C for further tests, including mRNA expression analysis.
Immunohistochemistry characterization
Cells (hvSMCs) were analyzed with immunohistochemistry using monoclonal antibodies for smooth muscle cell (SMC)-specific markers, namely the myosin heavy chain 11 (MYH11; mouse monoclonal antibody, Cat# ab683, RRID:AB_2235569; Abcam, Cambridge, UK) (15), vimentin (mouse monoclonal antibody, Cat# sc-6260, RRID:AB_628437; Santa Cruz Biotechnology, Dallas, TX) (16) and actin (αSMA; mouse monoclonal antibody, Cat# A2547, RRID:AB_476701; Sigma-Aldrich) (17), and the epithelial marker antipan keratin (prediluted murine monoclonal antibody, Cat# 760-2135, RRID:AB_2810237; Roche Diagnostics, Indianapolis, IN) (18) was used as a negative control. An immunohistochemical study to assess the expression of the AR (rabbit polyclonal antibody, Cat# sc-816, RRID:AB_1563391; Santa Cruz Biotechnology) (19) was also performed.
Briefly, the cells were fixed in 2% paraformaldehyde; 0.1% Triton X-100 was used for permeabilization, and a nonspecific site blockade was attained with 1% bovine serum albumin. Cells were subsequently incubated with MYH11 (1:200 vol/vol), αSMA (1:100 vol/vol), vimentin (1:100 vol/vol), pankeratin (prediluted), and AR (1:50 vol/vol) specific antibodies, and later with secondary specific antimouse (goat monoclonal secondary antibody, Alexa fluor 488, Cat# A-11029, RRID:AB_2534088; Thermo Fisher Scientific, Waltham, MA) (20) or antirabbit (goat polyclonal secondary antibody, Alexa fluor 568, Cat# A-11011, RRID:AB_143157; Thermo Fisher Scientific) (21) antibodies, according to the manufacturer’s instructions. Nuclear localization of AR was highlighted by DAPI (4′,6-diamidino-2-phenylindol) dye. Finally, the specificity of antibodies was evaluated by immunostaining verification without primary antibody.
Real-time polymerase chain reaction
Total RNA from tissues and cells was collected using TRIzol (Life Technologies, Paisley, UK) and/or RNeasy Mini Kit (Qiagen, Hilden, Germany), as indicated by the manufacturer. cDNA synthesis was attained using the iScript cDNA Synthesis Kit (Bio-Rad Laboratories, Hercules, CA). Amplification and detection through real time PCR were carried out using either SsoAdvanced Universal Probes Supermix or SsoAdvanced Universal SYBR Green Supermix, and using the CFX96 Detection System (all Bio-Rad Laboratories). Probes and primers were purchased from Life Technologies. Primers for target genes were designed using available sequences obtained from the “National Center for Biotechnology Information GenBank” (http://www.ncbi.nlm.nih.gov) and the “Ensembl Genome” (http://www.ensembl.org) registers. Primer sequences and amplified fragment sizes are reported in Table 1. Expression of the RNA 18S ribosomal was used as housekeeping for semiquantitation of target genes, based on the comparative threshold cycle (Ct) 2-ΔΔCt method (22).
Human primers . | . | . | Product length . |
---|---|---|---|
AR | F | TACCAGCTCACCAAGCTCCT | 195 bp |
R | GCTTCACTGGGTGTGGAAAT | ||
CYP11A1 | F | ACGCTCAGTCCTGGTCAAAG | 133 bp |
R | GGGGATCTCATTGAAGGGGC | ||
CYP17A1 | F | TCCTGCTGCACAATCCTCAG | 82 bp |
R | ATAGTTGGTGTGCGGCTGAA | ||
CYP19A1 | F | TGCGAGTCTGGATCTCTGGA | 106 bp |
R | AGTTTGCTGCCGAATCGAGA | ||
ERα | F | AGCACCCTGAAGTCTCTGGA | 153 bp |
R | GATGTGGGAGAGGATGAGGA | ||
ERβ | F | AAGAAGATTCCCGGCTTTGT | 173 bp |
R | TCTACGCATTTCCCCTCATC | ||
GPER1 | F | AGGGACAAGCTGAGGCTGTA | 137 bp |
R | GTCTACACGGCACTGCTGAA | ||
GUCY1A2 | F | CCCTATCCATGATGCCACCC | 121 bp |
R | CAGGGCCTGGTGAGTTCTTT | ||
HSD3β1 | F | CCCTTCAACCGCCACATAGT | 121 bp |
R | CCGTTTTCTGCTTGGCTTCC | ||
HSD3β2 | F | GGAAGCCAAGCAGAAAACCG | 172 bp |
R | GCCCCTGTTGCCTTCTGTAT | ||
HSD17β2 | F | AGTCTGCCTGCTCATCCTGT | 138 bp |
R | CAATCACCACCTGTCACCAG | ||
HSD17β3 | F | ATTTCCTGAACGCACCGGAT | 186 bp |
R | ACGCCTTGGAAGCTGAGTAC | ||
HSD17β5 | F | CGAGACAAACGATGGGTGGA | 169 bp |
R | GTCTGATGCGCTGCTCATTG | ||
PKG1 | F | ATTGGCATGACAGAGTGGGG | 183 bp |
R | CCACCCTACCCCAAGCATTT | ||
PR | F | GTACGGAGCCAGCAGAAGTC | 143 bp |
R | TCTCTGGCATCAAACTCGTG | ||
SRD5A1 | F | ACTTGCCAACCTTCGTGTCA | 167 bp |
R | TATGAACCACCACCAGCACC | ||
SRD5A2 | F | GGCCGGTCCTTGAGATGAAA | 107 bp |
R | TAGAAAGCGCCCTCTTGTGG | ||
SRD5A3 | F | CAACGTAGCATGGCCTTTGG | 140 bp |
R | AGTCCCTGATGCTCTCCCTT | ||
STAMP2 | F | CAAGTCGGCAGGTGTTTGTG | 113 bp |
R | GGCTGCCATGAGTGATCCTT | ||
STAR | F | TCCTTGACCCCTTCCTTTGC | 186 bp |
R | GCTGCTGCCTCTTCTCTCAA | ||
STS | F | ACACGTGTGCTTCTGTTTCG | 171 bp |
R | AGGGTCTGGGTGTGTCTGTC | ||
SULT2A1 | F | GTGGATCCAATCTGTGCCCA | 112 bp |
R | GGGGAGGTGGGAGGAGAATA |
Human primers . | . | . | Product length . |
---|---|---|---|
AR | F | TACCAGCTCACCAAGCTCCT | 195 bp |
R | GCTTCACTGGGTGTGGAAAT | ||
CYP11A1 | F | ACGCTCAGTCCTGGTCAAAG | 133 bp |
R | GGGGATCTCATTGAAGGGGC | ||
CYP17A1 | F | TCCTGCTGCACAATCCTCAG | 82 bp |
R | ATAGTTGGTGTGCGGCTGAA | ||
CYP19A1 | F | TGCGAGTCTGGATCTCTGGA | 106 bp |
R | AGTTTGCTGCCGAATCGAGA | ||
ERα | F | AGCACCCTGAAGTCTCTGGA | 153 bp |
R | GATGTGGGAGAGGATGAGGA | ||
ERβ | F | AAGAAGATTCCCGGCTTTGT | 173 bp |
R | TCTACGCATTTCCCCTCATC | ||
GPER1 | F | AGGGACAAGCTGAGGCTGTA | 137 bp |
R | GTCTACACGGCACTGCTGAA | ||
GUCY1A2 | F | CCCTATCCATGATGCCACCC | 121 bp |
R | CAGGGCCTGGTGAGTTCTTT | ||
HSD3β1 | F | CCCTTCAACCGCCACATAGT | 121 bp |
R | CCGTTTTCTGCTTGGCTTCC | ||
HSD3β2 | F | GGAAGCCAAGCAGAAAACCG | 172 bp |
R | GCCCCTGTTGCCTTCTGTAT | ||
HSD17β2 | F | AGTCTGCCTGCTCATCCTGT | 138 bp |
R | CAATCACCACCTGTCACCAG | ||
HSD17β3 | F | ATTTCCTGAACGCACCGGAT | 186 bp |
R | ACGCCTTGGAAGCTGAGTAC | ||
HSD17β5 | F | CGAGACAAACGATGGGTGGA | 169 bp |
R | GTCTGATGCGCTGCTCATTG | ||
PKG1 | F | ATTGGCATGACAGAGTGGGG | 183 bp |
R | CCACCCTACCCCAAGCATTT | ||
PR | F | GTACGGAGCCAGCAGAAGTC | 143 bp |
R | TCTCTGGCATCAAACTCGTG | ||
SRD5A1 | F | ACTTGCCAACCTTCGTGTCA | 167 bp |
R | TATGAACCACCACCAGCACC | ||
SRD5A2 | F | GGCCGGTCCTTGAGATGAAA | 107 bp |
R | TAGAAAGCGCCCTCTTGTGG | ||
SRD5A3 | F | CAACGTAGCATGGCCTTTGG | 140 bp |
R | AGTCCCTGATGCTCTCCCTT | ||
STAMP2 | F | CAAGTCGGCAGGTGTTTGTG | 113 bp |
R | GGCTGCCATGAGTGATCCTT | ||
STAR | F | TCCTTGACCCCTTCCTTTGC | 186 bp |
R | GCTGCTGCCTCTTCTCTCAA | ||
STS | F | ACACGTGTGCTTCTGTTTCG | 171 bp |
R | AGGGTCTGGGTGTGTCTGTC | ||
SULT2A1 | F | GTGGATCCAATCTGTGCCCA | 112 bp |
R | GGGGAGGTGGGAGGAGAATA |
Abbreviations: AR, androgen receptor; CYP17A1, cytochrome P450 17A1; CYP11A1, cytochrome P450 11A1; CYP19A1, cytochrome P450 19A1; ERα, estrogen receptor alpha; ERβ, estrogen receptor beta; GPER1, G protein-coupled receptor 1; GUCY1A2, guanylate cyclase soluble subunit alpha-2; HSD3β1, 3-beta-hydroxysteroid dehydroxynase/delta 5-4 isomerase type 1; HSD3β2, 3-beta-hydroxysteroid dehydroxynase/delta 5-4 isomerase type 2; HSD17β2, 17β-hydroxysteroid dehydrogenase 1; HSD17β3, 17β-hydroxysteroid dehydrogenase 3; HSD17β5, 17β-hydroxysteroid dehydrogenase 5; PKG1, cGMP-dependent protein kinase 1; PR, progesterone receptor; SRD5A1, 3-oxo-5α-steroid 4-dehydrogenase 1; SRD5A2, 3-oxo-5α-steroid 4-dehydrogenase 2; SRD5A3, 3-oxo-5α-steroid 4-dehydrogenase 3; STAMP2, six transmembrane protein of prostate 2; STAR, steroidogenic acute regulatory protein; STS, steroid sulfatase; SULT2A1, sulfotransferase 2A1.
Human primers . | . | . | Product length . |
---|---|---|---|
AR | F | TACCAGCTCACCAAGCTCCT | 195 bp |
R | GCTTCACTGGGTGTGGAAAT | ||
CYP11A1 | F | ACGCTCAGTCCTGGTCAAAG | 133 bp |
R | GGGGATCTCATTGAAGGGGC | ||
CYP17A1 | F | TCCTGCTGCACAATCCTCAG | 82 bp |
R | ATAGTTGGTGTGCGGCTGAA | ||
CYP19A1 | F | TGCGAGTCTGGATCTCTGGA | 106 bp |
R | AGTTTGCTGCCGAATCGAGA | ||
ERα | F | AGCACCCTGAAGTCTCTGGA | 153 bp |
R | GATGTGGGAGAGGATGAGGA | ||
ERβ | F | AAGAAGATTCCCGGCTTTGT | 173 bp |
R | TCTACGCATTTCCCCTCATC | ||
GPER1 | F | AGGGACAAGCTGAGGCTGTA | 137 bp |
R | GTCTACACGGCACTGCTGAA | ||
GUCY1A2 | F | CCCTATCCATGATGCCACCC | 121 bp |
R | CAGGGCCTGGTGAGTTCTTT | ||
HSD3β1 | F | CCCTTCAACCGCCACATAGT | 121 bp |
R | CCGTTTTCTGCTTGGCTTCC | ||
HSD3β2 | F | GGAAGCCAAGCAGAAAACCG | 172 bp |
R | GCCCCTGTTGCCTTCTGTAT | ||
HSD17β2 | F | AGTCTGCCTGCTCATCCTGT | 138 bp |
R | CAATCACCACCTGTCACCAG | ||
HSD17β3 | F | ATTTCCTGAACGCACCGGAT | 186 bp |
R | ACGCCTTGGAAGCTGAGTAC | ||
HSD17β5 | F | CGAGACAAACGATGGGTGGA | 169 bp |
R | GTCTGATGCGCTGCTCATTG | ||
PKG1 | F | ATTGGCATGACAGAGTGGGG | 183 bp |
R | CCACCCTACCCCAAGCATTT | ||
PR | F | GTACGGAGCCAGCAGAAGTC | 143 bp |
R | TCTCTGGCATCAAACTCGTG | ||
SRD5A1 | F | ACTTGCCAACCTTCGTGTCA | 167 bp |
R | TATGAACCACCACCAGCACC | ||
SRD5A2 | F | GGCCGGTCCTTGAGATGAAA | 107 bp |
R | TAGAAAGCGCCCTCTTGTGG | ||
SRD5A3 | F | CAACGTAGCATGGCCTTTGG | 140 bp |
R | AGTCCCTGATGCTCTCCCTT | ||
STAMP2 | F | CAAGTCGGCAGGTGTTTGTG | 113 bp |
R | GGCTGCCATGAGTGATCCTT | ||
STAR | F | TCCTTGACCCCTTCCTTTGC | 186 bp |
R | GCTGCTGCCTCTTCTCTCAA | ||
STS | F | ACACGTGTGCTTCTGTTTCG | 171 bp |
R | AGGGTCTGGGTGTGTCTGTC | ||
SULT2A1 | F | GTGGATCCAATCTGTGCCCA | 112 bp |
R | GGGGAGGTGGGAGGAGAATA |
Human primers . | . | . | Product length . |
---|---|---|---|
AR | F | TACCAGCTCACCAAGCTCCT | 195 bp |
R | GCTTCACTGGGTGTGGAAAT | ||
CYP11A1 | F | ACGCTCAGTCCTGGTCAAAG | 133 bp |
R | GGGGATCTCATTGAAGGGGC | ||
CYP17A1 | F | TCCTGCTGCACAATCCTCAG | 82 bp |
R | ATAGTTGGTGTGCGGCTGAA | ||
CYP19A1 | F | TGCGAGTCTGGATCTCTGGA | 106 bp |
R | AGTTTGCTGCCGAATCGAGA | ||
ERα | F | AGCACCCTGAAGTCTCTGGA | 153 bp |
R | GATGTGGGAGAGGATGAGGA | ||
ERβ | F | AAGAAGATTCCCGGCTTTGT | 173 bp |
R | TCTACGCATTTCCCCTCATC | ||
GPER1 | F | AGGGACAAGCTGAGGCTGTA | 137 bp |
R | GTCTACACGGCACTGCTGAA | ||
GUCY1A2 | F | CCCTATCCATGATGCCACCC | 121 bp |
R | CAGGGCCTGGTGAGTTCTTT | ||
HSD3β1 | F | CCCTTCAACCGCCACATAGT | 121 bp |
R | CCGTTTTCTGCTTGGCTTCC | ||
HSD3β2 | F | GGAAGCCAAGCAGAAAACCG | 172 bp |
R | GCCCCTGTTGCCTTCTGTAT | ||
HSD17β2 | F | AGTCTGCCTGCTCATCCTGT | 138 bp |
R | CAATCACCACCTGTCACCAG | ||
HSD17β3 | F | ATTTCCTGAACGCACCGGAT | 186 bp |
R | ACGCCTTGGAAGCTGAGTAC | ||
HSD17β5 | F | CGAGACAAACGATGGGTGGA | 169 bp |
R | GTCTGATGCGCTGCTCATTG | ||
PKG1 | F | ATTGGCATGACAGAGTGGGG | 183 bp |
R | CCACCCTACCCCAAGCATTT | ||
PR | F | GTACGGAGCCAGCAGAAGTC | 143 bp |
R | TCTCTGGCATCAAACTCGTG | ||
SRD5A1 | F | ACTTGCCAACCTTCGTGTCA | 167 bp |
R | TATGAACCACCACCAGCACC | ||
SRD5A2 | F | GGCCGGTCCTTGAGATGAAA | 107 bp |
R | TAGAAAGCGCCCTCTTGTGG | ||
SRD5A3 | F | CAACGTAGCATGGCCTTTGG | 140 bp |
R | AGTCCCTGATGCTCTCCCTT | ||
STAMP2 | F | CAAGTCGGCAGGTGTTTGTG | 113 bp |
R | GGCTGCCATGAGTGATCCTT | ||
STAR | F | TCCTTGACCCCTTCCTTTGC | 186 bp |
R | GCTGCTGCCTCTTCTCTCAA | ||
STS | F | ACACGTGTGCTTCTGTTTCG | 171 bp |
R | AGGGTCTGGGTGTGTCTGTC | ||
SULT2A1 | F | GTGGATCCAATCTGTGCCCA | 112 bp |
R | GGGGAGGTGGGAGGAGAATA |
Abbreviations: AR, androgen receptor; CYP17A1, cytochrome P450 17A1; CYP11A1, cytochrome P450 11A1; CYP19A1, cytochrome P450 19A1; ERα, estrogen receptor alpha; ERβ, estrogen receptor beta; GPER1, G protein-coupled receptor 1; GUCY1A2, guanylate cyclase soluble subunit alpha-2; HSD3β1, 3-beta-hydroxysteroid dehydroxynase/delta 5-4 isomerase type 1; HSD3β2, 3-beta-hydroxysteroid dehydroxynase/delta 5-4 isomerase type 2; HSD17β2, 17β-hydroxysteroid dehydrogenase 1; HSD17β3, 17β-hydroxysteroid dehydrogenase 3; HSD17β5, 17β-hydroxysteroid dehydrogenase 5; PKG1, cGMP-dependent protein kinase 1; PR, progesterone receptor; SRD5A1, 3-oxo-5α-steroid 4-dehydrogenase 1; SRD5A2, 3-oxo-5α-steroid 4-dehydrogenase 2; SRD5A3, 3-oxo-5α-steroid 4-dehydrogenase 3; STAMP2, six transmembrane protein of prostate 2; STAR, steroidogenic acute regulatory protein; STS, steroid sulfatase; SULT2A1, sulfotransferase 2A1.
Steroids quantification
Steroids were quantified in cellular media using LC-MS/MS using a Sciex 6500QTRAP machine (Sciex, Framingham, MA) equipped with a 1260 high-performance liquid chromatography (Agilent Technologies, Santa Clara, CA). Briefly, 300 µL of cellular medium were added to 300 µL of methanol containing the internal standards of T 2,3,4-13C3 (Sigma-Aldrich), androstane 3–17 dione 2,3,4-13C3 (Sigma-Aldrich), 5α-dihydrotestosterone (DHT) 16,16,17 D3 (Sigma-Aldrich), estrone (E1) 2,4,16,16 D4 (Sigma-Aldrich), and E2 16,16,17 D3 (Sigma-Aldrich), and successively extracted with a mixture of hexane/ethyl acetate (9:1 vol/vol). The extract was dried under nitrogen and reconstituted with 150 µL of water/methanol (70/30 vol/vol); then 50 µL was injected. The analytic system consisted of a washing/preconcentrating column of SPE C18 5 µm 20 × 2 mm and an analytic column of Luna C18 3 µm 50 × 2 mm for androgens, or an analytic column of Luna PFP 3 µm 50 × 2 mm for estrogens. All high-performance liquid chromatography columns were purchased from Phenomenex (Torrance, CA). The standard curve range was 2 to 100 pg/mL for each steroid and internal standards were 200 pg/mL. The limits of quantitation were 4.6 pg/mL for T, 3.8 pg/mL for Δ4-androstenedione (A), 4.5 pg/mL for DHT, 4.1 pg/mL for E1, and 2.8 pg/mL for E2, respectively. The analysis was performed in multiple reaction monitoring mode in positive (A, T, and DHT) or negative (E1 and E2) mode. Two transitions were followed for each analyte: T (289-109 and 289-97); T 13C3 (292-97); A (287-109 and 287-97); A 13C3 (290-100); DHT (291-255 and 291-159); DHT D3 (294-258); E1 (269-183 and 269-145); E1 D4 (273-147); E2 (271-183 and 271-145); E2 D3 (274-145). Quantitation was done using MULTIQUANT software (Sciex).
Statistical analysis
Results are expressed as mean ± SEM (standard error of mean), when normally distributed, and median ± SEM when non-normally distributed. Comparisons of means were performed with 1-way analysis of variance followed by unpaired 2-sided Student’s t-tests, with P < .05 taken to be statistically significant.
When data were non-normally distributed, statistical differences were assessed by the Kruskal–Wallis test followed by the Mann–Whitney test where appropriate to evaluate differences between the groups, with P < .05 considered to be statistically significant. The statistical analyses were performed with the Statistical Package for the Social Sciences for Windows (SPSS v.26.0; SPSS Inc., Chicago, IL).
Results
Human vaginal smooth muscle cell characterization
Figure 1 shows the results of immunofluorescence studies. hvSMCs, isolated from human vaginal biopsies, showed high positivity for the following SMC markers: MYH11 (91.5 ± 6.2%; Fig. 1A), αSMA (100%; Fig. 1B), and vimentin (100%; Fig. 1C); however, they were negative for the specific epithelial marker cytokeratin C (0.9 ± 0.4%; Fig. 1D).
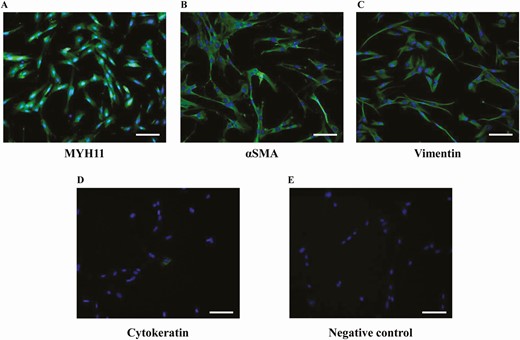
Characterization of hSMCs isolated from human vagina. (A, B, C, D) Representative images of hSMCs immunostaining for specific smooth muscle markers MYH11, αSMA, and vimentin, and for the epithelial marker cytokeratin (taken as a negative control), respectively. (E) A representative image of the negative control obtained omitting the primary antibodies. The quantitative analysis was performed by counting positive cells in at least 10 fields per slide of 3 different cell preparations obtained from three patients (scale bar = 100 µm).
mRNA expression of sex steroid receptors in human distal vaginal tissues and cells
The relative gene expression of sex steroid hormone receptors in the human vagina and in primary SMC lines (hvSMCs) is reported in Fig. 2A and 2B.
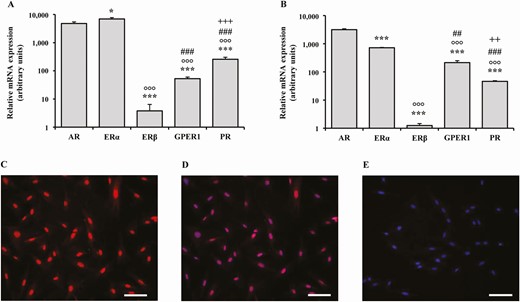
Relative mRNA expression of sex steroid receptors in human distal vaginal tissues and cells. (A, B) The relative mRNA expression of sex steroid receptors AR, ERα, ERβ, GPER1, and PR in human vaginal tissues derived from laparoscopic biopsies (n = 10) and in smooth muscle cells (hSMCs) isolated from the corresponding vaginal samples (n = 4, performed in triplicate), respectively. Data were calculated according to the 2–ΔΔCt comparative method, using ribosomal subunit 18S as the housekeeping gene for normalization. Results are expressed as arbitrary units, calculated as fold increase vs the lower mRNA expression value in the experiment, and are reported as mean ± SEM. *P < .05, ***P < .001 vs AR; °°°P < .001 vs ERα; ##P < .01, ###P < .001 vs ERβ; ++P < .01, +++ P < .001 vs GPER1. (C, D) Two representative images of nuclear immunostaining for AR receptor and the corresponding nuclear DAPI double labeling, respectively. (E) A representative image of the negative control obtained omitting the primary antibodies. At least 3 preparations obtained from 3 different patients were evaluated (scale bar = 100 µm).
In human vaginal tissue, AR mRNA expression was higher than estrogen receptor (ER)β, G protein-coupled estrogen receptor 1 (GPER1), and progesterone receptor (PR) (all P < .001), but lower than ERα (P < .05) (Fig. 2A). In hvSMCs, the AR was the most expressed receptor (all P < .001). In addition, all the investigated hvSMCs show AR immunostaining (Fig. 2C). Figure 2D shows nuclear DAPI counterstaining. Among ERs, in both vaginal tissue and hvSMCs, ERα was the most expressed, followed by GPER1 and ERβ (Fig. 2A and 2B).
mRNA expression of steroidogenesis-related genes in human distal vaginal tissues
In order to evaluate the ability of hvSMCs to synthetize de novo androgens, we analyzed the expression of the main genes involved in steroidogenic pathways in an extract of the human vagina by using real-time RT-PCR (Fig. 3). Results are expressed as percentage of the relative target gene mRNA abundance in human ovary tissue, indicated by a dotted line in Fig. 3.
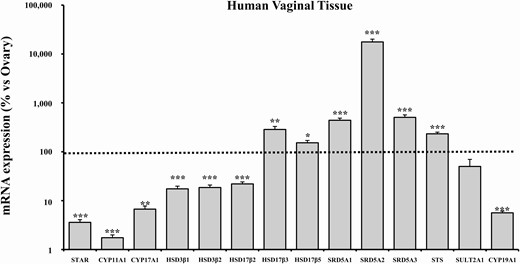
mRNA expression of steroidogenesis pathway-related genes in human vaginal tissue. This figure shows the mRNA expression of genes involved in steroidogenesis in human distal vaginal tissues derived from laparoscopic biopsies (n = 29). Data were calculated according to the 2–ΔΔCt comparative method, using ribosomal subunit 18S as the housekeeping gene for normalization. Results are expressed as percentage of ovary mRNA expression and are reported as mean ± SEM. *P < .05, **P < .01, ***P < .001 vs ovary.
In the human vagina, the enzymes linked to cholesterol transport (steroidogenic acute regulatory protein, STAR) and those involved in the first steps of steroidogenesis (cytochrome P450 11A1 [CYP11A1] and cytochrome P450 17A1 [CYP17A1]) were expressed at much lower levels than in the ovary tissue (Fig. 3; STAR: 3.59 ± 0.48%, P < .001 vs ovary; CYP11A1: 1.74 ± 0.24%, P < .001 vs. ovary; CYP17A1: 6.71 ± 1.05%, P < .01 vs. ovary).
The 3-beta-hydroxysteroid dehydroxynase/delta 5-4 isomerase type 1 (HSD3β1) and type 2 (HSD3β2), catalyzing the oxidative conversion of Δ5-3β-hydroxysteroids to the Δ4-3-keto configuration and converting DHEA to Δ4-androstenedione, showed detectable mRNA expression levels (17.43 ± 2.36% and 18.55 ± 2.32%, respectively), although statistically lower than the ovary (Fig. 3; both P < .001).
Interestingly, compared with the ovary, we observed a significantly higher mRNA expression of enzymes specifically involved in androgen synthesis, namely 17β-hydroxysteroid dehydrogenase type 3 (HSD17β3; 285.61 ± 43.98%, P < .01 vs ovary) and 17β-hydroxysteroid dehydrogenase type 5 (HSD17β5; 152.59 ± 17.71%, P < .05 vs ovary). In contrast, the mRNA expression of 17β-hydroxysteroid dehydrogenase type 2 (HSD17β2), which is implicated in the back reversal of the steroidogenesis pathway, was significantly lower than in the ovary (21.93 ± 2.23%, P < .001). The 3 isoforms of the 5α reductase (3-oxo-5α-steroid 4-dehydrogenase types 1, 2, and 3; SRD5A1, SRD5A2, SRD5A3, respectively), which are involved in the conversion of T to DHT, showed a significantly higher mRNA expression than ovarian tissue, with SRD5A2 as the most abundant (SRD5A1: 440.93 ± 47.45%; SRD5A2: 17 715.29 ± 2475.49%; SRD5A3: 504.89 ± 64.65%; all P < .001). Of note, aromatase (CYP19A1) mRNA expression was significantly lower in the vagina than in the ovary (5.62 ± 0.46%, P < .001), just at the limit of detection (Fig. 3).
Finally, the mRNA expression of the steroid sulfatase (STS), which catalyzes the conversion of DHEAS to DHEA, was significantly higher than the ovary (233.30 ± 18.79%, P < .001), whereas the mRNA expression of the sulfotransferase 2A1 (SULT2A1), which catalyzes the inverse reaction, was lower than in the ovary, albeit not reaching statistical significance (50.20 ± 19.81%, P = .136) (Fig. 3).
mRNA expression of steroidogenesis-related genes in hvSMCs
As observed in the vaginal tissue, in hvSMCs the main steroidogenic enzymes required for the initial steps of steroid synthesis (STAR, CYP11A1, CYP17A1) and the aromatase gene (CYP19A1) were expressed at very much lower levels than the ovarian tissue (STAR: 4.09 ± 0.64%, P < .001; CYP11A1: 1.17 ± 0.20%, P < .001; CYP17A1: 2.96 ± 0.35%, P < .05; CYP19A1: 3.36 ± 0.26%, P < .001) (Fig. 4). HSD3β1 and HSD3β2, involved in conversion of DHEA into Δ4-androstenedione, showed relatively abundant mRNA levels, although still significantly lower than those observed in the ovary (HSD3β1: 11.67 ± 1.89%; HSD3β2: 14.85 ± 3.05%; both P < .001) (Fig. 4).
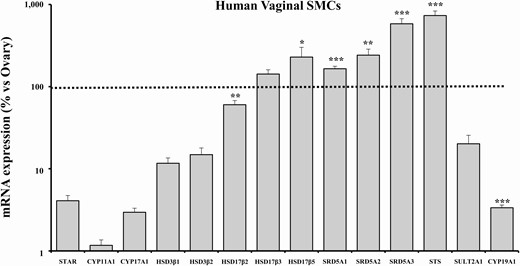
mRNA expression of steroidogenesis pathway-related genes in human vaginal SMCs. This figure shows the mRNA expression of genes involved in steroidogenesis in human distal vaginal SMCs derived from laparoscopic biopsies. Data were calculated according to the 2–ΔΔCt comparative method, using ribosomal subunit 18S as the housekeeping gene for normalization. Results are expressed as percentage of ovary mRNA expression and are reported as mean ± SEM of 3 independent experiments performed in triplicate. *P < .05, **P < .01, ***P < .001 vs ovary.
Remarkably, hvSMCs showed relevantly higher mRNA levels, when compared with ovarian tissue, of all the enzymes involved in T and DHT synthesis, namely HSD17β3 (142.14 ± 18.41%, P = .062 vs ovary), HSD17β5 (229.16 ± 71.33%, P < .05 vs ovary), SRD5A1 (165.11 ± 12.15%, P < .001 vs ovary), SRD5A2 (242.03 ± 43.60%, P < .01 vs ovary), and SRD5A3 (582.74 ± 88.02%, P < .001 vs ovary) (Fig. 4).
In hvSMCs, SULT2A1 mRNA expression was lower (20.16 ± 5.41%, P = .076), whereas STS expression was higher (733.75 ± 98.26%, P < .001) than in the ovary (Fig. 4).
Δ4-steroids levels in spent media of DHEA-stimulated hvSMCs
In the second part of this study, we evaluated the production of Δ4-androstenedione (A), T, DHT, E1, and E2 by hvSMCs in the spent medium, after prolonged stimulation (24 and 48 hours) with increasing concentrations of DHEA (ranging from 1 nM to 30 nM). In untreated (NT) hvSMCs, neither A nor T were detected in culture medium at both 24 or 48 hours (negative controls, Fig. 5).

Measurement in mass spectrometry of androstenedione, testosterone and DHT concentrations by LC-MS/MS in the culture media of hvSMCs in response to stimulation with increasing doses of DHEA. (A) Androstenedione concentrations (expressed as nmol/L) measured in the culture media of hvSMCs after stimulation with increasing doses of DHEA (from 1 to 30 nM) at 24 and 48 hours. Results are reported as mean ± SEM of 3 independent experiments performed in triplicate. (B) Testosterone concentrations (expressed as nmol/L) measured in the culture media of hvSMCs after stimulation with increasing doses of DHEA (from 1 to 30 nM) at 24 and 48 hours. Results are reported as mean ± SEM of 3 independent experiments performed in triplicate. (C) DHT concentrations (expressed as nmol/L) measured in the culture media of hvSMCs after stimulation with increasing doses of DHEA (from 1 nM to 1 µM) and physiological/supraphysiological doses of T (0.6 and 30 nM) at 48 hours. Results are reported as mean ± SEM of 3 independent experiments performed in triplicate.°°°P < .001 vs NT, *P < .05, ***P < .001 vs all lower DHEA doses (nM); ^^^P < .001 vs T (0.6 nM).
As reported in Fig. 5 A, DHEA induced a dose- and time-dependent elevation of A in the medium culture compared with NT. At both 24 and 48 hours, the highest concentration of DHEA (30 nM), increased A levels to close to physiological, circulating, ones (2.77 ± 0.15 nM and 6.64 ± 0.95 nM, respectively).
As reported in Fig. 5 B, DHEA incubation also induced T secretion in a dose- and time-dependent manner. In particular, in the spent hvSMC medium, T was detectable, in a dose-dependent fashion, after 24 hours at the highest DHEA doses only, while after longer incubation (48 hours) T was detectable at all DHEA concentrations. At 48 hours, DHEA 30 nM stimulates a near-physiological level of T (0.52 ± 0.02 nM). In these experiments, DHT was detectable in the culture medium of hvSMCs only after 48 hours of treatment with DHEA 30 nM. To demonstrate that DHT production was related to DHEA stimulation, we performed a further experiment increasing the dose of DHEA to 100 nM and 1 µM for 48 hours. As reported in Fig. 5 C, upon such a high-dose DHEA incubation, DHT showed a dose-dependent increase in the culture medium. Figure 5C also shows the effect of T stimulation (0.6 nM and 30 nM) on DHT secretion (positive control). Upon T stimulation, there was a dose-dependent DHT rise in the spent medium
With regard to estrogens, E2 was not detected at any DHEA or T concentration, while E1 was barely detectable (0.02 nM) upon DHEA (10 nM) stimulation at 48 hours, but did not further increase with DHEA concentration up to 1 µM (data not shown).
mRNA expression of AR target genes and PR after DHEA and testosterone stimulation
We then tested the ability of increasing concentrations of DHEA to induce mRNA expression of AR target genes (STAMP2, GUCY1A2, PKG1) and PR in hvSMCs as a readout of androgen- and estrogen-dependent genes, respectively.
We also tested the effect on androgen-responsive genes of a near-physiological dose of T (0.6 nM), which was also close to the expression induced at 48 hours by DHEA 30 nM treatment. The ability of pretreatment with 1 µM bicalutamide, a nonsteroidal AR antagonist, to counteract either DHEA or T effects was also evaluated.
As shown in Fig. 6, we found that 48-hour treatments with the highest doses of DHEA (10 and 30 nM) induced a significant STAMP2 mRNA expression in hvSMCs compared with NT (both P < .001). A remarkably higher effect was observed after 48-hour stimulation with T (0.6 nM) compared with all DHEA treatments (P < .01). Bicalutamide completely counteracted these effects, promoting a net reduction in the mRNA expression of STAMP2, both in DHEA- and in T-treated cells (P < .01 and P < .01 vs DHEA and T alone, respectively). Likewise, a similar pattern was observed in 2 other androgen-responsive genes, namely GUCY1A2 (guanylate cyclase soluble subunit alpha-2) and PKG1 (cGMP-dependent protein kinase 1). In contrast, none of the tested treatments was able to modulate mRNA expression of PR.
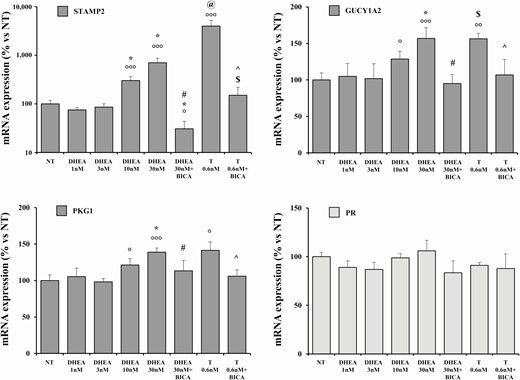
mRNA expression of androgen responsive genes and PR in human vagina hSMCs. The figure shows the mRNA expression of PKG1, GUCY1A2, STAMP2, and PR in human distal vaginal hSMCs derived from laparoscopic biopsies. Data were calculated according to the 2–ΔΔCt comparative method, using ribosomal subunit 18S as the housekeeping gene for normalization. Results are reported as mean ± SEM of 3 independent experiments performed in triplicate. °P < .05, °°°P < .001 vs NT; *P < .05 all lower DHEA doses (nM); #P < 0.01 vs DHEA 30 nM; @P < 0.01 vs all DHEA treatments; $P < .05 vs DHEA 30 nM+ bicalutamide; ^P < 0.01 vs T 0.6 nM.
Discussion
The present study shows, for the first time, that human vaginal tissue harvested from postmenopausal women possesses the machinery to convert an extremely weak androgenic precursor, DHEA, into the active androgens T and DHT. This evidence definitely substantiates the construct of intracrinology in the human vagina, and highlights the androgen-producing ability of this tissue. In fact, we here have evidence of a high abundance of mRNA levels of steroidogenic enzymes downstream to HSD3β, actively involved in androgen production in the human vagina, as compared to the ovary—the most important steroidogenic organ of the female urogenital tract. Noteworthy, we found not only the mRNA expression, but also the activity of these enzymes: indeed, by using LC MS/MS—the gold standard method for steroid measurement (23)—we observed that hvSMCs were able to convert DHEA into its main androgenic metabolites, in a time- and dose-dependent manner. When treating hvSMCs with doses of DHEA in the physiological range (10 and 30 nM), T and Δ4-androstenedione were detected in their culture mediums, at levels very close to those physiologically observed in circulation in females (9, 24). Specifically, T was detected in the culture medium starting from 10 nM DHEA stimulation at 24 hours and, after 48 hours, at all tested DHEA concentrations. This suggests that T production from DHEA in hvSMCs occurs through the conversion of Δ4-androstenedione, requiring 2 different enzymatic reactions (catalyzed by HSD3β1/2 and HSD17β3/5, respectively), and, therefore, with a longer incubation time. In addition, we found extremely high mRNA expression levels of all 3 5α-reductase isoenzymes, which are able to convert T into the most potent AR agonist, DHT. In contrast, aromatase (CYP19A1), which is able to convert T into 17β-estradiol, was almost undetectable in both vaginal tissue and hvSMCs. As a result, both DHEA and T stimulations induced a dose-dependent production of DHT after 48 hours, while estrogens were absent (E2), or slightly detectable (E1), in the culture medium, independently of the dose of DHEA. The lack of E2 production is of particular importance in terms of understanding the potential downstream effects of either T or DHEA.
In both vaginal tissue and isolated hvSMCs, compared with ovarian tissue, the main enzymes required for the initial steps of steroidogenesis (STAR, CYP11A1, CYP17A1) were expressed at very low levels (less than 10% of the ovary expression). Compared with the ovary, HSD3β1 and HSD3β2 mRNA expression in vaginal tissue was 17.4% and 18.5%, respectively. The other HSD17β isoforms—that counteract androgen production: HSD17β3 and HSD17β5—and the 3 isoforms of 5α-reductase were expressed at even higher levels than in the ovary. In parallel, a high expression of STS, along with a low expression of SULT2A1, was detected in the human vagina. STS is known to cleave the sulfate moiety off DHEA sulfate (DHEAS), converting it into DHEA, while SULT2A1 catalyzes DHEA inactivation, through its sulfation. These data suggest that the vagina of postmenopausal women does not possess the ability to de novo synthesize DHEA, but it is able to locally produce active androgens, starting from physiological concentrations of DHEA or even from Δ4-androstenedione.
In the present study, we also confirm that the human vagina is one of the female-specific target sites for androgens (8, 25). ERs are expressed on the whole female genital tract, especially in the vaginal epithelium, stroma, and SMCs. Similarly, the AR is diffusely located in the female genitourinary tract, with a predominance in those areas derived from the urogenital sinus, namely the bladder, urethra, clitoris, and distal vagina (26, 27). The AR is located on all vaginal compartments, including mucosa, submucosa, stroma, smooth muscle layer, and the endothelium, thus hypothetically mediating the direct effect of androgens on genitourinary and vaginal tissues (8).
Indeed, analyzing the mRNA expression of sex steroid receptors, AR expression immediately followed ERα abundance in the whole vaginal tissue and was the most expressed in isolated hvSMCs. This discrepancy between the tissue as a whole and isolated hvSMCs might be due to the different distribution of sex steroid receptors within the vaginal layers. Indeed, ERα is mainly located in the vaginal epithelium, whereas AR seems to be equally expressed in both the epithelial and the stromal compartments (8, 28, 29).
Based on present results, we hypothesized that the ability of the human vagina to locally produce active androgens could represent an intracrine mechanism to regulate in situ androgen-dependent signaling. To strengthen this hypothesis, we showed that increasing concentrations of DHEA promoted a dose-dependent increase in the mRNA expression of STAMP2, a strongly androgen-regulated gene (30), in hvSMCs. A similar, but 5-fold higher effect, was obtained by stimulating hvSMCs with a physiological dose of T (0.6 nM). Pretreatment with AR antagonist bicalutamide abrogated DHEA- and T-induced expression of STAMP2, indicating a pivotal role for AR in the observed results. It is well known that downregulation of STAMP2 induces inflammation, while its upregulation is able to prevent inappropriate activation of inflammatory pathways (31-33). More importantly, we recently demonstrated that androgens exert potent anti-inflammatory effects in the human vagina, also increasing STAMP2 expression (14). Similarly to STAMP2, both DHEA and T treatments upregulated—with bicalutamide normalizing these effects—the mRNA expression of GUCY1A2 and PKG1, 2 genes involved in nitric oxide signaling that are androgen responsive, as previously demonstrated in rat clitoris SMCs (27). However, a direct effect of DHEA on AR is not conceivable, since the tested doses (ranging between 1 and 30 nM) are 3- and 2-log units lower, respectively, than the DHEA affinity constant (Kd) of this steroid for the AR (34-36). This observation further suggests the need of DHEA conversion into a more active androgen in order to stimulate AR-related pathways more effectively.
Conversely, the mRNA expression of PR, an estrogen-dependent gene, was not modulated by DHEA and T stimulation, indicating their predominant androgenic vs. estrogenic effect on hvSMCs. This finding is in line with the almost undetectable expression of aromatase (CYP19A1), both in vaginal tissue and in isolated hvSMCs.
Taken together, these data are highly relevant from several points of view.
The results of this study improve our knowledge of vagina physiology by providing evidence for the presence of steroidogenic machinery in the human vagina, thus supporting, for the first time, the construct of intracrinology in this organ (5, 37).
They also reinforce the view that the vagina is an androgen-dependent organ, and appears to be able to locally produce these hormones, mainly thanks to a high level of steroidogenic enzymes involved in androgen production.
Finally, the observations here reported suggest the potential mechanism and resulting action of intravaginally administered DHEA, which has recently appeared on the market for the treatment of GSM (8, 38, 39), and open new perspectives for the treatment of this common and bothersome condition.
Our study has some limitations. First, we evaluated only vaginal tissue biopsies from menopausal women. In addition, we did not evaluate the steroidogenic pathway and DHEA effects on vaginal epithelial cells, which are known to highly express sex steroid receptor genes. Further studies are warranted to establish the effect and the role of vaginal steroidogenesis in premenopausal women and in cells of other vaginal layers, such as the epithelium, in order to completely understand the DHEA conversion pathway in the human vagina. For example, immunohistochemistry data in human vaginal biopsies would allow us to discern the cell types where intracrine androgen production and response may be most important. Moreover, to further confirm the role of intracrinology in this experimental model, it would be of interest to use either cholesterol as the substrate or some conversion enzyme inhibitors (such as 5-alpha reductase inhibitors) in DHEA- and T-treated cells. Finally, the use of enzalutamide, an AR signaling inhibitor that binds AR with 5-fold higher affinity than bicalutamide, merits further investigation.
The main strength of this study is the novelty of the findings obtained on human vaginal cells and human tissues. Indeed, previous studies have demonstrated the role of androgenic pathways only in animal models (13, 40).
Finally, our data confirm that the human vagina is an androgen synthesis and target organ, supporting a more substantiated use of local androgens in the GSM.
Abbreviations
- AR
androgen receptor
- DHEA
dehydroepiandrosterone
- DHT
dihydrotestosterone
- E1
estrone
- E2
estradiol
- ER
estrogen receptor
- GSM
genitourinary syndrome of menopause
- HSD
hydroxysteroid dehydroxynase
- hvSMC
human vagina smooth muscle cell
- LC-MS/MS
liquid chromatography tandem-mass spectrometry
- NT
untreated
- RT-PCR
reverse transcription-polymerase chain reaction
- STAR
steroidogenic acute regulatory protein
- STS
steroid sulfatase
- T
testosterone
Acknowledgments
Financial Support: This research was supported by a Progetti di rilevante Interesse Nazionale (PRIN) grant (PRIN: 2017S9KTNE) from Ministero dell’Istruzione, dell’Università e della Ricerca (MIUR) to GR.
Additional Information
Disclosures: The authors declare that they have no conflict of interest.
Data Availability
All data generated or analyzed during this study are included in this published article. All datasets generated and analyzed during the current study are not publicly available but are available from the corresponding author upon reasonable request. Restrictions apply to some or all of the availability of data generated or analyzed during this study to preserve patient confidentiality. The corresponding author will, upon request, detail the restrictions and any conditions under which access to some data may be provided.
References
Author notes
Equally contributed.