-
PDF
- Split View
-
Views
-
Cite
Cite
Ahmed E. El Zowalaty, Rong Li, Yi Zheng, John P. Lydon, Francesco J. DeMayo, Xiaoqin Ye, Deletion of RhoA in Progesterone Receptor–Expressing Cells Leads to Luteal Insufficiency and Infertility in Female Mice, Endocrinology, Volume 158, Issue 7, 1 July 2017, Pages 2168–2178, https://doi.org/10.1210/en.2016-1796
- Share Icon Share
Abstract
Ras homolog gene family, member A (RhoA) is widely expressed throughout the female reproductive system. To assess its role in progesterone receptor-expressing cells, we generated RhoA conditional knockout mice RhoAd/d (RhoAf/f-Pgr-Cre+/−). RhoAd/d female mice had comparable mating activity, serum luteinizing hormone, prolactin, and estradiol levels and ovulation with control but were infertile with progesterone insufficiency, indicating impaired steroidogenesis in RhoAd/d corpus luteum (CL). RhoA was highly expressed in wild-type luteal cells and conditionally deleted in RhoAd/d CL. Gestation day 3.5 (D3.5) RhoAd/d ovaries had reduced numbers of CL, less defined corpus luteal cord formation, and disorganized CL collagen IV staining. RhoAd/d CL had lipid droplet and free cholesterol accumulation, indicating the availability of cholesterol for steroidogenesis, but disorganized β-actin and vimentin staining, indicating disrupted cytoskeleton integrity. Cytoskeleton is important for cytoplasmic cholesterol movement to mitochondria and for regulating mitochondria. Dramatically reduced expression of mitochondrial markers heat shock protein 60 (HSP60), voltage-dependent anion channel, and StAR was detected in RhoAd/d CL. StAR carries out the rate-limiting step of steroidogenesis. StAR messenger RNA expression was reduced in RU486-treated D3.5 wild-type CL and tended to be induced in progesterone-treated D3.5 RhoAd/d CL, with parallel changes of HSP60 expression. These data demonstrated the in vivo function of RhoA in CL luteal cell cytoskeleton integrity, cholesterol transport, StAR expression, and progesterone synthesis, and a positive feedback on StAR expression in CL by progesterone signaling. These findings provide insights into mechanisms of progesterone insufficiency.
Ras homolog gene family, member A (RhoA) is a small GTPase protein in the Rho family of GTPases. The GTP-bound active RhoA transduces downstream signaling cascades to regulate various fundamental cellular functions, such as migration, adhesion, cytokinesis, stress fiber formation, apical constriction, vesicle trafficking, survival, proliferation, and gene expression (1–3). Besides some redundant roles of RhoA with RhoB and RhoC in regulating cytoskeletal organization and cell-extracellular matrix (ECM) interaction in certain cell types, mouse genetic models have been revealing essential in vivo cell-type specific functions of RhoA signaling, such as protection of heart from injuries, regulation of lens epithelium morphology, regulation of hematolymphoid system, and diverse functions in different parts of the nervous system [reviewed by Zhou and Zheng (2)].
Defined in vivo functions of RhoA in the female reproductive system are largely unexplored despite implications of RhoA signaling in tissues/cells from vagina, uterus, and ovary (4–13). RhoA expression was increased in the vaginal wall of patients with pelvic organ prolapse (4). Our microarray analysis revealed high levels of RhoA messenger RNA (mRNA) expression in the peri-implantation mouse uterine luminal epithelium (5). The RhoA/ROCK pathway may regulate F-actin remodeling in human endometrial adhesiveness (6), and RhoA in decidual cells may facilitate embryo development and differentiation after attachment (7) in in vitro embryo implantation models. RhoA signaling might be implicated in endometriosis (8) and in uterine contractility (9–11). RhoA mRNA expression in the ovary was induced by an endocrine disruptor (DBP)di-2-ethly hexyl phthalate (13). The RhoA/ROCK/actomyosin pathway was shown to mediate chemokine-induced integrin activation and ECM assembly in cumulus cells after ovulation (12). It was unknown if RhoA played a role in the corpus luteum (CL) after ovulation.
The CL is a transient endocrine gland formed in the ruptured follicle after ovulation that produces progesterone (P4) to prepare the uterus for embryo implantation. Ovulation is triggered by the surge of luteinizing hormone (LH) from the pituitary, which activates LH receptors in theca cells and granulosa cells of preovulatory follicles in humans (14), and it is also regulated by other luteotrophic hormones such as prolactin and estradiol in rodents (15). Upon ovulation, the oocyte with cumulus cells is released from the mature follicle, and the remaining ruptured follicle immediately forms corpus hemorrhagicum (16) and subsequently forms CL upon trauma healing. The majority cells in CL are vascular endothelial cells and luteal cells (17, 18).
P4 is essential for establishing and maintaining pregnancy in all mammals. It is mainly synthesized in the CL during early pregnancy. The precursor for P4 synthesis is cholesterol. The main source of cholesterol is through endocytosis or selective uptake and a minor source is through de novo cholesterol synthesis in luteal cells (15). Cholesterol in cytoplasmic lipid droplets moves along the cytoskeleton to reach mitochondria. The rate-limiting step of steroidogenesis is the transport of cholesterol from outer to inner mitochondrial membrane, a step that is carried out by steroidogenic acute regulatory protein (StAR) (15, 19). P4 synthesis in CL involves the conversion of cholesterol to pregnenolone by P450 side chain cleavage (P450SCC/CYP11A1) on the inner mitochondrial membrane and the conversion of pregnenolone to P4 by 3β-hydroxysteroid dehydrogenase in the smooth endoplasmic reticulum (15). Both mitochondria and smooth endoplasmic reticulum undergo dramatic changes in organization and increases in quantity for P4 synthesis (15).
RhoA has critical cellular functions (1–3) and has been widely detected in the female reproductive system (4–13), but its in vivo functions in female reproduction have not been established. Therefore, it was hypothesized that RhoA played critical roles in female reproduction. To test the hypothesis and determine in vivo functions of RhoA in female reproduction, RhoAf/f mice (2, 20) and Pgr-Cre mice (21) were bred to generate RhoA conditional deletion mice RhoAd/d (RhoAf/f-Pgr-Cre+/−). Analyses of RhoAd/d female mice led us to the identification of an in vivo function of RhoA in CL steroidogenesis.
Materials and Methods
Animals and genotyping
RhoA floxed (RhoAf/f) mice were derived from Dr. Zheng’s colony at the University of Cincinnati (2, 20). Progesterone receptor-Cre (Pgr-Cre) mice were derived from Dr. DeMayo and Dr. Lydon’s colony at Baylor College of Medicine (21). They were crossed in the College of Veterinary Medicine animal facility at University of Georgia to generate RhoAf/f-Pgr-Cre+/− (RhoAd/d) mice. Polymerase chain reaction (PCR) was used to genotype the mice. Primers P1 (5′-atgtttagctggcccaaatg-3′), P2 (5′-tataccgatctccctggacg-3′), and P3 (5′-cccaaagagacaccaggaag-3′) were used to amplify the Pgr wild-type (WT) allele (285 bp) and the Cre allele (590 bp) (22). Primers P4 (5′-tctctgcactgagggagttagg-3′) and P5 (5′-gtacatacagggaatggaaacaagg-3′) were used to identify RhoA WT allele (482 bp) and floxed allele (633 bp) (23). All mice were maintained on PicoLab mouse diet 20. They were housed in polypropylene cages with free access to food and water on a 12-hour light/dark cycle (0600 hours to 1800 hours) at 23 ± 1°C with 30% to 50% relative humidity. All methods used in this study were approved by the University of Georgia Institutional Animal Care and Use Committee and conform to National Institutes of Health guidelines and federal law.
Mating behavior and fertility tests
Virgin WT (n = 8) and RhoAd/d (n = 8) female mice (2 to 3 months old) were housed with stud WT male mice. Each female mouse was checked for the presence of a copulation plug every morning to determine mating activity during the previous night. The day of vaginal plug detection was designated as gestation day (D) 0.5 (mating night as D0). The plugged female mice were separated from the male mice. Plugging rate and plugging latency (the duration from cohabitation to detection of the first copulation plug) were used to evaluate mating behavior. Delivery rate and litter size at birth were used to assess fertility.
Pseudopregnancy
Pseudopregnant WT female mice were generated by mating WT female mice with Bscl2−/− male mice that had normal mating activity but were infertile (24).
Embryo implantation and P4 treatment to rescue embryo implantation
Embryo implantation on D4.5 WT (n = 6) and RhoAd/d (n = 4) female mice (2 to 3 months old) mated with WT stud male mice was determined using Evan’s blue dye injection as previously described (25). RhoAd/d female mice mated with WT stud male mice were injected subcutaneously with 4 mg/mouse/d of progesterone (P0130; Sigma-Aldrich, St. Louis, MO) at 1100 hours from D2.5 to D6.5, and implantation sites were detected on D7.5.
Natural ovulation and superovulation
Natural ovulation was done in young virgin WT and RhoAd/d female mice (2 to 3 months old) mated with WT stud male mice. On the day of vaginal plug detection (D0.5), female mice were euthanized, and oocytes were counted from both oviducts. Superovulation was performed in two sets of female mice to determine ovulation in adult female mice (first set) and RhoA regulation by ovulation in immature female mice (second set). In the first set, 4-month-old adult WT (n = 5) and RhoAd/d (n = 4) female mice were intraperitoneally injected with 10 IU gonadotropin from pregnant mare serum (eCG, G4877; Sigma-Aldrich) followed by 10 IU human chorionic gonadotropin (hCG) (NC0234619; Fisher Scientific, Hampton, NH) 48 hours later. The oocytes were recovered from oviduct 16 hours after hCG injection as previously described (26). In the second set, immature postnatal day (PND)21 WT female mice (n = 13) were intraperitoneally injected with 5 IU eCG followed by 5 IU hCG 48 hours later. The mice were randomly assigned into three groups and killed 4 hours, 8 hours, and 24 hours after hCG injection. The ovaries were flash frozen. One ovary from each mouse was used for RNA extraction and real-time PCR, and the other was sectioned for immunohistochemistry.
Serum P4 and 17β-estradiol measurement
D3.5 WT (n = 12) and RhoAd/d (n = 9) female mice (2 to 4 months old) were anesthetized at ∼1100 hours for blood collection via orbital sinus. Serum was collected after clotting at room temperature for 90 minutes and stored at −80°C. Serum P4 and 17β-estradiol (E2) were measured in the Ligand Assay and Analysis Core of the Center for Research in Reproduction at the University of Virginia (Charlottesville, VA).
Serum LH and prolactin measurement
Serum samples from 2- to 3-month-old WT (n = 7) and RhoAd/d (n = 4) female mice on proestrus stage ∼18:00 hours (27, 28) were collected as described previously for LH measurement. Proestrus stage was determined by monitoring vaginal smear as previously described (26, 29). Serum samples from WT (n = 14) and RhoAd/d (n = 6) on D1.5 at ∼1800 hours, when there is a peak in diurnal prolactin secretion, were collected for prolactin measurement (30–32). LH and prolactin were measured at Oregon National Primate Research Center, Oregon Health & Science University.
Ovary histology
D3.5 ovaries from WT (n = 8) and RhoAd/d (n = 5) female mice (2 to 4 months old) were fixed in Bouin’s solution (Ricca Chemical Company, Arlington, TX) for 24 hours and then kept in 70% ethanol until embedding in paraffin. Each ovary was sectioned through and every tenth section (5 µm) was collected, processed, and stained with hematoxylin and eosin.
Number of corpora lutea
All the histology sections from one ovary per mouse were examined and the total number of corpora lutea from each ovary was counted. In addition, the number of corpora lutea, which can be indicated by collagen IV (Col IV) immunofluorescence staining, was also counted in a section crossing the widest part of an ovary each from another set of D3.5 WT (n = 11) and RhoAd/d (n = 11) female mice. The numbers of CL were counted by two people, and consistent results were obtained.
Immunohistochemistry and immunofluorescence
Frozen sections (10 µm) of WT and RhoAd/d uteri and ovaries were processed for immunohistochemistry and immunofluorescence as previously described (24). The dilutions of each primary antibody are listed in Table 1. The secondary antibodies used were biotinylated goat anti-rabbit IgG (1:200, 7.5 μg/mL, BA-1000; Vector Laboratories, Burlingame, CA) for immunohistochemistry and Alexa Fluor 568 goat anti-mouse and Alexa Fluor 488 goat anti-rabbit antibodies (1:200; Life Technologies, Grand Island, NY) for immunofluorescence. Sections from immunofluorescence were counterstained and mounted in 4′,6′-diamino-2-phenylindole (DAPI)-containing Vectashield (Vector Laboratories). Negative controls were RhoAd/d uterine or ovary sections processed in parallel as WT sections described previously and WT uterine or ovary sections processed in parallel as WT sections described previously except without primary antibody. Sections from at least three different mice in each group were examined. The fluorescence of heat shock protein 60 (HSP60) staining in CL was quantified using ImageJ (24).
Peptide/Protein Target . | Antigen Sequence (if Known) . | Name of Antibody . | Manufacturer, Catalog No. . | Species Raised in; Monoclonal or Polyclonal . | Dilution Used . | RRID . |
---|---|---|---|---|---|---|
Transforming protein RhoA | RhoA (67B9) Rabbit mAb #2117 | Cell Signaling Technology, RhoA (67B9) Rabbit mAb #2117 | Rabbit; monoclonal | 1:200 | AB_10693922 | |
Collagen α-1(IV) chain | Anti-Collagen IV | Abcam, ab19808 | Rabbit; polyclonal | 1:200 | AB_445160 | |
Proliferating cell nuclear antigen | PCNA (D3H8P) XP® Rabbit mAb #13110 | Cell Signaling Technology, PCNA (D3H8P) XP® Rabbit mAb #13110 | Rabbit; monoclonal | 1:1000 | AB_2636979 | |
Actin, cytoplasmic 1 | Antibeta Actin | Abcam, ab8227 | Rabbit; polyclonal | 1:200 | AB_2305186 | |
60-kDa heat shock protein, mitochondrial | HSP60 (D6F1) XP® Rabbit mAb #12165 | Cell Signaling Technology, HSP60 (D6F1) XP® Rabbit mAb #12165 | Rabbit; monoclonal | 1:400 | AB_2636980 | |
Voltage-dependent anion-selective channel protein 1 | VDAC (D73D12) Rabbit mAb | Cell Signaling Technology, VDAC (D73D12) Rabbit mAb #4661 | Rabbit; monoclonal | 1:500 | AB_10557420 | |
Vimentin | Vimentin Antibody (E-5) | Santa Cruz Biotechnology, sc-373717 | Mouse; monoclonal | 1:500 | AB_10917747 |
Peptide/Protein Target . | Antigen Sequence (if Known) . | Name of Antibody . | Manufacturer, Catalog No. . | Species Raised in; Monoclonal or Polyclonal . | Dilution Used . | RRID . |
---|---|---|---|---|---|---|
Transforming protein RhoA | RhoA (67B9) Rabbit mAb #2117 | Cell Signaling Technology, RhoA (67B9) Rabbit mAb #2117 | Rabbit; monoclonal | 1:200 | AB_10693922 | |
Collagen α-1(IV) chain | Anti-Collagen IV | Abcam, ab19808 | Rabbit; polyclonal | 1:200 | AB_445160 | |
Proliferating cell nuclear antigen | PCNA (D3H8P) XP® Rabbit mAb #13110 | Cell Signaling Technology, PCNA (D3H8P) XP® Rabbit mAb #13110 | Rabbit; monoclonal | 1:1000 | AB_2636979 | |
Actin, cytoplasmic 1 | Antibeta Actin | Abcam, ab8227 | Rabbit; polyclonal | 1:200 | AB_2305186 | |
60-kDa heat shock protein, mitochondrial | HSP60 (D6F1) XP® Rabbit mAb #12165 | Cell Signaling Technology, HSP60 (D6F1) XP® Rabbit mAb #12165 | Rabbit; monoclonal | 1:400 | AB_2636980 | |
Voltage-dependent anion-selective channel protein 1 | VDAC (D73D12) Rabbit mAb | Cell Signaling Technology, VDAC (D73D12) Rabbit mAb #4661 | Rabbit; monoclonal | 1:500 | AB_10557420 | |
Vimentin | Vimentin Antibody (E-5) | Santa Cruz Biotechnology, sc-373717 | Mouse; monoclonal | 1:500 | AB_10917747 |
Abbreviation: RRID, research resource identifier.
Peptide/Protein Target . | Antigen Sequence (if Known) . | Name of Antibody . | Manufacturer, Catalog No. . | Species Raised in; Monoclonal or Polyclonal . | Dilution Used . | RRID . |
---|---|---|---|---|---|---|
Transforming protein RhoA | RhoA (67B9) Rabbit mAb #2117 | Cell Signaling Technology, RhoA (67B9) Rabbit mAb #2117 | Rabbit; monoclonal | 1:200 | AB_10693922 | |
Collagen α-1(IV) chain | Anti-Collagen IV | Abcam, ab19808 | Rabbit; polyclonal | 1:200 | AB_445160 | |
Proliferating cell nuclear antigen | PCNA (D3H8P) XP® Rabbit mAb #13110 | Cell Signaling Technology, PCNA (D3H8P) XP® Rabbit mAb #13110 | Rabbit; monoclonal | 1:1000 | AB_2636979 | |
Actin, cytoplasmic 1 | Antibeta Actin | Abcam, ab8227 | Rabbit; polyclonal | 1:200 | AB_2305186 | |
60-kDa heat shock protein, mitochondrial | HSP60 (D6F1) XP® Rabbit mAb #12165 | Cell Signaling Technology, HSP60 (D6F1) XP® Rabbit mAb #12165 | Rabbit; monoclonal | 1:400 | AB_2636980 | |
Voltage-dependent anion-selective channel protein 1 | VDAC (D73D12) Rabbit mAb | Cell Signaling Technology, VDAC (D73D12) Rabbit mAb #4661 | Rabbit; monoclonal | 1:500 | AB_10557420 | |
Vimentin | Vimentin Antibody (E-5) | Santa Cruz Biotechnology, sc-373717 | Mouse; monoclonal | 1:500 | AB_10917747 |
Peptide/Protein Target . | Antigen Sequence (if Known) . | Name of Antibody . | Manufacturer, Catalog No. . | Species Raised in; Monoclonal or Polyclonal . | Dilution Used . | RRID . |
---|---|---|---|---|---|---|
Transforming protein RhoA | RhoA (67B9) Rabbit mAb #2117 | Cell Signaling Technology, RhoA (67B9) Rabbit mAb #2117 | Rabbit; monoclonal | 1:200 | AB_10693922 | |
Collagen α-1(IV) chain | Anti-Collagen IV | Abcam, ab19808 | Rabbit; polyclonal | 1:200 | AB_445160 | |
Proliferating cell nuclear antigen | PCNA (D3H8P) XP® Rabbit mAb #13110 | Cell Signaling Technology, PCNA (D3H8P) XP® Rabbit mAb #13110 | Rabbit; monoclonal | 1:1000 | AB_2636979 | |
Actin, cytoplasmic 1 | Antibeta Actin | Abcam, ab8227 | Rabbit; polyclonal | 1:200 | AB_2305186 | |
60-kDa heat shock protein, mitochondrial | HSP60 (D6F1) XP® Rabbit mAb #12165 | Cell Signaling Technology, HSP60 (D6F1) XP® Rabbit mAb #12165 | Rabbit; monoclonal | 1:400 | AB_2636980 | |
Voltage-dependent anion-selective channel protein 1 | VDAC (D73D12) Rabbit mAb | Cell Signaling Technology, VDAC (D73D12) Rabbit mAb #4661 | Rabbit; monoclonal | 1:500 | AB_10557420 | |
Vimentin | Vimentin Antibody (E-5) | Santa Cruz Biotechnology, sc-373717 | Mouse; monoclonal | 1:500 | AB_10917747 |
Abbreviation: RRID, research resource identifier.
Quantitative reverse transcription-PCR
The ovaries from the second set of superovulation were processed for total RNA extraction using Trizol (Life Technologies). Complementary DNA was transcribed using Superscript III reverse transcription (Life Technologies) with random primers. Quantitative PCR was performed in 384-well plates using Ssoadvanced SYBR Green Supermix (Bio-Rad, Hercules, CA) on CFX384 TouchReal-Time PCR Detection System (Bio-Rad). RhoA specific primers RhoAe4F (5′-CTGGACAGGAAGATTATGACC-3′) and RhoAe6R (5′-AGGCACCCAGACTTTTTCT-3′) (Integrated DNA Technology, Coralville, IA) were used to quantify RhoA mRNA expression. Glyceraldehyde-3-phosphate dehydrogenase was used as a loading control, and hypoxanthine phosphoribosyltransferase 1 served as a second control as previously described (5).
In situ hybridization
Sense and antisense cRNA probes synthesis and in situ hybridization were carried out as previously described (5). StAR primers were StARe4F (5′-AGCCCATGGACAGACTCTA-3′) and StARe7R (5′-TAGGACCTGGTTGATGATTG-3′). Ovaries from at least three different mice were examined for each time point.
Lipid droplet staining and free cholesterol staining
Sections (10 μm) were fixed in 4% paraformaldehyde at room temperature for 5 minutes, washed twice in 1× PBS, and covered with 1.5 μg/mL Nile red (N3013; Sigma-Aldrich) in 1× PBS at room temperature for 15 minutes to detect lipid droplets. Sections were then washed in 1× PBS and counterstained with DAPI. The fluorescent areas of randomly selected lipid droplets were quantified using ImageJ (24). Free cholesterol was detected by Filipin (F9765; Sigma-Aldrich) staining, and nuclei were counter stained with ethidium bromide (33).
RU486 and P4 treatments
Young adult WT female mice were mated with WT stud male mice and randomly split into two groups (n = 5) for vehicle and RU486 (400 µg/mouse, a progesterone receptor antagonist in the presence of P4 and a partial agonist in the absence of P4) injections, respectively, around noon on D1.5 and D2.5. Young adult RhoAd/d female mice were mated with WT stud male mice and randomly split into two groups (n = 3) for vehicle and P4 (2 mg/mouse) injections, respectively, around noon on D1.5 and D2.5. All the mice were dissected around noon on D3.5, and the ovaries were snap frozen for StARin situ hybridization as well as Col IV and HSP60 immunofluorescence. The StAR signal intensity in CL was quantified as follows: each CL was assigned a score from 0 to 3, and the average score from all CLs in each mouse represented one data point.
Statistical analysis
Data are presented as means ± SD where applicable. Wilcoxon rank sum test was used for plugging latency and for serum LH and prolactin levels. For parameters with percentage, two-tailed Fisher exact test was used. Two-tailed unequal variance Student t test was used for the other quantitative data. Significance level was set at P < 0.05.
Results
Infertile RhoAd/d female mice with progesterone insufficiency
RhoAd/d (RhoAf/f-Pgr-Cre+/−) female mice mated with WT stud male mice had 100% plugging rate and comparable plugging latency with their age matched WT female mice [Supplemental Fig. 1(A)]. However, none of the plugged RhoAd/d female mice (n = 6) delivered any pups, whereas 80% of the plugged WT female mice (n = 10) delivered litters with a litter size of 7.0 ± 2.0 (n = 8) at birth. Because the body weight of all mated RhoAd/d female mice did not show significant changes during the 3 weeks after mating, whereas the pregnant WT female mice had steady increases of body weight, which reached >60% increase at D18.5 over the weight at D0.5 [Supplemental Fig. 1(B)], it indicated early pregnancy failure in the RhoAd/d female mice, which was confirmed by embryo implantation failure in the D4.5 RhoAd/d uteri [Supplemental Fig. 1(C)]. Proliferating cell nuclear antigen (PCNA) immunostaining revealed positive proliferating cells in the stromal cells but not in the uterine epithelium in D4.5 pseudopregnant WT uteri [Supplemental Fig. 1(D)], whereas D4.5 RhoAd/d uteri had proliferating cells mainly detected in the epithelium but not the stromal cells [Supplemental Fig. 1(E)]. This uterine cell proliferation pattern in the D4.5 RhoAd/d uteri resembled that in D0.5 and D1.5 WT uteri (34) before the dramatic increase of progesterone (P4) during early pregnancy (27), suggesting that there might be P4 deficiency in the RhoAd/d female mice. Indeed, a significant reduction of serum P4 levels was detected in the RhoAd/d female mice compared with WT female mice on D3.5 [Fig. 1(a)] when P4 level reaches a plateau during early pregnancy (27). No difference in E2 levels was detected between these two groups [Fig. 1(a)]. Exogenous P4 treatment restored embryo implantation in RhoAd/d female mice, but the average number of implantation sites (3.8 ± 1.5; n = 6) was significantly lower than that in the natural pregnant WT control mice (10.3 ± 0.5; n = 4; P < 0.0001). These data demonstrated P4 deficiency in the RhoAd/d female mice.
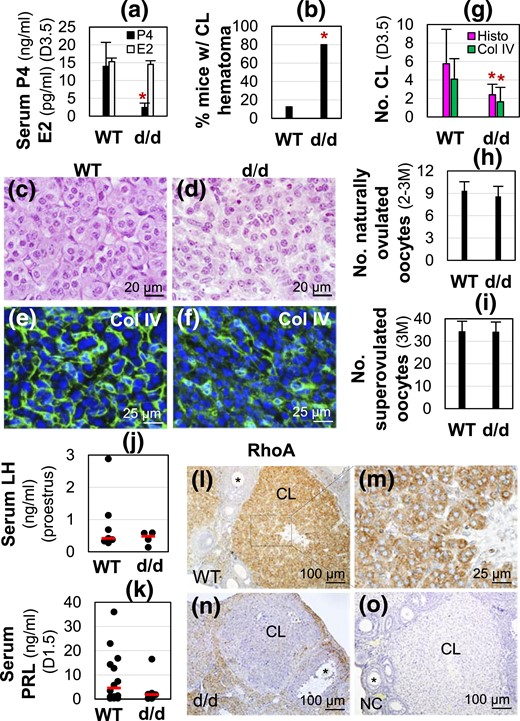
RhoAd/d (d/d) female infertility, hormonal profiles, and RhoA expression in CL. (a) Serum progesterone (P4, ng/mL) and estradiol (E2, pg/mL) levels in D3.5 female mice (n = 9 to 12). *P < 0.05. Error bar indicates standard deviation. (b) Percentage of mice with CL hematoma (n = 5 to 8). (c) WT D3.5 ovary histology. (d) D3.5 RhoAd/d (d/d) ovary histology. (e and f) Collagen IV immunofluorescence in (e) D3.5 WT and (f) RhoAd/d CL. (c–f) Images with lower magnifications are shown in Supplemental Fig. 2. (g) Average total number of CL per ovary in D3.5 ovaries by histology (Histo) and average number of CL per ovary from D3.5 ovary sections by collagen IV (Col IV) staining (n = 5 to 11). (h) Number of naturally ovulated oocytes per mouse from 2- to 3-month-old female mice detected on D0.5 (n = 5 to 9). (i) Number of superovulated oocytes per mouse from 3-month-old (3M) female mice (n = 4 or 5). In (a, g, h and i), *P < 0.05. Error bar indicates standard deviation. (j) Serum LH levels on proestrus stage (n = 4 to 7). (k) Serum prolactin (PRL) levels on D1.5 at 1800 hours (n = 6 to 14). (j and k) Each dot represents one individual mouse (2 to 3 months old); the red line indicates the median in each group. (l–o) RhoA immunohistochemistry. (l) RhoA expression in D3.5 WT ovary. (m) Enlarged from boxed area in (l) showing RhoA expression in luteal cells. (n) RhoA expression in D3.5 RhoAd/d ovary. (o) Negative control (NC) of a D3.5 WT ovarian section.
Defective CL function but normal ovulation in RhoAd/d ovary
Because P4 is mainly produced in CL during early pregnancy, P4 insufficiency in the RhoAd/d female mice was an indication of defective CL. Histological sections throughout the entire D3.5 ovaries showed the presence of follicles at different stages and CL in each ovary in both WT and RhoAd/d groups [Supplemental Fig. 2(A1) and 2(B1)]. There was an increased percentage of mice with CL hematoma in the RhoAd/d ovaries [Fig. 1(b)]. The only CL with a possible hematoma in the WT female mice (1/8) appeared to narrow down at the center of the CL [Supplemental Fig. 2(A2)], whereas the ones in the D3.5 RhoAd/d ovaries were bigger [Supplemental Fig. 2(B2)]. Hematoma was not observed in every CL of RhoAd/d ovaries with hematoma [Supplemental Fig. 2(B1)]. However, all D3.5 RhoAd/d CL had less defined corpus luteal cord formation compared with D3.5 WT CL [Fig. 1(c) and 1(d); Supplemental Fig. 2(A2) and 2(B2)].
Because the CL is highly vascularized, disrupted corpus luteal cord formation seen in RhoAd/d CL histology might be associated with disorganized vasculature. Immunofluorescence of Col IV that was detected in CL but not in follicles [Supplemental Fig. 2(C1)] revealed organized Col IV staining surrounding luteal cell cords in D3.5 WT CL [Supplemental Fig. 2(C1) and 2(C2); Fig. 1(e)], whereas Col IV staining in the RhoAd/d CL was overall decreased and disorganized [Supplemental Fig. 2(D1) and 2(D2); Fig. 1(f)].
Based on the histological sections throughout the ovaries, the average total number of CL per ovary was reduced in the D3.5 RhoAd/d ovaries (n = 5 to 8) [Fig. 1(g)]. The number of CL was also determined in one section through the middle of each ovary immunostained with Col IV [Supplemental Fig. 2(C1–D2)]. The results from Col IV staining (n = 11) were consistent with histology that showed that D3.5 RhoAd/d ovaries had reduced numbers of CL [Fig. 1(g)].
Ovulation is a prerequisite for true CL formation. RhoAd/d female mice had normal mating activity [Supplemental Fig. 1(A)], suggesting normal neuroendocrine function in these mice. In addition, comparable numbers of naturally ovulated oocytes per mouse [Fig. 1(h)] and superovulated oocytes per mouse [Fig. 1(i)] as well as comparable serum LH levels on proestrus [Fig. 1(j)] and prolactin levels on D1.5 [Fig. 1(k)] between WT and RhoAd/d female mice (2 to 3 months old) demonstrated normal ovulation in RhoAd/d female mice. These data suggested an important role of local RhoA in CL development and function.
RhoA expression and regulation in CL
Immunohistochemistry showed the highest level of RhoA expression in CL and lower levels of RhoA expression in different stages of follicles, medulla, and interstitial compartment of D3.5 WT ovary [Fig. 1(l)]. In the CL, RhoA was mainly detected in the luteal cells [Fig. 1(m)]. RhoA expression was dramatically reduced in the D3.5 RhoAd/d CL [Fig. 1(n)]. No specific staining was detected in the negative control [Fig. 1(o)].
To determine the regulation of RhoA expression upon ovulation, superovulation was performed on PND21 WT mice. All the newly weaned female mice had eCG injection on PND21. Two days later, they received hCG injection. RhoA mRNA was barely detectable 4 hours after hCG injection. Its level increased dramatically 8 hours after hCG injection and was maintained through 24 hours after hCG injection [Supplemental Fig. 3(A)]. Immunohistochemistry also showed RhoA upregulation from 4 hours to 8 hours after hCG injection, and higher RhoA expression appeared to be in the cortex than in the medulla of the ovary 24 hours after hCG injection [Supplemental Fig. 3(B–D)]. The high levels of RhoA expression in the luteal cells and its upregulation by eCG+hCG injection suggested local function(s) of RhoA in the CL.
Lipid droplet accumulation in RhoAd/d CL
To dissect the mechanism of RhoA in P4 synthesis in CL, the availability of cholesterol was examined. Nile Red staining of D3.5 ovaries showed bright dots, which represented lipid droplets that contain cholesterol, in both WT and RhoAd/d follicles [Supplemental Fig. 4(A1–B2)]. Nile Red staining was relatively even with fine lipid droplets in all WT CL from three female mice examined [Supplemental Fig. 4(A1–A4)] except one CL that showed bright dots [Supplemental Fig. 4(A1) and (A4)]. Nile Red staining in all CL from five RhoAd/d female mice examined showed bright dots that were larger than those seen in the WT [Supplemental Fig. 4(B1) and 4(B2); Fig. 2(a–c)]. Free cholesterol detected by filipin also showed stronger staining in the RhoAd/d CL [Supplemental Fig. 4(C1–D2); Fig. 2(d) and 2(e)], indicating no lack of cholesterol in RhoAd/d luteal cells for P4 synthesis.

(a–c) Nile red staining of lipid droplets and (d, e) filipin staining of free cholesterol in gestation D3.5 (a and d) WT and (b and e) RhoAd/d ovaries. In (a and b), green indicates Nile red staining, blue indicates DAPI staining nuclei, and inserts are confocal images. (c) Quantification of fluorescent areas of lipid droplets (n = 32 to 41 randomly selected lipid droplets). *P < 0.05. Error bar indicates standard deviation. In (d and e), blue indicates filipin staining, and red indicates EtBr staining nuclei. (a, b, d, and e) Images with lower magnifications are provided in Supplemental Fig. 4.
Disrupted cytoskeleton in RhoAd/d luteal cells
A main cellular function of RhoA is to regulate cytoskeleton (35), which plays an important role in intracellular transport of lipid droplets (36). Immunofluorescence showed β-actin as relatively strong and even staining with fine granules, which are most likely cytoplasmic β-actin messenger ribonucleoprotein granules (37), in the cytoplasm of WT luteal cells but overall weaker and uneven with more prominent granules in the cytoplasm of RhoAd/d luteal cells [Supplemental Fig. 5(A1–B2); Fig. 3(a) and 3(b)]. Similar expression patterns of vimentin staining were also seen in the cytoplasm of WT and RhoAd/d luteal cells, except that no obvious granules were present [Supplemental Fig. 5(C1–D2)]. Col IV double staining in vimentin-labeled sections confirmed disorganized luteal cords in the RhoAd/d CL [Fig. 3(c) and 3(d)]. These data demonstrated disrupted cytoskeleton integrity in the RhoAd/d luteal cells.
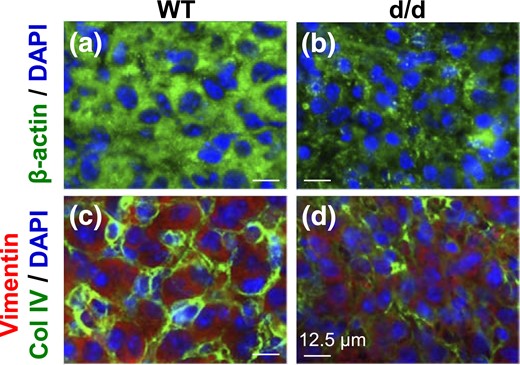
Immunofluorescence detection of (a and b) β-actin and (c and d) vimentin in D3.5 (a and c) WT corpus luteum and (b and d) RhoAd/d corpus luteum. In (a and b), green indicates β-actin, and blue indicates DAPI staining nuclei. In (c and d), green indicates Col IV, red indicates vimentin, and blue indicates DAPI staining nuclei. Scale bar, 12.5 µm. (a–d) Images with lower magnifications are provided in Supplemental Fig. 5.
Severe reduction of mitochondrial density in RhoAd/d CL
Because cytoskeleton plays important roles in regulating mitochondria (38, 39), it was hypothesized that disrupted cytoskeleton in the RhoAd/d luteal cells affected mitochondrial density. To test this hypothesis, mitochondrial markers HSP60 and voltage-dependent anion channel (VDAC) were used to label mitochondria in the CL using immunofluorescence and immunohistochemistry, respectively. Consistent results indicated HSP60 labeling in the ovaries of all D3.5 WT mice examined (n = 3), with the strongest labeling in the CL and interstitial compartment, whereas HSP60 labeling in the follicles was much weaker [Fig. 4(a1) and 4(a2)]. However, the strongest HSP60 labeling was detected in the interstitial compartment, weaker staining in the follicles, and the weakest staining in the CL of all D3.5 RhoAd/d female mice examined (n = 5) [Fig. 4(b1) and 4(b2)]. Confocal images showed mitochondrial labeling as abundant bright dots surrounding the nuclei of WT luteal cells [Fig. 4(a3)], whereas those in the RhoAd/d luteal cells were much less abundant [Fig. 4(b3) and 4(c)]. The CL localizations in RhoAd/d ovaries were verified by Col IV staining of CL in a neighboring section and by DAPI staining of both sections for HSP60 staining and Col IV staining that showed less tightly organized and larger nuclei compared with the nuclei in the follicles (Supplemental Fig. 6). Consistent results were also revealed in VDAC staining, which showed much stronger staining in the WT CL [Fig. 4(a4)] than in the RhoAd/d CL [Fig. 4(b4)]. These results demonstrated a dramatic reduction of mitochondrial density in the RhoAd/d CL.
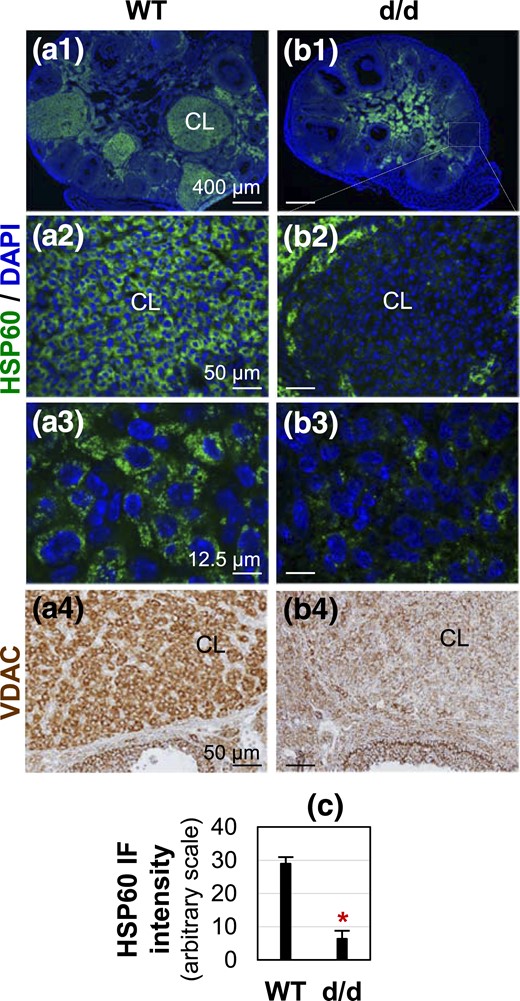
Detection of mitochondrial markers HSP60 and VDAC in D3.5 (a) WT and (b) RhoAd/d corpora lutea. (a1–b3) Detection of HSP60 by immunofluorescence. HSP60 staining is shown in green; DAPI staining is shown in blue. (a3 and b3) Confocal images of CL from sections in (a1) and (b1), respectively. (a4–b4) Detection of VDAC by immunohistochemistry. VDAC staining is in brown. Scale bars, (a1 and b1) 400 µm, (a2 and b2) 50 µm, (a3 and b3) 12.5 µm, and (a4 and b4) 50 µm. (c) Quantification of HSP60 immunofluorescence intensity in D3.5 WT and RhoAd/d CL (n = 3 to 5). *P < 0.05. Error bar indicates standard deviation.
Dramatic downregulation of StAR mRNA expression in RhoAd/d CL
Besides the availability of cholesterol in the CL, the next essential step and also the rate-limiting step for P4 synthesis in CL is the transport of cholesterol from outer membrane to inner membrane of mitochondria by StAR (15, 19). In situ hybridization revealed that StAR mRNA expression was highly and specifically detected in D3.5 WT CL [Fig. 5(a1) and 5(a2)] but was barely detectable in all ovary sections with CL from four D3.5 RhoAd/d female mice [Fig. 5(b1–b3)]. No specific signals were detected in WT CL with a StAR sense probe [Fig. 5(c)]. The dramatic reduction of StAR expression implied insufficient cholesterol transported to the inner mitochondria for P4 synthesis and agreed with the reduced serum P4 levels in D3.5 RhoAd/d female mice [Fig. 1(a)].
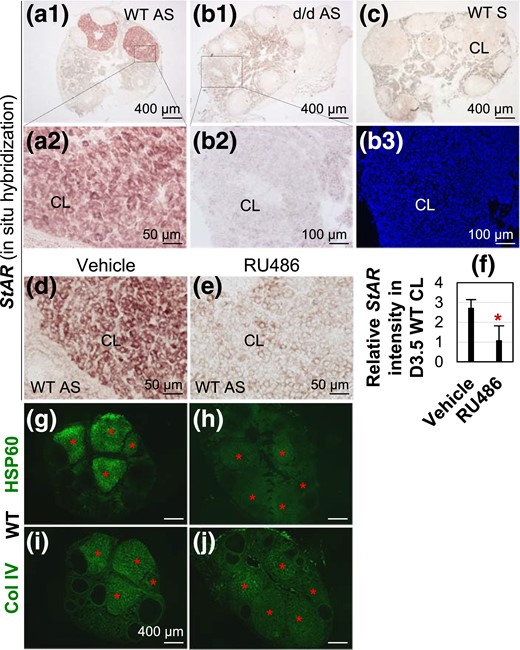
Detection of StAR mRNA expression in D3.5 ovaries by in situ hybridization and regulation of StAR mRNA by RU486 AS, StAR antisense probe; S, StAR sense probe for negative control. (a1) StAR expression in D3.5 WT CL. (a2) Enlarged from the boxed area in A1. (b1) StAR expression in D3.5 RhoAd/d CL. (b2) Enlarged from the boxed area in B1. (b3) DAPI staining of the section in B2. (c) Negative control using WT section and StAR sense probe. (d) StAR expression in D3.5 vehicle-treated WT CL. (e) StAR expression in D3.5 RU486-treated WT CL. (f) Relative StAR expression intensity in D3.5 vehicle and RU486-treated RhoAd/d CL (n = 5). *P < 0.05. Error bar indicates standard deviation. (g and h) Expression of HSP60 in D3.5 vehicle- and RU486-treated WT CL. (i and j) Col IV staining of a nearby section each to the sections in (g) and (h), respectively, to confirm the positions of CL. Red star, CL.
In vivo regulation of StAR mRNA levels by P4
P4 was shown to induce StAR expression in Leydig cell line MA-10 (40) and StAR mRNA expression in the injured spinal cord (41). Does P4 signaling regulate StAR expression in CL in vivo? To test the hypothesis that StAR was upregulated by P4 signaling in vivo and the reduction of P4 in RhoAd/d female mice [Fig. 1(a)] also contributed to the downregulation of StAR in the RhoAd/d CL [Fig. 5(a1–b3)], two mouse models were used: mated WT female mice treated with RU486 and mated RhoAd/d female mice treated with P4. RU486 treatment significantly reduced StAR expression and the expression of mitochondrial marker HSP60 in D3.5 WT CL [Fig. 5(d–j)]. P4 treatment tended to increase StAR expression and HSP60 expression in the RhoAd/d CL (Supplemental Fig. 7). These data demonstrated in vivo upregulation of StAR and mitochondrial density in the CL by P4 signaling and that the reduction of P4 in RhoAd/d female mice weakened such a positive feedback on P4 synthesis.
Discussion
The dominant cell types in the CL are luteal cells and endothelial cells (17, 42). RhoA signaling is involved in angiogenesis (43, 44), and angiogenesis is essential for CL development (17). Because RhoA is specifically and highly expressed in the WT luteal cells where it is deleted in the RhoAd/d CL, it is reasonable to conclude that the phenotypes observed in the RhoAd/d CL are mainly contributed by the function of RhoA in the luteal cells. Because P4 may act on vascular endothelial cells in CL (45), the disorganized Col IV staining, an indication of disorganized vasculature ECM, in the RhoAd/d CL could be a secondary phenotype from disorganized luteal cells and reduced P4 production. Because there are defective corpus luteal cord formation and dramatically reduced mitochondria density in the D3.5 RhoAd/d CL, it is possible that RhoAd/d granulosa and theca cells in some ovulated follicles fail to differentiate into luteal cells, resulting in reduced CL numbers. Because ECM remodeling is important for folliculo-luteal transition (46), the disorganized ECM indicated by disorganized Col IV staining may also impede luteal cell differentiation, leading to reduced CL numbers.
Although it had not been previously reported about any in vivo function of RhoA in steroidogenesis in the CL, RhoA signaling plays critical roles in regulating the dynamics of all three main types of cytoskeletal filaments in eukaryotic cells [microtubules (47), intermediate filaments (48), and actin microfilaments (49)], and cytoskeletons are important for steroidogenesis, with which the majority information has been derived from adrenal cells (38, 50–54). Several studies have demonstrated the involvement of cytoskeleton in ovarian steroidogenesis. For example, there was reduced P4 production in vimentin-deficient (Vim−/−) mice as well as in Vim−/− luteinized granulosa cells treated with high-density lipoprotein and cyclic adenosine monophosphate (51, 55), indicating a role of the intermediate filament vimentin in ovarian steroidogenesis; treatments of isolated porcine luteal cells with microtubule- and microfilament-altering agents revealed that both microtubules and microfilaments play an important role in LH-stimulated P4 synthesis in luteal cells (53). Therefore, the role of RhoA in the CL is most likely associated with its function in regulating cytoskeleton dynamics.
To make cholesterol available for the first step of steroidogenesis in the mitochondria, the lipid droplets that store free cholesterol and cholesteryl ester (38, 56) have to be transported to the mitochondria outer membrane. It has been demonstrated in the adrenal cells that both lipid droplets and mitochondria are attached to intermediate filaments that cross-link actin microfilaments (38, 52). Reorganization of the cytoskeleton facilitates the interaction of lipid droplets with mitochondria to make cholesterol available for steroid synthesis in mitochondria (52). The altered expression of microfilament β-actin and intermediate filament vimentin in the RhoAd/d CL demonstrates the critical in vivo role of RhoA in cytoskeleton function in luteal cells. The disrupted cytoskeleton in RhoAd/d CL could lead to impaired transport of lipid droplets to their proper destinations (e.g., mitochondria, for expenditure, resulting in lipid droplet accumulation in the cytoplasm).
Mitochondria are very dynamic organelles that continually undergo fission and fusion, and their morphology and quantity can change dramatically (57). The cristae of luteal mitochondria are more tubulovesicular in appearance and the sizes of luteal mitochondria are much larger than those of follicular mitochondria (58, 59), in addition to increased mitochondrial quantity in luteal cells (15). These changes accommodate significant increase of P4 synthesis. Cytoskeleton plays critical roles in mitochondrial morphology, motility, and distribution (60–63), and actin cytoskeleton is involved in regulating mitochondrial quantity via mitochondrial fission/division (38, 39). The disrupted cytoskeleton in the RhoAd/d CL is associated with significantly reduced expression of mitochondria markers, indicating reduced mitochondrial density in the RhoAd/d CL and an essential role of RhoA in regulating mitochondrial dynamics.
StAR is primarily expressed in steroid-producing cells, such as luteal cells in the ovary, Leydig cells in the testis, and cell types in the adrenal cortex, to regulate the rate-limiting step in steroidogenesis (64). Multiple factors could potentially contribute to the dramatically reduced StAR expression in the RhoAd/d CL. One such factor is the availability of cholesterol on the outer mitochondrial membrane: the disrupted cytoskeleton in RhoAd/d CL hinders the transport of lipid droplets to mitochondria, resulting in reduced level of cholesterol on mitochondria. Therefore, a reduced level of StAR would be required to transport cholesterol from outer to inner mitochondrial membrane, which may lead to reduced level of StAR detected in RhoAd/d CL. Another contributing factor is mitochondrial density. Because StAR protein is targeted to mitochondria, dramatically reduced mitochondrial density would limit the level of StAR in RhoAd/d CL. A final contributing factor is positive feedback regulation of StAR by P4: a significantly reduced P4 level in the D3.5 RhoAd/d serum weakens such a positive feedback.
Positive feedback regulation of StAR expression by P4 in CL in vivo has not been previously reported. Several lines of evidence have implied connections between P4 and StAR expression. Progesterone receptor is expressed in the luteal cells (65), supporting P4 as a local luteotropin in the developing CL, possibly involved in regulating StAR mRNA expression. P4 and progestin R5020, but not other steroids, induced StAR expression in Leydig cell line MA-10 (40). P4 treatment increased StAR mRNA expression in the injured spinal cord (41). However, another study indicated that P4 treatment decreased StAR mRNA expression in ex vivo fathead minnow ovary (66). Decreased StAR mRNA expression in RU486-treated WT CL and increased StAR mRNA expression in P4-treated RhoAd/d CL in this study demonstrate the role of P4 signaling in feedback upregulation of StAR mRNA expression in vivo and potential involvement of progesterone receptor in such upregulation.
This study using RhoAd/d mice reveals an in vivo function of RhoA in luteal cells. It is proposed that RhoA regulates cytoskeleton to facilitate cytoplasmic lipid droplet movement and thus cholesterol transport to mitochondria as well as to increase mitochondrial density in the luteal cells. These processes may contribute to the upregulation of StAR, which transports cholesterol into inner mitochondrial membrane for P4 synthesis. P4 provides positive feedback upregulation of StAR and mitochondrial density to further facilitate steroidogenesis. The RhoA signaling pathways involved in these processes and the molecular mechanisms of P4 feedback regulation of StAR transcription in luteal cells remain to be elucidated. A potential role of uterine RhoA in embryo implantation is being investigated. These findings provide insights into a local factor RhoA in regulating luteal cell progesterone synthesis and female reproduction.
Abbreviations:
- CL
corpus luteum
- Col IV
collagen IV
- D
gestation day
- DAPI
4′,6′-diamino-2-phenylindole
- E2
17β-estradiol
- ECM
extracellular matrix
- hCG
human chorionic gonadotropin
- HSP60
heat shock protein 60
- LH
luteinizing hormone
- mRNA
messenger RNA
- P4
progesterone
- PCNA
proliferating cell nuclear antigen
- PCR
polymerase chain reaction
- PND
postnatal day
- RhoA
Ras homolog gene family, member A
- VDAC
voltage-dependent anion channel
- WT
wild-type.
Acknowledgments
The authors thank the Office of the Vice President for Research, the Interdisciplinary Toxicology Program, and the Department of Physiology and Pharmacology at the University of Georgia, and Jun Zhou for participating in the initial mouse breeding.
This work was supported by National Institutes of Health Grants R15HD066301 and R01HD065939 (co-funded by the Office of Research on Women's Health and the Eunice Kennedy Shriver National Institute of Child Health and Human Development) (to X.Y.). Serum P4 and E2 levels were determined at The University of Virginia Center for Research in Reproduction Ligand Assay and Analysis Core, which is supported by Eunice Kennedy Shriver National Institute of Child Health and Human Development/National Institutes of Health (National Centers for Translational Research in Reproduction and Infertility) Grant P50-HD28934.
Author contributions: X.Y. conceived the project; A.E.Z. and X.Y. designed the experiments; A.E.Z. performed the majority of the experiments; R.L. counted the corpora lutea; Y.Z. provided the RhoAf/f mouse strain; J.P.L. and F.J.D. provided the Pgr-Cre mouse strain; X.Y. supervised the entire project; A.E.Z. and X.Y. analyzed the data and wrote the manuscript.
Disclosure Summary: The authors have nothing to disclose.
References
Taggart MJ, Arthur P, Zielnik B, Mitchell BF. Molecular pathways regulating contractility in rat uterus through late gestation and parturition. Am J Obstet Gynecol. 2012;207:76 e15-24. 10.1016/j.ajog.2012.04.036
Author notes
Address all correspondence and requests for reprints to: Xiaoqin Ye, MD, PhD, 501 DW Brooks Dr., Department of Physiology and Pharmacology, College of Veterinary Medicine; Interdisciplinary Toxicology Program, University of Georgia, Athens, Georgia 30602. E-mail: [email protected].