-
PDF
- Split View
-
Views
-
Cite
Cite
Luan Wen, Yuki Shibata, Dan Su, Liezhen Fu, Nga Luu, Yun-Bo Shi, Thyroid Hormone Receptor α Controls Developmental Timing and Regulates the Rate and Coordination of Tissue-Specific Metamorphosis in Xenopus tropicalis, Endocrinology, Volume 158, Issue 6, 1 June 2017, Pages 1985–1998, https://doi.org/10.1210/en.2016-1953
- Share Icon Share
Abstract
Thyroid hormone (T3) receptors (TRs) mediate the effects of T3 on organ metabolism and animal development. There are two TR genes, TRα and TRβ, in all vertebrates. During animal development, TRα expression is activated earlier than zygotic T3 synthesis and secretion into the plasma, implicating a developmental role of TRα both in the presence and absence of T3. Using T3-dependent amphibian metamorphosis as a model, we previously proposed a dual-function model for TRs, in particular TRα, during development. That is, unliganded TR represses the expression of T3-inducible genes during premetamorphosis to ensure proper animal growth and prevent premature metamorphosis, whereas during metamorphosis, liganded TR activates target gene transcription to promote the transformation of the tadpole into a frog. To determine if TRα has such a dual function, we generated homozygous TRα-knockout animal lines. We show that, indeed, TRα knockout affects both premetamorphic animal development and metamorphosis. Surprisingly, we observed that TRα is not essential for amphibian metamorphosis, given that homozygous knockout animals complete metamorphosis within a similar time period after fertilization as their wild-type siblings. On the other hand, the timing of metamorphosis for different organs is altered by the knockout; limb metamorphosis occurs earlier, whereas intestinal metamorphosis is completed later than in wild-type siblings. Thus, our studies have demonstrated a critical role of endogenous TRα, not only in regulating both the timing and rate of metamorphosis, but also in coordinating temporal metamorphosis of different organs.
Thyroid hormone (T3) receptors (TRs) mediate the biological effects of T3 during vertebrate development and in organ physiology and pathology. TRs are members of the nuclear hormone receptor superfamily, which also includes 9-cis retinoic acid receptors. TRs bind to T3 response elements (TREs) in target genes and regulate their transcription in a T3-dependent manner (1–6). For T3-inducible genes, TRs function mainly as heterodimers with retinoic acid receptors, repressing target gene expression in the absence of T3, and activating target gene expression when T3 is available by recruiting corepressors and coactivators, respectively (3, 7–11). Although these dual functions of TRs have been well established in vitro and in cell cultures, few studies have addressed the role of unliganded TRs during vertebrate development.
We and others have used amphibian development as a model to study TR function during vertebrate development (3, 12–15). Anurans, such as the highly related frog species Xenopus laevis and X. tropicalis, undergo a T3-dependent metamorphosis to transform from tadpoles into frogs. This involves drastic changes in essentially every organ and tissue of the animal (13). Earlier molecular and transgenic studies in X. laevis have indicated that TR is necessary and sufficient to mediate the metamorphic effects of T3, and that corepressor recruitment by unliganded TR regulates the timing for the onset of metamorphosis (stage 54) (16–30). With the development of transcription activatorlike effector nuclease (TALEN) and clustered regularly interspaced short palindromic repeat/associated protein-9 nuclease technologies for editing endogenous genes in X. laevis and X. tropicalis (31, 32), it became possible to investigate the role of endogenous TRs during Xenopus development.
As in other vertebrates, there are only two known TR genes, TRα and TRβ, in amphibians, although both genes are duplicated in the pseudotetraploid X. laevis (33, 34). Of the TR genes, TRα is expressed prior to the maturation of the thyroid gland during development in vertebrates, including mammals and amphibians (5, 30, 34–39). Interestingly, Xenopus TRα expression reaches high levels by the end of embryonic development, when a free-feeding tadpole is formed, well before the onset of metamorphosis at stage 54, whereas the TRβ gene has little expression during embryogenesis or premetamorphosis but is strongly activated by T3 during metamorphosis (5, 16, 34, 39–41). These findings suggest that TRα most likely functions as unliganded TR in premetamorphic tadpoles and binds to T3-inducible genes to repress their expression prior to metamorphosis, whereas both TRα and TRβ participate in the activation of these T3 target genes when T3 becomes available during metamorphosis (35, 42). Consistently, chromatin-immunoprecipitation (ChIP) assays have shown that TRs indeed bind constitutively to the promoter regions of T3-induced genes in premetamorphic as well as metamorphosing tadpoles (17, 18).
To investigate the role of endogenous TRα during frog development, we and the Buchholz laboratory previously made use of the newly developed, TALEN-mediated in vivo gene mutation technology to generate TRα-knockdown tadpoles of X. tropicalis (43–46), a diploid species that is highly related to the more widely studied X. laevis (34, 47–50). The studies with these F0-generation knockdown animals revealed that the knockdown of TRα enhanced the development of premetamorphic tadpoles while making the tadpoles resistant to T3 treatment, supporting the previously hypothesized roles of TRα. However, the lack of a well-defined, homozygous, total TRα-knockout line prevented the analysis of the role of TRα during natural metamorphosis in these earlier studies. Here, using F0 knockdown animals, we have generated TRα–total knockout animals to investigate the role of TRα in the development of X. tropicalis. Our results have confirmed the earlier observations with the knockdown animals; that is, the homozygous animals initiate metamorphosis earlier but metamorphose more slowly than their wild-type and heterozygous siblings. More importantly, we have several findings to report. First, the total removal of TRα has no observable effect on embryogenesis. Second, and surprisingly, the animals lacking TRα are able to complete metamorphosis, as judged by external morphology (complete resorption of the tail) within an apparently similar developmental time period as the wild-type and TRα heterozygous animals. Last, the knockout also alters the temporal coordination of organ-specific transformations: limb formation is precocious, but intestinal metamorphosis is delayed in the knockout animals compared with the wild-type and heterozygous animals. Thus, TRα, although nonessential, plays a critical role in regulating metamorphosis timing and rate as well as tissue-specific temporal transformations of different organs.
Materials and Methods
Animal rearing and staging
Wild-type adult X. tropicalis were purchased from Nasco. Embryos and tadpoles were staged according to the description for X. laevis (51). All animal care and treatments were performed in accordance with guidelines of the Animal Use and Care Committee of Eunice Kennedy Shriver National Institute of Child Health and Human Development of the National Institutes of Health.
Generation of TRα-knockout tadpoles and genotyping
TALEN-injected X. tropicalis embryos, as described previously (43), were reared to sexual maturity (F0-generation frogs). A sexually mature F0 frog was mated with a wild-type frog, and their offspring were screened to identify TRα heterozygous mutant tadpoles. After TRα heterozygous mutants were sexually mature (F1 frogs), female and male mutant frogs were primed with 20 U of human chorionic gonadotropin (Novarel) one day before egg laying. They were then boosted with 200 U of human chorionic gonadotropin on the second day for natural mating. The resulting fertilized eggs/embryos were collected and reared for three days at 25°C to reach the feeding stage (stage 45). The tadpoles were then transferred to a 2-L container and fed.
Tadpoles were anesthetized with MS222 for photography, tail clipping, and body length measurement. For genotyping, the tadpole’s tail tip or one forelimb digit (for stage-66 animals) was clipped and lysed in 30 to 50 μL QuickExtract DNA extraction solution (Epicentre) at 65°C for 10 to 15 minutes, according to manual instructions. After incubating at 95°C for 5 minutes, 2 μL of the DNA extraction solution was immediately used for genotyping polymerase chain reaction (PCR). For the F1 animals, genotyping was performed by PCR amplification of the TALEN-targeted region, following by sequencing. This led to the identification of heterozygous F1 animals with out-of-frame mutations. The studies presented here were based on one F1 mutant line. Genotyping the offspring of this F1 line was subsequently performed by PCR. The forward primer 5′-ACATCCCCAGCTATCCCCAGCTATG-3′ was designed to detect mutated allele, whereas the forward primer 5′-AGCTATCTGGACAAAGACGAGCCG-3′ was used to detect wild-type allele. Each of the forward primers was mixed with the same reverse primer 5′-GCAAACTTTTTGGCTCAGAGGCCAC-3′ for PCR. For each DNA sample, two sets of PCRs, for detecting the wild-type and mutant allele, respectively, were carried out to determine the genotype with the same PCR program: 94°C for 30 seconds, 68°C for 60 seconds, then repeated for 35 cycles. The PCR products were analyzed by gel electrophoresis. The specificity of the primers was verified by using mutant and wild-type genome DNA for PCR.
T3 treatment
For gene expression analysis, 6-day-old tadpoles were randomly selected and treated with 10 nM T3 for 18 hours at 25°C. The tadpole tail was cut for genotyping, and the rest of each animal was homogenized in 200 μL TRIzol (Life Technologies). The homogenized solutions from tadpoles of the same genotype were mixed together for RNA extraction. For the ChIP assay, 7-day-old tadpoles were genotyped by tail clipping and then reared in 24-well plates; 5 tadpoles for each genotype were pooled together and treated with 10 nM T3 for 18 hours at 25°C.
RNA extraction and quantitative real-time PCR
The intestine, tail, or limb was isolated from animals at indicated stages and homogenized in 500 μL of TRIzol per intestine, tail, or limb, respectively. The homogenates of individual tissues from at least three animals, or three whole animals, of each genotype were mixed together for RNA extraction. Total RNA was extracted with Direct-zol RNA MiniPrep (Zymogen), according to the manufacturer’s instructions. The RNA concentration was measured by using a NanoDrop instrument (Thermo Scientific). The same amount of RNA from the three genotypes (wild-type, heterozygous, and homozygous) was reverse transcribed, respectively, with the QuantiTect reverse transcription kit (Qiagen). Quantitative real-time polymerase chain reaction (qRT-PCR) was carried out by using the SYBR Green method. The PCR primers for genes TH/bzip, TRβA, Klf9, GH1, GH2, and internal control EF1α were described previously (43) (note that EF1α expression is similar in all three TRα genotypes; data not shown). Other primers included forward primer 5′-AAATGCATTGCCGTTGGCAT and reverse primer 5′-GCCGCTCTCGATTCTCTTCA for TRα; forward primer 5′-GGTTATGTGTGGCGCCTTCG-3′ and reverse primer 5′-AATGGGAAAGGGCCCAGAGG-3′ for T3 target gene ST3; forward primer 5′-GAGCACATTGGATGCGGCAA-3′ and reverse primer 5′-TGTGTTCTTGGACCCAAATGCG-3′ for Tbx4; and forward primer 5′-TGCCTGCAGATGACCACAGA-3′ and reverse primer 5′-TAGCAGGTTCAGCCTTGCCC-3′ for Tbx5. All expression data were normalized against that of the internal control gene EF1α. The expression analyses were performed at least twice, with similar results.
ChIP assay and quantitative PCR
Tadpoles at 7 days of age were randomly selected and anesthetized with MS222. For genotyping, the anesthetized tadpoles were subjected to tail clipping and reared in 12-well plates individually. After genotyping, five tadpoles of the same genotype were pooled together and homogenized for ChIP assay with anti-TR, anti-NCoR, and anti-ID14 antibodies to serve as negative controls, as described previously (50, 52) (Table 1). The immunoprecipitated DNA was analyzed by using TaqMan quantitative PCR (Thermo Fisher Scientific) with gene-specific primers/probes for TRβ promoter, TH/bZIP promoter, and exon 5 of TRβ, as previously described (18).
Peptide/Protein Target . | Antigen Sequence (if Known) . | Name of Antibody . | Manufacturer, Catalog No., and/or Name of Individual Providing the Antibody . | Species Raised in; Monoclonal or Polyclonal . | Dilution Used . | RRID . |
---|---|---|---|---|---|---|
ID14 | DKVTPKKDDGATS-KLH, ETKCRCNMDGDVE-MAP | Anti-ID14 | (24, 50)a | Rabbit; polyclonal | 1/100 | AB_2636986 |
TR | Recombinant × l TRb protein | Anti-TR | (24, 34, 50)a | Rabbit; polyclonal | 1/100 | AB_2636985 |
NCoR | Recombinant × l NCoR fragment | Anti-NCoR | (53)a | Rabbit; polyclonal | 1/100 | AB_2636987 |
Peptide/Protein Target . | Antigen Sequence (if Known) . | Name of Antibody . | Manufacturer, Catalog No., and/or Name of Individual Providing the Antibody . | Species Raised in; Monoclonal or Polyclonal . | Dilution Used . | RRID . |
---|---|---|---|---|---|---|
ID14 | DKVTPKKDDGATS-KLH, ETKCRCNMDGDVE-MAP | Anti-ID14 | (24, 50)a | Rabbit; polyclonal | 1/100 | AB_2636986 |
TR | Recombinant × l TRb protein | Anti-TR | (24, 34, 50)a | Rabbit; polyclonal | 1/100 | AB_2636985 |
NCoR | Recombinant × l NCoR fragment | Anti-NCoR | (53)a | Rabbit; polyclonal | 1/100 | AB_2636987 |
Abbreviation: RRID, Research Resource Identifier.
aManufactured in the Shi laboratory. Numbers indicate references.
Peptide/Protein Target . | Antigen Sequence (if Known) . | Name of Antibody . | Manufacturer, Catalog No., and/or Name of Individual Providing the Antibody . | Species Raised in; Monoclonal or Polyclonal . | Dilution Used . | RRID . |
---|---|---|---|---|---|---|
ID14 | DKVTPKKDDGATS-KLH, ETKCRCNMDGDVE-MAP | Anti-ID14 | (24, 50)a | Rabbit; polyclonal | 1/100 | AB_2636986 |
TR | Recombinant × l TRb protein | Anti-TR | (24, 34, 50)a | Rabbit; polyclonal | 1/100 | AB_2636985 |
NCoR | Recombinant × l NCoR fragment | Anti-NCoR | (53)a | Rabbit; polyclonal | 1/100 | AB_2636987 |
Peptide/Protein Target . | Antigen Sequence (if Known) . | Name of Antibody . | Manufacturer, Catalog No., and/or Name of Individual Providing the Antibody . | Species Raised in; Monoclonal or Polyclonal . | Dilution Used . | RRID . |
---|---|---|---|---|---|---|
ID14 | DKVTPKKDDGATS-KLH, ETKCRCNMDGDVE-MAP | Anti-ID14 | (24, 50)a | Rabbit; polyclonal | 1/100 | AB_2636986 |
TR | Recombinant × l TRb protein | Anti-TR | (24, 34, 50)a | Rabbit; polyclonal | 1/100 | AB_2636985 |
NCoR | Recombinant × l NCoR fragment | Anti-NCoR | (53)a | Rabbit; polyclonal | 1/100 | AB_2636987 |
Abbreviation: RRID, Research Resource Identifier.
aManufactured in the Shi laboratory. Numbers indicate references.
Results
X.tropicalis TRα regulates T3 target genes both in the presence and absence of T3 but is not required for embryogenesis
To generate animals lacking functional TRα, we previously showed that microinjecting TALEN messenger RNAs (mRNAs) targeting exon 4 (the third coding exon), which encodes a portion of the DNA binding domain of TRα in one-cell-stage embryos, led to tadpoles (the F0-generation animals) containing mosaic TRα mutations (43). We raised some of these animals to sexual maturity and then crossed them with wild-type animals to generate F1 animals [Fig. 1(a)]. Genotyping of the F1 animals at tadpole stages identified a number of heterozygous F1 animals with out-of-frame TRα mutations. For functional studies, we chose one mutant line, M5, with seven base substitutions and seven base-pair deletions in the TRα gene [Fig. 1(b)], leading to a mutant TRα gene encoding a nonfunctional truncated protein lacking the DNA and ligand binding domains. The heterozygous animals from this M5 line were crossed to generate F2-generation TRα total knockout animals, as confirmed by PCR genotyping [Fig. 1(c)].

Generation of TRα knockout X. tropicalis animals. (a) The procedure to generate TRα-knockout frogs with TALEN. TRα TALEN mRNAs were injected in one-cell–stage embryos. The resulting F0 animals containing mosaic TRα mutations were raised to sexual maturity and then were crossed with wild-type (WT) animals to generate F1 animals. Heterozygous F1 animals with TRα mutations (red) were selected through sequencing at tadpole stages. TRα-knockout animals (red) were generated through crossing these F1 siblings to produce F2-generation animals. Immediately after fertilization, the F2 embryos/animals had two WT copies of the TRα gene (clear animal), one WT and one mutant copy (half-red animal, heterozygous), or two mutant copies (fully red animal, homozygous). (b) Schematic diagram depicting sequences of the TALEN-targeted region in the WT and TRα-mutant line (M5). Arrows represent primers used for genotyping: the forward primer detecting the wild-type (P-wtF) and the mutant sequence (P-m5F), respectively. (c) Representative examples of genotyping by PCR. Two independent sets of PCR were carried out on genomic DNA by using a common reverse primer and P-wtF or P-m5F to detect the WT or mutant sequence, respectively. The presence of only mutant or WT PCR product in the two PCR reactions indicates the animal as a homozygous mutant (Hom) or WT, respectively, whereas the presence of both mutant and WT PCR products indicates a heterozygous mutant (Het).
It has been shown that low levels of TRs are expressed in early embryos, and TRα is strongly activated during late embryogenesis to reach high levels at the end of embryogenesis, although TRβ expression remains very low until metamorphosis (5, 16, 34, 39–41, 54–57). Furthermore, altering TR function has been shown to affect embryonic development (16, 54, 57). Interestingly, total knockout of TRα has no apparent effect on embryogenesis, given that wild-type, heterozygous, and homozygous TRα-mutant animals all completed embryogenesis to become feeding stage (stage 45) tadpoles at the same time (3 days after fertilization) without any detectable morphological difference (data not shown), suggesting that TRα is not essential for embryogenesis and/or that its role is offset by the low-level expression of TRβ.
TRα mRNA reaches high levels by the end of embryogenesis when the feeding begins (stage 45) (5, 34, 39), and TR is known to bind to the TREs of endogenous target genes and recruit corepressors in premetamorphic tadpoles (12). Thus, to investigate the effect of TRα knockout on premetamorphic tadpoles, we first analyzed TR binding and corepressor recruitment to two well-known target genes, TRβ (58) and the transcription factor TH/bzip (59), in 7-day-old premetamorphic tadpoles by ChIP assay. As shown in Fig. 2(a), both TR binding and the recruitment of the corepressor NCoR to the TREs were drastically reduced in the homozygous TRα-knockout animals compared with the wild-type and heterozygous siblings. The remaining TR binding was likely due to the expression of TRβ in the knockout tadpoles.
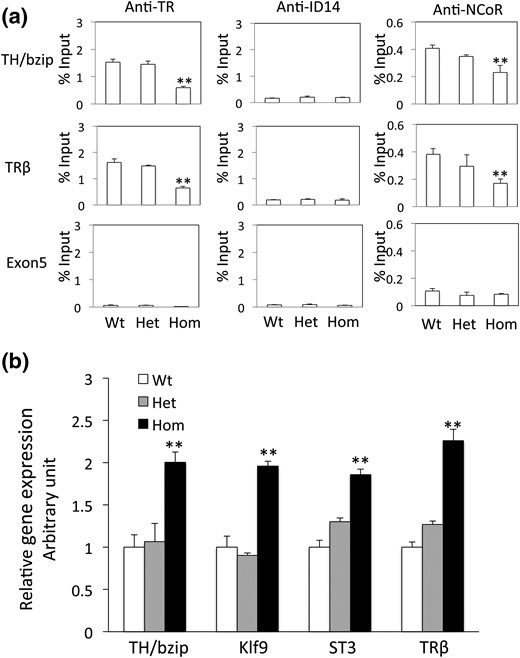
TRα knockout reduces NCoR recruitment and increases target gene expression. (a) ChIP assay reveals reduced TR binding and corepressor recruitment at endogenous TREs in premetamorphic tadpoles. Seven-day-old tadpoles were genotyped, and two days after genotyping, at least five tadpoles of the same genotype were homogenized together for ChIP assays with antibodies against TR, corepressor NCoR, and ID14, an extracellular protein, as a nonspecific control. Note that TRα homozygous knockout dramatically reduced receptor binding or recruitment of the corepressor NCoR at the well-characterized TRE regions of TRβ and TH/bzip genes. Exon 5 of the TRβ gene was also analyzed as a control genomic region lacking TRE. Two asterisks (**) indicate a significant difference between homozygous knockout and the other groups (P < 0.01). (b) TRα knockout increases basal expression of T3 direct target genes in premetamorphic tadpoles. Total RNA from 7-day-old tadpoles was used for real-time PCR analysis of the expression of several well-known T3 direct target genes: TH/bzip (58), Klf9 (60), ST3 (61), and TRβ (57). The expression levels were normalized against that of EF1α. Two asterisks (**) indicate a significant difference between the homozygous knockout and other groups (P < 0.01).
Consistent with the reduced TR binding and corepressor recruitment, we also observed that the expression of several known, direct TR target genes was much higher in the 7-day-old premetamorphic homozygous TRα-knockout tadpoles compared with the wild-type and heterozygous siblings [Fig. 2(b)], suggesting that TRα participated in the repression of these genes during premetamorphosis, when there is little T3.
We next investigated how the knockout animals responded to T3 treatment. We treated 6-day-old, randomly selected tadpoles with 10 nM T3 for 18 hours. Both T3-treated and control tadpoles were euthanized at the same time. The tail tip was clipped for genotyping, and the rest of each animal was homogenized for RNA extraction and expression analysis. As shown in Fig. 3(a), the induction of all direct target genes analyzed was reduced by threefold or more in the homozygous knockout animals compared with the controls. Consistently, when we performed ChIP assays on the T3-treated animals, we observed that homozygous TRα-knockout animals had significantly reduced TR binding to the TREs at endogenous target genes [Fig. 3(b)], indicating that TRα knockout reduced animal response to T3 treatment due to reduced TR binding to target genes, even in the presence of T3. Thus, TRα knockout increases basal expression in premetamorphic tadpoles but reduces the activation of target genes by T3.

TRα-knockout tadpoles are resistant to T3-induced precocious metamorphosis. (a) TRα knockout reduces T3 induction of target gene expression in premetamorphic tadpoles. Six-day-old tadpoles were treated with 10 nM T3 for 18 hours. Both T3-treated and control tadpoles were euthanized at the same time. The tail tip was clipped for genotyping and the rest of each animal was homogenized for RNA extraction. At least three animals of the same genotype were mixed together for RNA isolation and subsequent expression analysis by qRT-PCR. The expression levels were normalized against that of EF1α. The fold of T3 induction was obtained by dividing the value for the T3-treated animal by that of the animal of the same genotype without T3 treatment. Two asterisks (**) indicate a significant difference between homozygous knockout and the other groups (P < 0.01). (b) TRα knockout reduced TR binding to TREs in target genes even after T3 treatment. Seven-day-old tadpoles were genotyped. Five tadpoles of each genotype were pooled together and treated with 10 nM T3 for 18 hours at 25°C two days after genotyping. ChIP assay was performed on the whole animals with anti-TR or ID14 antibodies, as described in the legend of Fig. 2. Note that homozygous TRα-knockout animals had dramatically reduced TR binding at well-known target genes. Two asterisks (**) indicate a significant difference between the homozygous knockout and other groups (P < 0.01).
TRα-knockout tadpoles develop faster during premetamorphosis and initiate metamorphosis earlier
To determine the consequences of the altered expression of T3 target genes in the TRα-knockout tadpoles, we randomly chose tadpoles of the same age and similar size for genotyping and determined their developmental stage, as previously described (51). The results revealed that homozygous TRα-knockout tadpoles were at more advanced stages, as shown by one representative animal for each genotype in Fig. 4(a); the knockout animal had much more developed hind limbs. To quantify the developmental difference, we randomly selected 55 tadpoles of 11 days of age, and staged (on the basis of limb development) and genotyped each animal. Figure 4(b) shows that, of these 11-day-old tadpoles, the median stage for both the heterozygous and wild-type animals was 47.5, whereas that for the homozygous knockout animals was 51, demonstrating accelerated development of the knockout animals.
![TRα knockout accelerates premetamorphic tadpole development. (a) TRα-knockout tadpoles are at more advanced stages of development compared with wild-type or heterozygous animals of the same age and similar size. Randomly selected tadpoles were reared separately after tail clipping for genotyping. For each experiment, animals of the same age and similar size were compared after genotyping. One representative animal from one experiment is shown for each genotype. Boxes with dashed borders in the left panel were enlarged and are shown in the right panel. Homozygous TRα-knockout tadpoles always had significantly larger and more advanced hind limb buds. This analysis was repeated seven times from three batches of tadpoles. Altogether, there were 48 homozygous, 118 heterozygous, and 53 wild-type tadpoles. (b) TRα-knockout tadpoles are at more advanced stages of development compared with their age-matched wild-type and heterozygous siblings. Tadpoles in Fig. 4 (a) were staged on the basis of their limb morphology (51), and the stage of the individual animals for each genotype was plotted with the median (darker solid line) for each genotype shown in the figure. Note that homozygous TRα-knockout tadpoles reached a median stage of 51, whereas the wild-type and heterozygous TRα-knockout tadpoles reached a mediate stage of 47.5. The two asterisks (**) indicate a significant difference between homozygous knockout and the other groups (P < 0.01). (c) TRα-knockout tadpoles reach the onset of metamorphosis, i.e., stage 54, at a younger age than do heterozygous and wild-type animals. Tadpoles were genotyped at 7 days of age, and tadpoles of different genotypes were reared under identical conditions. The age in days for each tadpole to reach stage 54 was plotted, with the median (darker solid line) for each genotype shown in the figure. Note that homozygous TRα-knockout tadpoles reached stage 54 significantly sooner than wild-type and heterozygous TRα-knockout tadpoles. There was no statistically significant difference between wild-type and heterozygous TRα-knockout tadpoles. Two asterisks (**) indicate a significant difference between homozygous knockout and the other groups (P < 0.01). (d) The expression of the hind limb–specific gene Tbx4 and forelimb-specific gene Tbx5 is increased in homozygous TRα-knockout tadpoles at 15 days of age. Animals at 7 or 15 days of age [days postfertilization (DPF)] were genotyped by tail clipping, and the rest of the animal for each genotype (three animals for each genotype) were homogenized together for gene expression analysis by qRT-PCR. Two asterisks (**) indicate a significant difference between the homozygous knockout and other groups (P < 0.01).](https://oup.silverchair-cdn.com/oup/backfile/Content_public/Journal/endo/158/6/10.1210_en.2016-1953/3/m_en.2016-1953f4.jpeg?Expires=1748024296&Signature=G4jBbJuBDdm5iKR4ofJIPbPtdmFKH5MavsJxNhuVICk2Q6irG~TE3FGIJfYVoMmyQfTxYlGVZbIOVAs5zSEUd~j5EWWBNokAGQas87Cec0UwtAXhAcfwHf220GxUbTHOZ9otopGO0m3ODBeZW2zhAI35nLjpnXotj1RU~-p9g7MSz4a3jnBMH4JZbO5mOkxnFcd9temtRfhBKxh-lcECkQkDt0qFARpo9xlJ09aFLQbwgS-pLJ2Zn2Gghmvn-x1074Tn88YuTU0hGgv0d2nxijiBfFTXqTvGPueBMecZcmMwbsKxFwYCH944n0n1Y3NEXoxYSitoAQ1zekeMNk9Emw__&Key-Pair-Id=APKAIE5G5CRDK6RD3PGA)
TRα knockout accelerates premetamorphic tadpole development. (a) TRα-knockout tadpoles are at more advanced stages of development compared with wild-type or heterozygous animals of the same age and similar size. Randomly selected tadpoles were reared separately after tail clipping for genotyping. For each experiment, animals of the same age and similar size were compared after genotyping. One representative animal from one experiment is shown for each genotype. Boxes with dashed borders in the left panel were enlarged and are shown in the right panel. Homozygous TRα-knockout tadpoles always had significantly larger and more advanced hind limb buds. This analysis was repeated seven times from three batches of tadpoles. Altogether, there were 48 homozygous, 118 heterozygous, and 53 wild-type tadpoles. (b) TRα-knockout tadpoles are at more advanced stages of development compared with their age-matched wild-type and heterozygous siblings. Tadpoles in Fig. 4 (a) were staged on the basis of their limb morphology (51), and the stage of the individual animals for each genotype was plotted with the median (darker solid line) for each genotype shown in the figure. Note that homozygous TRα-knockout tadpoles reached a median stage of 51, whereas the wild-type and heterozygous TRα-knockout tadpoles reached a mediate stage of 47.5. The two asterisks (**) indicate a significant difference between homozygous knockout and the other groups (P < 0.01). (c) TRα-knockout tadpoles reach the onset of metamorphosis, i.e., stage 54, at a younger age than do heterozygous and wild-type animals. Tadpoles were genotyped at 7 days of age, and tadpoles of different genotypes were reared under identical conditions. The age in days for each tadpole to reach stage 54 was plotted, with the median (darker solid line) for each genotype shown in the figure. Note that homozygous TRα-knockout tadpoles reached stage 54 significantly sooner than wild-type and heterozygous TRα-knockout tadpoles. There was no statistically significant difference between wild-type and heterozygous TRα-knockout tadpoles. Two asterisks (**) indicate a significant difference between homozygous knockout and the other groups (P < 0.01). (d) The expression of the hind limb–specific gene Tbx4 and forelimb-specific gene Tbx5 is increased in homozygous TRα-knockout tadpoles at 15 days of age. Animals at 7 or 15 days of age [days postfertilization (DPF)] were genotyped by tail clipping, and the rest of the animal for each genotype (three animals for each genotype) were homogenized together for gene expression analysis by qRT-PCR. Two asterisks (**) indicate a significant difference between the homozygous knockout and other groups (P < 0.01).
Stage 54 is commonly considered to be the onset of metamorphosis, when hind limb morphogenesis/digit formation begins (13, 51). To determine the time required for the animals of different genotypes to initiate metamorphosis, we genotyped tadpoles at 7 days of age and then reared the tadpoles of different genotypes under identical conditions. The age, in days, when each tadpole reached stage 54 was then determined for each genotype and showed that homozygous TRα-knockout tadpoles reached stage 54 in ∼20 days after fertilization, whereas the wild-type and TRα heterozygous siblings initiated metamorphosis at the age of ∼37 days, nearly twice the age of the homozygous tadpoles [Fig. 4(c)].
Because the initiation of metamorphosis is characterized mainly by the morphogenesis of the limbs, we examined the expression of the hind limb–specific gene Tbx4 and forelimb–specific gene Tbx5 by qRT-PCR analysis of RNA from whole tadpoles at 7 or 15 days of age. In agreement with the accelerated development of the homozygous TRα-knockout tadpoles, we observed that both genes were expressed at significantly higher levels in the homozygous TRα-knockout tadpoles compared with the wild-type and heterozygous siblings [Fig. 4(d)]. Thus, TRα regulates the rate of premetamorphic tadpole development, consequently controlling the timing of metamorphosis initiation.
Removing TRα increases growth rate of premetamorphic tadpoles by upregulating growth hormone gene expression
During our analysis of the developmental stages of the age-matched animals, we noticed that the homozygous TRα-knockout animals appeared to be bigger than the wild-type and heterozygous siblings. To quantify the potential difference, we grouped the 11-day-old tadpoles in Fig. 4(b) into three categories (large, medium, and small) on the basis of their body size [Fig. 5(a–b)]. The results showed that most of the homozygous knockout animals were in the large category, whereas the vast majority of the heterozygous and wild-type animals were in the medium or small category. Given that growth hormone (GH) plays a critical role in regulating growth, and the fact that the mammalian GH gene is induced by T3 (41, 62), we analyzed the expression of the two GH genes in the tadpoles and observed that both were significantly upregulated in the homozygous TRα-knockout tadpoles [Fig. 5(c)], suggesting that unliganded TRα represses GH expression in premetamorphic tadpoles, and its removal leads to increased GH expression.
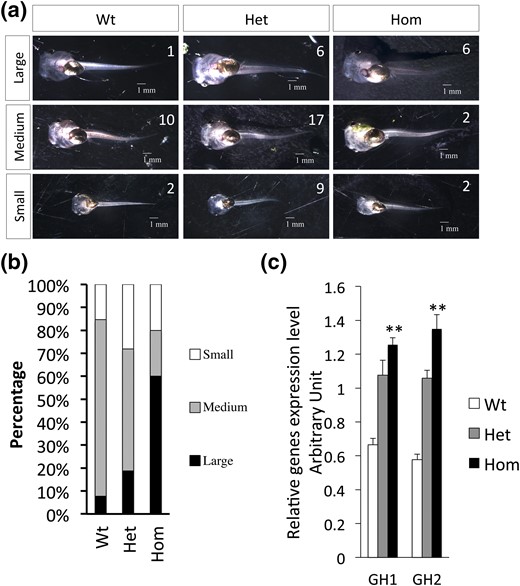
TRα knockout increases the growth rate and accelerates development of premetamorphic tadpoles. (a and b) TRα-knockout tadpoles grow larger than their age-matched wild-type (WT) and heterozygous (Het) siblings. Fifty-five randomly selected 11-day-old tadpoles were classified into three categories (small, medium, and large) on the basis of body size. The tadpoles were then photographed and genotyped. (a) One representative photo for each category from each genotype is shown with the number of animals in that category. (b) Bar graph showing the percentage of each category in the three genotypes. Note that most of the homozygous (Hom) knockout animals were in the large category, whereas the Het and WT animals were in the medium or small category. To group the animals, each tadpole was photographed under the same magnification, and the length of the ventral side of the body (minus tail) was measured with ImageJ software (https://imagej.net/). “Large” referred to length >4.7 mm, “small” referred to length <3.2 mm, and the remaining animals, measuring 3.2 mm to 4.7 mm, were categorized as “medium.” (c) The expression of the two GH genes is elevated in the knockout animals. Total RNA was extracted from 11-day-old tadpole samples. Gene expression was analyzed by qRT-PCR. Two asterisks (**) indicate a significant difference between Hom knockout and WT animals (P < 0.01).
To investigate whether the accelerated development was due to the increased growth rate in the homozygous TRα-knockout tadpoles, we next analyzed the size of the animals at the onset of metamorphosis (stage 54), regardless of the age of the tadpoles. Interestingly, we observed that homozygous TRα-knockout tadpoles at stage 54 were much smaller than the wild-type and heterozygous siblings (Fig. 6), and the latter two groups reached stage 54 at much older ages [Fig. 4(c)]. Thus, TRα appears to have two independent functions in premetamorphic tadpoles: controlling the timing of the initiation of metamorphosis and regulating animal growth rate. Upon knocking out TRα, the animals develop so quickly that they reach stage 54 at a much younger age and smaller size, despite their faster growth rate compared with the wild-type siblings during premetamorphosis.

The homozygous TRα-knockout tadpole (Hom) has a smaller body size at the onset of metamorphosis despite a faster growth rate during premetamorphosis. (a) A representative tadpole of each genotype at stage 54, the onset of metamorphosis. Arrows point to the hind limbs. Note that the homozygous TRα-knockout tadpole has a significantly smaller body size but similarly sized hind limbs compared with the heterozygous TRα-knockout (Het) and wild-type (WT) tadpoles at stage 54. (b) TRα-knockout tadpoles have a shorter body length. Animals were allowed to develop to stage 54 regardless of age. The tadpole body length was measured before tail clipping for genotyping. The sample included 15 wild-type, 23 heterozygous TRα-knockout, and 29 homozygous TRα-knockout tadpoles. The body length of individual animals for each genotype was plotted, with the median (darker solid line) for each genotype shown in the figure. Note the median length of the homozygous TRα-knockout tadpoles was about half of that for the heterozygous TRα-knockout and wild-type tadpoles. There was no statistically significant difference between heterozygous TRα-knockout and wild-type tadpoles. Two asterisks (**) indicate a significant difference between the homozygous knockout and other groups (P < 0.01).
TRα is required for the normal progression of natural metamorphosis
As described above, we showed that TRα knockout reduced the response of TR target genes to T3 treatment in premetamorphic tadpoles, which suggests that TRα is important for natural metamorphosis triggered by endogenous T3. To investigate this possibility, we randomly selected tadpoles at stage 54, the onset of metamorphosis, and reared them together. Individual tadpoles were genotyped when they reached stage 58, the beginning of the metamorphic climax. The time required for each animal to develop from stage 54 to stage 58 was recorded and shown in Fig. 7(a). Consistent with the reduced response to T3 treatment shown in Fig. 3, the homozygous TRα-knockout tadpoles took twice as long to reach stage 58 compared with wild-type and heterozygous siblings, indicating that TRα is required for normal progression during the initial phase of metamorphosis.
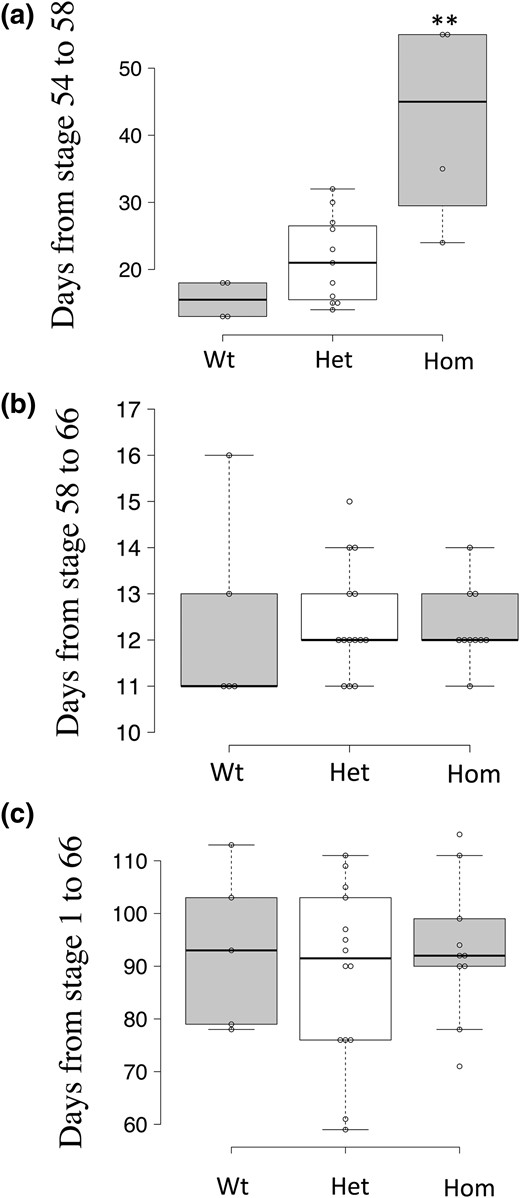
Homozygous TRα-knockout tadpoles (Hom) have a slower rate of metamorphic progression. (a) Homozygous TRα-knockout tadpoles take longer to develop from stage 54 to 58 (prometamorphosis). Stage 54 tadpoles were selected at the same time and reared together. Individual tadpoles were genotyped when they reached stage 58, the beginning of the metamorphic climax. The time required for each animal to reach stage 58 was plotted, with the median (darker solid line) for each genotype shown in the figure. The groups included four wild-type (WT), 10 heterozygous TRα-knockout (Het), and four homozygous TRα-knockout tadpoles. Note that homozygous TRα-knockout tadpoles required over twice as long to reach stage 58 compared with wild-type and heterozygous TRα-knockout tadpoles. Two asterisks (**) indicate a significant difference between the homozygous knockout and other groups (P < 0.05). (b) TRα knockout has no effect at the climax of metamorphosis. The time for each animal to develop from stage 58 to the end of metamorphosis (stage 66) was plotted, with the median (darker solid line) for each genotype shown in the figure. Note that no significant difference was observed among the three genotypes. (c) TRα knockout does not affect the overall development time from fertilization (stage 1) to the end of metamorphosis (stage 66). The time for each animal to proceed from stage 1 to the end of metamorphosis (stage 66) was plotted with the median (darker solid line) for each genotype shown in the figure. Note that no significant difference was observed among the three genotypes.
To determine if the homozygous knockout animals could complete metamorphosis, we let stage 58 animals continue to develop and observed that they were all able to complete metamorphosis, as judged by the total resorption of the tail (not shown). Surprisingly, the time required to progress from stage 58 to stage 66 (the end of metamorphosis) was similar between the homozygous TRα-knockout animals and the wild-type and heterozygous siblings [Fig. 7(b)]. Furthermore, when the age of the individual animals at stage 66 was analyzed, we observed that there were no significant differences among homozygous TRα-knockout animals and heterozygous and wild-type siblings [Fig. 7(c)]. Thus, the early onset of metamorphosis in the TRα-knockout animals was essentially offset by the slow progression of metamorphosis between stages 54 and 58.
TRα is required for proper coordination of tissue-specific metamorphosis
It is well known that metamorphosis in different organs is regulated temporally in an organ-dependent manner. The results described above showed that TRα knockout allowed the animals to initiate metamorphosis sooner, as judged by limb development. On the other hand, tail resorption was completed at similar ages for homozygous TRα-knockout animals and their heterozygous and wild-type siblings [Fig. 7(c)]. Thus, TRα knockout altered the temporal coordination of the tail and limb, two external organs with the most dramatic changes. To investigate if TRα knockout alters the temporal coordination of metamorphosis in other organs, we analyzed the intestine at the end of metamorphosis. During metamorphosis, the intestine is remodeled drastically, which involves the degeneration of larval epithelium and de novo development of the adult epithelium (63–68). One of the most dramatic changes in the intestine is the reduction in its length; the small intestine decreases in length by as much as 90% by the end of metamorphosis (63). Because homozygous TRα-knockout animals initiate metamorphosis at a younger age and smaller size, we first measured the body size of the animals at the conclusion of metamorphosis and found, not surprisingly, that the knockout animals were significantly smaller at the end of metamorphosis (stage 66) [Fig. 8(a)]. We next measured the length of the intestine for each individual animal at the end of metamorphosis (stage 66). It is well known that animals can differ in size considerably at the end of natural metamorphosis, and that larger animals generally have correspondingly larger organs. Thus, we normalized the length of the intestine against body size. The results showed that the homozygous TRα-knockout animals had significantly longer intestines compared with their wild-type and heterozygous siblings [Fig. 8(b)]. Although our analysis of the intestinal cross-section did not detect significant differences among the three genotypes, the longer length of the intestine suggests that TRα is critical for the complete metamorphosis of the intestine.
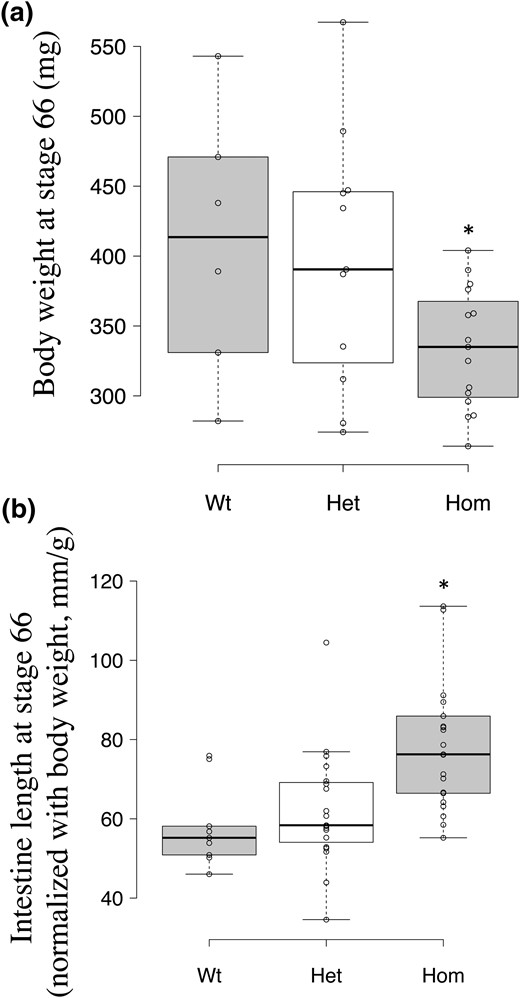
Animals lacking TRα complete metamorphosis with lower body weight but longer intestine. (a) The homozygous TRα-knockout animal (Hom) has a lower body weight at stage 66. Tadpoles were euthanized when they reached stage 66, which is considered to be the end of metamorphosis, when the tail is completely resorbed. Intestine was isolated from each animal, and both intestine and animal body were fixed in a 4% solution of MgSO4, ethylene glycol tetraacetic acid, 3-(N-morpholino)propanesulfonic acid, and formaldehyde overnight at 4°C. After washing in 70% ethanol twice, both body weight and intestinal length were measured. The body weight of each animal was plotted, with the median (darker solid line) for each genotype shown in the figure. Note that the homozygous TRα-knockout animal had significantly lower weight. The group included six wild-type (WT), 11 heterozygous TRα-knockout (Het), and 15 homozygous TRα-knockout animals. The asterisk (*) indicates a significant difference between the homozygous knockout and wild-type groups (P < 0.05). (b) homozygous TRα-knockout animals had a longer intestine at stage 66. The length of the intestine of animals described in (a) was measured from the bile duct to the large intestine. After normalization against the body weight for each animal, the relative intestine length was plotted with the median (darker solid line) for each genotype shown in the figure. Given that the length reduction is a hallmark of intestinal metamorphosis (62), the longer intestine length of the homozygous knockout animals suggests incomplete intestinal remodeling. The asterisk (*) indicates a significant difference between the homozygous knockout and wild-type groups (P < 0.05).
To investigate the underlying mechanism for the differential effect of TRα knockout on limb development, intestinal remodeling, and tail resorption, we measured the relative levels of TRα and TRβ mRNAs in the wild-type tadpoles at stage 54, the onset of metamorphosis, and stage 62 (Fig. 9), the climax of metamorphosis, when T3 level peaks (69). It is interesting to note that at stage 54, limbs had the highest levels of TRα and the highest ratio of TRα to TRβ. This may underlie the largest effect of TRα knockout on limb development. Similarly, TRα expression and the ratio of TRα to TRβ were also much higher in the intestine compared with the tail, which may be the cause of the effect of TRα knockout on intestinal remodeling. Finally, in the tail, TRα expression was very low prior to metamorphosis, and the ratio of TRα to TRβ was the lowest among the three organs, which may explain why TRα knockout has the least effect on the tail. At stage 62, TRβ expression was dramatically upregulated, especially in the intestine and tail, resulting in a much smaller ratio of TRα to TRβ expression. This may be why TRα knockout has little effect at the climax of metamorphosis, as reflected by the similar time needed for all three genotypes to progress from stage 58 to the end of metamorphosis.
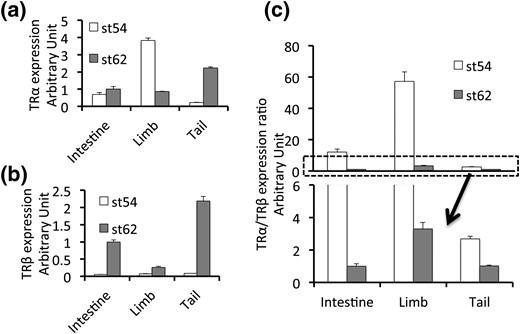
Organ-dependent expression of TRα and TRβ genes during metamorphosis. The expression of TRα and TRβ at premetamorphosis (stage 54) and climax (stage 62) was determined by real-time PCR and is shown relative to the level in the intestine at stage 62 for (a) TRα and (b) TRβ, or (c) as a ratio of the expression level of TRα to that of TRβ, with the ratio in the intestine at stage 62 set to 1. The region in the box with dashed borders in the upper panel (c) is enlarged and shown in the lower panel. Note that the limb has a much higher level of expression of TRα at stage 54 and higher levels of relative TRα/TRβ expression than the intestine, with the tail having the lowest.
Discussion
The ability of TRs to bind TREs in chromatin constitutively, but regulate gene expression in a T3-dependent manner, has long implicated a role for both liganded and unliganded TR in normal physiology and pathology. In particular, of the only two known TRs in all vertebrates, TRα and TRβ, TRα is expressed more broadly and prior to the synthesis of endogenous T3 during vertebrate development, implicating a role of unliganded TRα during development. Furthermore, T3 deficiency causes more severe effects on mouse development compared with TR knockout (36–38, 70–73), suggesting that the continued presence of unliganded TRs during development is detrimental to mouse development. However, the potential contribution of maternal T3 to the mouse embryo complicates the interpretation and makes it difficult to ascertain a role for unliganded TR in mammals.
Amphibian metamorphosis is totally dependent on T3 and takes place externally, without maternal influence. This makes it an ideal model to investigate the role of TR during vertebrate development. By using TALEN-mediated knockdown, we and the Buchholz laboratory previously showed that TRα knockdown accelerated premetamorphic development and reduced the response to exogenous T3, leading to a reduced rate of metamorphic progression (43–46). On the other hand, the lack of a well-defined, homozygous, total TRα-knockout line in these earlier studies prevented analysis of the role of TRα during natural metamorphosis and left a question about the possible role of the residual TRα in the knockdown animals. Our studies described here, with well-defined TRα–total knockout animals, have not only confirmed these earlier findings but also led to several discoveries. First, TRα knockout does not affect embryogenesis. Second, TRα is not essential for amphibian metamorphosis, but it controls the timing and rate of metamorphosis. Finally, TRα knockout has distinct effects on metamorphosis in different organs, enabling limb develop to occur earlier and disrupting the completion of intestinal metamorphosis, but having little effect on tail resorption.
Roles of TRα prior to metamorphosis
Earlier studies have shown that low levels of TRs and T3 are present during Xenopus embryogenesis (5, 34, 40, 41, 54–56) and that altering TR levels or function also affects embryonic development (16, 54). However, the role of endogenous TRs in embryogenesis was previously impossible to investigate because of the lack of TR knockout in amphibians prior to the development of gene-editing technologies. TRα is activated earlier and expressed at much higher levels than TRβ during embryogenesis (5, 34, 39–41). Interestingly, our studies have indicated that knocking out TRα has no apparent effect on embryogenesis, suggesting that either the previously reported effects of TR were due to overexpression of wild-type or mutant TR, or that endogenous TRβ was able to compensate for the loss of TRα.
On the other hand, in agreement with the high levels of TRα expression after embryogenesis and throughout premetamorphic development, TRα is critical for tadpole development. First, TRα knockout accelerated premetamorphic development, enabling the animals to initiate metamorphosis earlier. Because T3 levels prior to stage 54 are very low compared with levels during metamorphosis (55, 69), TRα likely functions as unliganded TR to repress target gene expression. Indeed, of the T3-inducible genes that we have analyzed, all were found to be derepressed upon TRα knockout, and accompanied by reduced recruitment of the corepressor NCoR. Thus, our studies provide direct support for the dual-function model for TR during frog development (12, 42). That is, in premetamorphic tadpoles, unliganded TR recruits corepressors to repress TR target genes to prevent premature metamorphosis, and when T3 becomes available, TR recruits coactivators to activate target gene transcription and initiate metamorphosis.
Unexpectedly, we also observed that TRα-knockout tadpoles grew faster compared with their age-matched wild-type and heterozygous siblings, and that this growth effect might be due to the increased expression of the GH genes. The mammalian GH gene is known to be a T3-inducible gene, and if this regulation is conserved in amphibians, high levels of TRα in premetamorphic tadpoles would repress GH expression, given that T3 levels are very low. Thus, TRα knockout would be expected to cause the derepression of GH expression, resulting in faster tadpole growth.
It is quite reasonable to expect that these two effects of TRα knockout on premetamorphic tadpoles are related to each other. One might expect that faster-growing tadpoles will reach the onset of metamorphosis sooner and thus at the onset of metamorphosis, the animals would be expected to be similar in size. Surprisingly, at the onset of metamorphosis (stage 54), homozygous TRα-knockout tadpoles were much smaller than wild-type or heterozygous siblings. It appears that despite the slower growth of the wild-type and heterozygous animals, the much slower development provided them with much longer growth time before reaching the onset of metamorphosis. This allowed them to grow into a much larger size at the onset of metamorphosis, even though they grew more slowly. Thus, TRα has two independent functions in premetamorphic tadpoles: reducing the growth rate by repression of GH expression and preventing precocious initiation of metamorphosis by repression of other T3-target genes that are critical for metamorphosis.
Roles of TRα during metamorphosis
Given the high levels of TRα mRNA but low levels of TRβ mRNA in premetamorphic tadpoles, it was not surprising that TRα-knockout tadpoles had reduced response to exogenous T3 treatment (Figs. 2–3). Interestingly, although TRα knockout led to reduced binding of TR to endogenous target genes, the remaining TR binding, especially after T3 treatment, was quite high. This appears to be a result of compensation by TRβ. TRβ is a well-known T3-induced gene (41, 58, 74–76), and thus, is repressed by unliganded TR, mainly TRα, in premetamorphic tadpoles, when there is little T3 present. TRα knockout will thus lead to derepression of TRβ (Fig. 2), which will in turn increase the binding of TRβ to TREs in TRα-knockout animals. After T3 treatment of premetamorphic tadpoles, TRβ is further activated by liganded TRβ itself, which further increases TR binding to endogenous TREs to additionally compensate for some of the loss of TR binding that results from the removal of TRα.
The reduced TR function in premetamorphic TRα-knockout tadpoles, as expected, also leads to an inhibition of natural metamorphosis: in the knockout animals, development from the onset of metamorphosis to the beginning of metamorphic climax (stage 58) takes twice as long compared with this stage of development in wild-type and heterozygous siblings, indicating that TRα regulates the rate of metamorphic progression. Interestingly, after stage 58, the rate of metamorphosis among homozygous and heterozygous TRα-knockout and wild-type animals is similar. This is likely a result of the upregulation of TRβ by the metamorphic climax when endogenous T3 levels are high, suggesting that TRβ, which is highly expressed at the climax of metamorphosis, can compensate for the loss of TRα.
When metamorphosis in different organs was analyzed, we also observed that TRα-knockout animals have distinct temporal patterns of metamorphosis in the limb, tail, and intestine compared with heterozygous and wild-type siblings. For the homozygous TRα-knockout animals, limb metamorphosis (stages 54 to 56 for limb morphogenesis, including digit formation) occurs at much younger ages, whereas tail resorption is completed at a similar age compared with wild-type or heterozygous siblings. On the other hand, intestinal remodeling, in particular, the reduction in the length of the intestine, appears to be incomplete or altered at stage 66, which is considered to be the end of metamorphosis, when tail resorption is complete. Thus, TRα is important for the temporal coordination of the metamorphosis of different organs. Furthermore, our analysis of the TRα and TRβ expression in different organs suggests that these differential effects are likely a result of the different expression levels of the receptors in these organs during metamorphosis. In addition, T3 availability in different tissues/organs during metamorphosis is also important in regulating the timing and rate of the metamorphosis of different organs/tissues. It would be interesting in the future to determine how TRα knockout affects the expression of various T3 transporters and intracellular binding proteins, and if these factors contribute to tissue-specific metamorphosis.
Conclusion
We previously proposed a dual-function model for TR during frog development (12, 42). This model has since been supported by a number of molecular and transgenic studies from different laboratories (8, 10, 16, 19–25, 28, 30, 77–86). A caveat of these studies is that transgenic overexpression may not reflect the role of endogenous factors in development.
With the development of TALEN and clustered regularly interspaced short palindromic repeat/associated protein-9 nuclease technologies for editing endogenous genes in X. laevis and X.tropicalis (31, 32), it is now possible to investigate the role of endogenous genes. By generating TRα–total knockout animals, we have now not only confirmed the findings from earlier studies involving knockdown animals but, more importantly, made a few unexpected discoveries. First, despite the high levels of TRα expression toward the end of embryogenesis, TRα is not required for embryogenesis. Second, TRα is not essential even for metamorphosis, although it is the predominant TR throughout most of Xenopus development. Last, TRα controls the temporal coordination of metamorphosis in different organs. Without TRα, the animals initiate metamorphosis at younger ages and smaller sizes but are able to complete metamorphosis, as judged by tail resorption, at similar ages as the wild-type and heterozygous siblings, but develop into smaller froglets. Our studies further suggest that TRβ can compensate at least partially for the role of TRα in the knockout animals. It thus would be of interest to generate animals lacking TRβ and/or both TRs to determine the role of TRs during Xenopus development.
Finally, it is worth pointing out that there are many similarities between frog and mammalian development. First, unliganded TR is likely present during early mammalian development, when plasma T3 levels are low. Second, several studies have implicated potential roles of unliganded TR in the development of at least some mouse organs (87–94). Third, mutations in the endogenous TR-binding corepressors, such as NCoR and SMRT in mice, which disrupt corepressor-TR interaction or deacetylase activation, suggest a role of corepressor recruitment by unliganded TR in gene regulation in vivo (95–97). It is possible that TRα may have similar roles in mammals to regulate the developmental timing and temporal coordination of different organs during mammalian early embryogenesis, when T3 levels are low, and to influence postembryonic organ maturation when T3 levels are high.
Abbreviations:
- ChIP
chromatin immunoprecipitation
- GH
growth hormone
- mRNA
messenger RNA
- qRT-PCR
quantitative real-time polymerase chain reaction
- PCR
polymerase chain reaction
- T3
thyroid hormone
- TALEN
transcription activator-like effector nucleases
- TR
thyroid receptor
- TRE
T3 response element.
Acknowledgments
This work was supported by the Intramural Research Program of the National Institute of Child Health and Human Development, National Institutes of Health.
Disclosure Summary: The authors have nothing to disclose.
References
Author notes
Address all correspondence and requests for reprints to: Yun-Bo Shi, PhD, Laboratory of Gene Regulation and Development, National Institutes of Health, 18 Library Drive, Building 18T, Room 106, MSC 5431, Bethesda, Maryland 20892. E-mail: [email protected].