-
PDF
- Split View
-
Views
-
Cite
Cite
Cecilia Ratner, Louise J. Skov, Zindy Raida, Thomas Bächler, Kathrin Bellmann-Sickert, Christelle Le Foll, Bjørn Sivertsen, Louise S. Dalbøge, Bolette Hartmann, Annette G. Beck-Sickinger, Andreas N. Madsen, Jacob Jelsing, Jens J. Holst, Thomas A. Lutz, Zane B. Andrews, Birgitte Holst, Effects of Peripheral Neurotensin on Appetite Regulation and Its Role in Gastric Bypass Surgery, Endocrinology, Volume 157, Issue 9, 1 September 2016, Pages 3482–3492, https://doi.org/10.1210/en.2016-1329
- Share Icon Share
Neurotensin (NT) is a peptide expressed in the brain and in the gastrointestinal tract. Brain NT inhibits food intake, but the effects of peripheral NT are less investigated. In this study, peripheral NT decreased food intake in both mice and rats, which was abolished by a NT antagonist. Using c-Fos immunohistochemistry, we found that peripheral NT activated brainstem and hypothalamic regions. The anorexigenic effect of NT was preserved in vagotomized mice but lasted shorter than in sham-operated mice. This in combination with a strong increase in c-Fos activation in area postrema after ip administration indicates that NT acts both through the blood circulation and the vagus. To improve the pharmacokinetics of NT, we developed a pegylated NT peptide, which presumably prolonged the half-life, and thus, the effect on feeding was extended compared with native NT. On a molecular level, the pegylated NT peptide increased proopiomelanocortin mRNA in the arcuate nucleus. We also investigated the importance of NT for the decreased food intake after gastric bypass surgery in a rat model of Roux-en-Y gastric bypass (RYGB). NT was increased in plasma and in the gastrointestinal tract in RYGB rats, and pharmacological antagonism of NT increased food intake transiently in RYGB rats. Taken together, our data suggest that NT is a metabolically active hormone, which contributes to the regulation of food intake.
Neurotensin (NT) is a 13-amino acid peptide primarily expressed in the brain and in the gastrointestinal (GI) tract. In the brain, NT is widely distributed and regulates several functions including appetite, nociception, thermoregulation, pituitary hormone secretion, and modulation of dopaminergic transmission (1). In the GI tract, NT is secreted from enteroendocrine cells primarily in the small intestine, in which it has effects on nutrient absorption and GI motility and secretion, although its physiological importance is unsettled (2).
NT inhibits food intake in rodents when injected intracerebroventricularly or directly into discrete brain regions (3–8). These effects are mainly mediated through the neurotensin receptor 1 (NTS1) because NTS1 knockout (ko) mice show increased appetite and body weight and are nonresponsive to NT's anorexigenic effects (9, 10). Contrarily, a more recent study found decreased feeding and increased activity levels in NTS1 ko mice placed on a chow diet with no overall effect on body weight and body composition (11). However, when placed on a palatable high-fat/high-sucrose diet, NTS1 ko mice ate more and gained more weight than controls and had higher sucrose preference, suggesting that the NTS1 regulates hedonic feeding behavior (11).
Whereas it is well founded that brain NT has anorexigenic potential, the metabolic effects of peripherally derived NT are less investigated. Previous studies indicate that peripherally administered NT produces a transient inhibition of food intake in rodents (7, 12, 13). In general, these studies have not considered the mechanisms causing the decreased feeding, and others report decreased feeding only when accompanied by nonphysiological side effects (4), indicating a general toxic effect. A complicating factor when working with NT is the very short half-life in the circulation in the order of 30 seconds in rodents (14). Accordingly, most of the focus has been on NT administered or expressed in various brain areas.
Recently it has been demonstrated that NT is coexpressed with the anorexigenic hormones glucagon-like peptide-1 (GLP-1) and peptide YY (PYY) in certain enteroendocrine cells (15, 16). All three hormones are increased in the blood after gastric bypass in humans (17–21), and increased numbers of enteroendocrine cells expressing NT have been observed in rats after Roux-en-Y gastric bypass (RYGB) (22). It has been suggested that these hormonal changes may contribute to the beneficial effects of bariatric surgery. Although some studies examining the causal relationship between bariatric surgery and GLP-1 and PYY exist (23–28), little effort has been made to elucidate a potential role of NT in bariatric surgery.
In this study, we investigated the anorexigenic potential of peripherally administered NT and a pegylated NT peptide. We also explored some of the mechanisms by which NT acts. Furthermore, we have examined plasma concentrations of NT and NT expression throughout the GI tract after RYGB surgery and assessed whether NT is involved in the reduced eating after RYGB using NT receptor antagonism.
Research Design and Methods
Animals
C57BL/6 mice weighing 25–30 g and Sprague Dawley rats weighing 250 g (Taconic) were used. Female mice were used for IntelliCage studies (New Behavior AG) and male mice and rats were used for the remaining studies. Animals had ad libitum access to water and a chow diet (number 1310; Altromin) unless otherwise stated. These studies were approved by the Danish Animals Inspectorate and performed according to institutional guidelines.
For immunohistochemical studies, 8-week-old C57BL/6 mice (Monash Animal Services) were housed in pairs with ad libitum access to water and a chow diet (meat free rat and mouse diet; Specialty Feeds). Immunohistochemical studies were conducted in accordance with the Monash University Animal Ethics Committee guidelines.
For RYGB studies, male Wistar rats (Janvier) were used. Rat bypass surgery experiments were performed at the University of Zurich and were approved by the Veterinary office of the Canton Zurich, Switzerland.
All animals were housed in a temperature-controlled environment under a 12-hour light, 12-hour dark cycle and were allowed a 1-week acclimatization period.
Food intake
All food intake studies were conducted under single-housed conditions in automated feeding-monitoring systems (mice studies: Phenomaster; TSE Systems; rat studies: MANI feedWin system; Ellegaard Systems A/S). Saline or NT (Polypeptide) were injected in the early-light phase after an overnight fast at a dose of 3600 nmol/kg ip in mice or 150 nmol/kg ip in rats. The NT dose used in mice was based on the most recent study in which the effect of NT on food intake was evaluated (7). The dose used in rats was optimized in our laboratory (data not shown). Rats also received the neurotensin receptor antagonist SR142948A peripherally (692 nmol/kg ip; Sigma). The SR142948A dose was based on previous publications (29, 30). NT modulated N terminally by pegylation (PEG-NT) was tested against native NT at a dose of 396 nmol/kg in mice after an overnight fast. Peptide synthesis and modification of NT with a 20-kDa pegylation was performed as previously described (31, 32), and peptide purity was greater than 95%.
Sucrose drinking in IntelliCage
IntelliCage is a system for automated recording of different behaviors, such as learning and motivation, in group-housed mice and has been described in detail previously (33, 34). Briefly, the system consists of a large polycarbonate cage, in which each corner is equipped with an operant learning chamber, consisting of two openings with access to drinking bottles equipped with lickometers. Mice are identified via an implanted transponder upon entering the chamber. Mice were habituated to the system for 5 days with water in all bottles. After this, one bottle per chamber (four per cage) was switched to a 10% sucrose solution with tap water in the remaining four bottles and mice received an ip injection of NT (3600 nmol/kg) or saline (n = 9–10) just before lights out to assess how NT affected sucrose drinking during novelty sucrose exposure. Mice had similar preference for the two sides of the chambers before sucrose was introduced. After a 4-day habituation period during which the mice had continuous access to sucrose and water, NT was administered again as above. Mice had access to chow at all times.
Conditioned taste aversion
To test whether NT decreases feeding due to malaise, we performed a conditioned taste aversion experiment. Mice were single housed for 1 week before being trained on a water deprivation schedule for 7 days during which water was available for 2 hours per day in the early light phase. One hour into the water access period, mice were given a saline injection. Two conditioning days followed the training schedule during which mice were given access to a novel flavor (0.15% saccharin) for 2 hours. After 1 hour mice received an ip injection of either saline, 3600 nmol/kg NT or LiCl (0.3 M) in a volume of 10 mL/kg. Conditioning was followed by 1 resting day during which mice were treated as during the training schedule. On the following day, mice received one bottle with water and one bottle with saccharin, and the saccharin preference ratio was calculated as the amount of saccharin intake over total intake. All fluids were presented in two bottles placed equidistant to the food.
Subdiaphragmatic vagotomy
Mice were anesthetized with sevoflurane (Baxter) and received antibiotics (5 mg/kg; Baytril; Bayer Healthcare) and analgesia (5 mg/kg, Rimadyl; Pfizer) preoperatively. Mice received an upper midline laparotomy, the liver was gently pushed cranially, and the stomach was gently pulled caudally to expose the esophagus. The ventral and dorsal branches of the vagus nerve were cauterized in two positions, starting just cranially to the stomach, and a piece of approximately 5 mm was excised. Sham animals received the same procedure without cauterizing the vagus nerve. Mice received a 15% sucrose solution and solubilized chow for 1 week after surgery and were then changed to pelleted chow and normal tap water. Mice received antibiotics and analgesia for 3 days postoperatively dosed as above.
After a 2-week recovery period, mice were placed in automated feeding cages (TSE Systems), habituated, and received NT (3600 nmol/kg) or saline ip in the early light phase after an overnight fast in a crossover design. The vagotomies were verified anatomically in all mice by tracing of retrograde labeling of fluorogold (15 mg/kg ip; Fluorochrome) to the dorsal motor nucleus of the vagus (DMX).
NT plasma concentrations
To estimate NT pharmacokinetics, mice (n = 4 per group) were injected ip with the two doses used in the present study (396 and 3600 nmol/kg), and blood samples were drawn 5, 15, and 30 minutes and 2 hours after the injection. Two in-house developed RIAs (antibody code number NTintact: 3D120; NTtotal: 3D97) were used to measure plasma concentrations of intact or total NT as previously described (35). Shortly, antibodies were produced by immunization of rabbits with either NT8–13, the biologically active part of the NT peptide, or NT1–8 for total NT because this peptide segment includes both active NT and metabolites. Assays were evaluated according to Richterich and Clinical and Laboratory Standards Institute Guidelines for Nondiagnostic Immunoassay (I/LA23-A, I/LA21-A2, and EP24-A) (36, 37). A125I-labeled NT peptide produced by stepwise oxidation (chloramine T method) was used as tracer for RIA analyses as previously described (38). The tracer was purified by high-pressure liquid chromatography (20%–25% gradient, 30 min), and the fraction with the highest immunoreactivity was used for hormone analyses. Cross-reactivity with neuromedin N was less than 1%, and the amino acid sequence of the immunogenes showed no similarity with other gut-derived peptides according to the uniprot database. The lower level of quantification was 2 pmol/L, the intraassay variation was 5%, the interassay variation was 9%, and recoveries were 100% ± 6% in perfusion buffer and 107% ± 17% in rat plasma.
c-Fos Immunohistochemistry
Mice (n = 7 per group) were fasted overnight and received NT (3600 nmol/kg) or saline in the early light phase. The effect of NT was assessed for 2 hours either during refeeding or continued fasting, after which mice were anesthetized with isoflurane and perfused through the heart with 0.05 M PBS followed by 4% paraformaldehyde (Sigma). Brains were removed and postfixed in 4% paraformaldehyde for 6 hours at 4°C followed by cryoprotection in 30% sucrose for 3 days. Brains were sectioned coronally on a cryostat in a thickness of 30 μm, collected in four series, and stored at −20°C in cryoprotectant. Brains were analyzed for c-Fos immunoreactivity (IR) in the arcuate nucleus (ARC; bregma −1.22 mm to −2.54 mm), the paraventricular nucleus (PVN; bregma −0.58 mm to −1.06 mm), the nucleus accumbens (Nac; two sections at bregma 1.18 mm and two sections at bregma 0.98 mm), bed nucleus of the stria terminalis (two sections at bregma 0.38 mm and two sections at 0.14 mm), area postrema (AP), and the nucleus of the solitary tract (NTS) (bregma −7.32 mm to −7.76 mm). c-Fos-stainings were performed as previously described (39). A Leica light microscope with a grid eye field was used to count c-Fos IR cells. All visible c-Fos-positive cells within the region of interest were counted and regions were identified using a Paxinos mouse brain atlas. Counting was performed blinded to the investigator.
Laser capture and quantitative PCR (qPCR)
Saline or PEG-NT (n = 7–8 per group) was injected ip at a dose of 396 nmol/kg, and mice were killed 4 hours later and their brains were removed and frozen in powdered dry ice. The arcuate nucleus was collected using laser capture microdissection (Zeiss PALM laser microdissection), RNA isolated, and qPCR performed as previously described (40). The following genes were analyzed: POMC forward, AGAGAGCTGCCTTTCCGCGAC, reverse, GCAGGAGGGCCAGCAACAGG; AgRP forward, GCTGCAGAAGGCAGAAGC, reverse, GACTCGTGCAGCCTTACACA; NPY forward, TGGACTGACCCTCGCTCTAT, reverse, TGTCTCAGGGCTGGATCTT, normalizing to the average value of the reference genes TBP forward, TCAAACCCAGAATTGTTCTCC, reverse, GGTAGATGTTTTCAAATGCTTCA; and Ywhaz forward, AGACGGAAGGTGCTGAGAAA, reverse, GAAGCATTGGGGATCAAGAA.
Inositol trisphosphate accumulation assay
HEK293 cells were seeded in Poly-D-lysine-coated 96-well plates (Costar; tissue culture treated) at a density of 35 000 cells per well in 100 μL growth medium (Dulbecco's modified Eagle's medium 1966 supplemented with 10% fetal bovine serum, 180 U/mL penicillin, and 45 μg/mL streptomycin) and incubated overnight at 37°C with 5% CO2 atmosphere. The cells were then transiently transfected with plasmid, pcDNA 3.1, containing the neurotensin receptor 1 (5 ng) Lipofectamine 2000 (Sigma) plus reagents. At 5 hours after transfection, 100 μL growth medium was added to the cells followed by incubation overnight. One day after transfection, human embryonic kidney-293 cells were incubated for 24 hours with 5 μCi of myo-[3H]-inositol (PerkinElmer) in growth medium. The following day, wells were washed twice in 1× Hanks' balanced salt solution buffer and incubated in 100 μL of 1× Hanks' balanced salt solution buffer supplemented with 10 mM LiCl at 37°C for 30 minutes. After stimulation with various concentrations of ligands for 45 minutes at 37°C, cells were lysed with 10 mM formic acid followed by incubation on ice for 30 minutes. YSi scintillation proximity assay beads (PerkinElmer) were diluted 1:8 with ultrapure water (final concentration of 1 mg YSi beads/well), and 80 μL of diluted beads was added to an opaque white 96-well plate. Lysates were then transferred to each well and incubated for 30 minutes at room temperature. The decay per minute by scintillation was evaluated at 2 minutes/well on a Topcount (Packard).
Roux-en-Y gastric bypass
Two cohorts of RYGB and sham rats were used in the present study. NT expression along the intestine was determined using qPCR in male Wistar RYGB (n = 5), sham (n = 5), and sham body weight matched to RYGB (BWM) rats (n = 5) (cohort 1). Rats were operated, treated, and qPCR performed as previously described (41). In short, rats were kept for 23 weeks after surgery before sample collection. The sham BWM group was given approximately 15 g of chow daily based on previous experience (42), which was adjusted every third day, dependent on their body weights compared with the RYGB rats. The entire small intestine and colon were sampled using stereological principles, and one set of samples was processed for RNA and analyzed for gene expression with 18s used as an internal standard (41). The primer sequences used were: NT forward, AACTTCCCCTTGTCCTGGAT, reverse, TTTTCTCATTGCCATGATCG, and 18s forward, TGTCAATCCTGTCCGTGTCC, reverse, ACGGACCAGAGCGAAAGCAT. NT expression levels were calculated as relative expression multiplied by mucosal volume (41).
Another cohort of RYGB and sham rats was used to test the effect of the NT antagonist SR142948A on feeding behavior (cohort 2). Twenty male Wistar rats were operated at a body weight of 448 ± 8 g (n = 12 RYGB and n = 8 sham). Preoperatively, rats received pain prophylaxis (2.5 mg/kg; Flunixin meglumin; Biokema SA) and prophylactic antibiotics (10 mg/kg; Baytril; Bayer Health Care), which was repeated on postoperative day 1. Rats were operated as previously described with minor modifications (43). Briefly, the jejunum was transected 10 cm distal to the pylorus and the proximal end, the biliopancreatic limb, was anastomosed to the ileum 25–30 cm orally from the cecum, creating the common channel distal to the anastomosis. The stomach was transected approximately 5 mm below the gastroesophageal junction, creating a gastric pouch of no more than 2%–3% of original stomach size. The distal end of the jejunum was connected to the gastric pouch, creating the alimentary limb. Sham rats had an anterior gastrostomy and a jejunostomy performed. All rats received chow before and after surgery (Provimi Kliba). The chow diet was solubilized during the first week after surgery before rats were switched back to pelleted chow. Rats were kept for 5 weeks after surgery during which body weight was recorded, and rats were then placed in automated feeding cages (BioDAQ; Research Diets) and habituated for 1 week before experiments were started. Saline or the NT antagonist SR142948A (692 nmol/kg) was injected in a balanced crossover design to RYGB and sham rats in the early light phase after an overnight fast.
During habituation to the BioDaQ system (Research Diets), a blood sample was drawn from the tail vein to ensure that NT was up-regulated before experiments were started. Total NT was measured as described above and GLP-1 levels were measured using the total GLP-1 kit (MesoScale Discovery).
Statistics
GraphPad prism version 6.0a was used for all statistical analyses. Two-tailed unpaired t tests, one-way ANOVA with Tukey post hoc test, two-way ANOVA with Sidak post hoc test, or Bonferroni-corrected paired t test as post hoc test, two-way ANOVA repeated measures with Sidak post hoc test was used as indicated in figure legends. A value of P < .05 was considered significant. Error bars represent SEM.
Results
Food intake and sucrose drinking
In accordance with previously published works (7, 12, 13), we observed a robust inhibition of food intake (chow) in mice using 3600 nmol/kg NT 2 hours (P = .0002) and 4 hours (P = .0006) after ip injections (Figure 1A). In addition to the previous observations, we found that NT also affected the intake of palatable substances when we assessed the time spent drinking from a 10% sucrose solution. NT decreased sucrose drinking time in free-fed mice both during novelty exposure (P = .04) and after habituation to sucrose (P = .04) without significantly affecting water intake (Figure 1B). In rats NT induced a transient (30 min) but significant inhibition of food intake compared with saline-treated rats (P = .0002; Figure 1C). The short duration of NT in rats is likely due to the low dose used (150 nmol/kg) because NT was not tolerated at larger doses, as previously described (4). To exclude nonspecific toxicity as causative to the anorexic effect of NT, a selective NT receptor antagonist, SR142948A, was coadministered peripherally with NT. When NT and the antagonist were coadministered, the antagonist abolished NT's inhibition of food intake (P = .0008; NT vs NT+antagonist), whereas the antagonist did not affect food intake on its own (Figure 1C). No significant differences in activity levels, resting behavior, or latency to eat were observed between groups (data not shown), supporting a specific receptor mediated anorexic effect. To examine whether NT inhibits feeding due to taste aversion, the high dose of NT (3600 nmol/kg) was tested in a conditioned taste aversion paradigm. The positive control LiCl decreased saccharin intake due to the development of taste aversion, whereas NT treatment did not significantly lower saccharin intake compared with saline treated mice (Figure 1D).
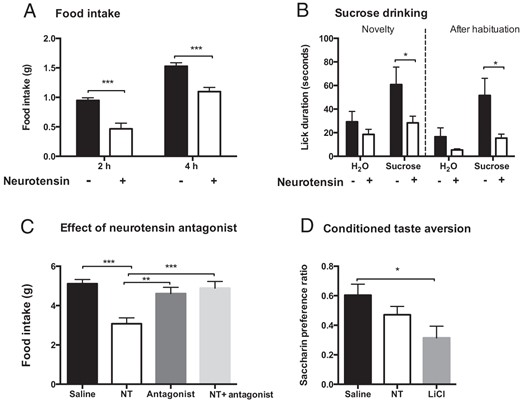
Effect of NT on feeding and sucrose drinking. NT (3600 nmol/kg) inhibited food intake in mice (A) and drinking of a 10% sucrose solution both during novelty exposure and after habituation to sucrose (B), NT (150 nmol/kg) inhibited feeding transiently in rats, which was abolished using the NT antagonist SR142948A (C), and NT (3600 nmol/kg) did not cause taste aversion in mice (D) (n = 8–12). Data were tested with a two-way ANOVA with Sidak post hoc test (A and B) or one-way ANOVA with Tukey post hoc test (C and D). *, P < .05; **, P < .01; ***, P < .001. Error bars represent SEM.
Neurotensin plasma exposure
An in-house-developed assay was used to assess intact and total NT levels in mice after injection of the doses used in the study (3600 and 396 nmol/kg). NT was rapidly degraded as previously described (14), with intact NT peaking 5 minutes after injection, the earliest time point assessed (Figure 2A). Intact NT was back to baseline levels at 15 minutes for the low dose and at 30 minutes for the high dose (Figure 2A). Total NT peaked after 5 or 15 minutes, respectively, for the low or high dose and almost reached baseline levels after 2 hours (Figure 2B).
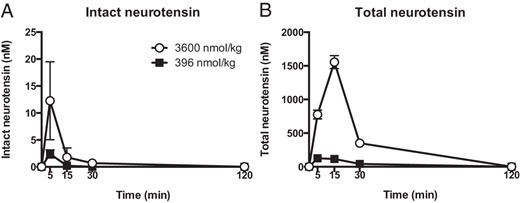
Pharmacokinetics of NT. Intact (A) and total NT (B) in plasma after ip administration of high (3600 nmol/kg) or low (396 nmol/kg) doses of NT in mice (n = 4). Error bars represent SEM.
Subdiaphragmatic vagotomy
Due to the rapid degradation of NT, we examined whether NT acts through the vagus nerve because hormones may act at higher concentrations locally around vagal afferent terminals close to their release site. Thirty minutes after NT injections, both sham-operated and vagotomized mice ate less than saline-treated mice (sham: P = .0004, vagotomized: P = .005) (Figure 3A). Two hours after NT treatment, only sham-operated mice had lower food intake compared with saline-treated mice (sham: P = .035, vagotomy: P = .40) (Figure 3A). No vagotomized mice had fluorogold labeling in the DMX (Figure 3B).
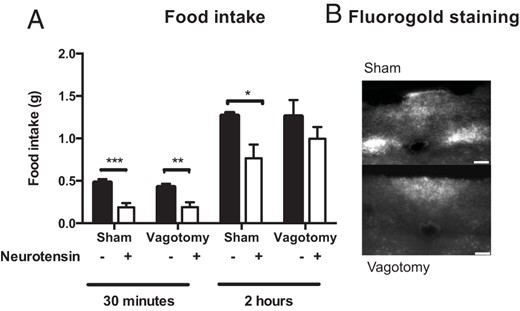
Subdiaphragmatic vagotomy. A, NT (3600 nmol/kg) inhibited feeding both in vagotomized and sham mice but the inhibition lasted longer in sham mice. B, Representative pictures of fluorogold labeling in the DMX (n = 6–7). Scale bars, 100 μM. Data were tested with a two-way ANOVA with Sidak post hoc test (A). *, P < .05; **, P < .01; ***, P < .001. Error bars represent SEM.
Immunohistochemistry
Because the vagotomy study indicated that NT can act directly in the brain through the circulation, c-Fos IR was used to identify brain regions activated by peripheral NT either during 2 hours of fasting or 2 hours of refeeding after an overnight fast. During continuous fasting, NT increased c-Fos expression in the AP (P = .024), NTS (P = .004), and PVN (P = .020), and we observed a trend toward increased c-Fos expression in the ARC (P = .050) (Figure 4, A–C). If mice were refed after NT injections, we observed a similar up-regulation of c-Fos expression after NT administration as during continuous fasting (AP [P = .0005], ARC [P = .049], PVN [P = .0061]), except in the NTS, in which no difference between saline and NT was observed (Figure 4, D–F). NT did not affect c-Fos IR in the reward regions Nac and bed nucleus of the stria terminalis (data not shown). Thus, NT activated brain regions involved in homeostatic rather than hedonic aspects of feeding behavior.
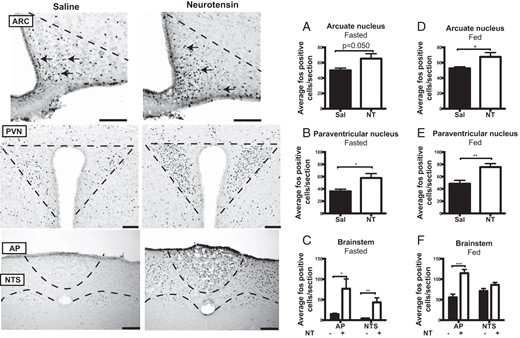
c-Fos immunohistochemistry. NT-induced c-Fos-IR after an overnight fast during 2 hours of continuous fasting (A–C) or during 2 hours of refeeding (D–F) in the ARC, PVN, and brainstem (n = 7). Scale bars, 100 μM. Data were tested with unpaired, two-tailed t tests. *, P < .05; **, P < .01; ***, P < .001. Error bars represent SEM. sal, saline.
Pegylated neurotensin
To extend the half-life of NT and to prevent penetration through the blood-brain barrier, we developed an N-terminally pegylated NT peptide. Pegylation of NT decreased the potency compared with native NT approximately 26-fold but did not affect the efficacy in an in vitro inositol trisphosphate accumulation assay (Figure 5A). Despite this, PEG-NT prolonged the in vivo decrease in food intake because PEG-NT decreased food intake to the same extent as native NT during early time points (1 h; P = .001, saline vs NT, P = .003, saline vs PEG-NT) but suppressed food intake up to 10 hours (P < .0001, saline and NT vs PEG-NT), whereas native NT suppressed food intake only during the first hour at the low dose used in this study (396 nmol/kg) (Figure 5B). Using laser capture microdissection, we found that PEG-NT increased the expression of proopiomelanocortin (POMC) in the arcuate nucleus, whereas the expression of neuropeptide Y and agouti gene-related peptide was unaltered when assessed 4 hours after injections (Figure 5C).
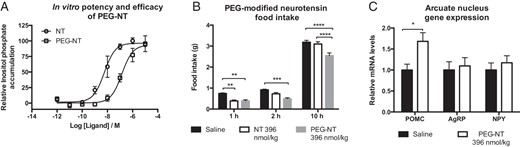
Pegylated NT. A, PEG-NT had a lower potency (26-fold) compared with native NT but had similar efficacy in an in vitro inositol trisphosphate accumulation assay. B, PEG-NT (396 nmol/kg) inhibited feeding for up to 10 hours, whereas native NT (396 nmol/kg) suppressed feeding only during the first hour after administration. C, PEG-NT (396 nmol/kg) increased mRNA levels of POMC 4 hours after injections (n = 3–4, A, and n = 7–8, B and C). Data were tested with a two-way ANOVA repeated measures (B) and unpaired two-tailed t test (C). *, P < .05; **, P < .01; ***, P < .001; ****, P < .0001. Error bars represent SEM.
Roux-en-Y gastric bypass surgery
NT expression along the rostrocaudal axis of the intestine was determined using qPCR. After the 23-week postoperative period, sham rats weighed significantly more than RYGB rats (617 ± 11 g vs 395 ± 19 g, P < .001), whereas no difference was observed between RYGB and BWM rats (41). The relative NT expression was significantly increased in the alimentary limb (RYGB vs sham and sham BWM, P < .0001) (Figure 6A). No differences were observed between sham and sham BWM rats (Figure 6A).
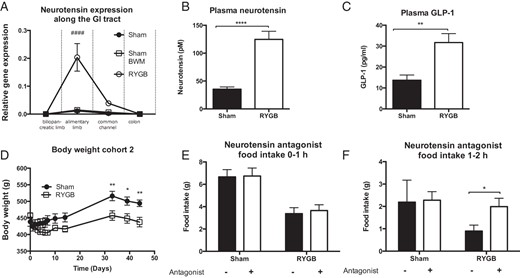
NT in a rat model of RYGB. A, NT expression was increased in the alimentary limb in RYGB compared with sham and sham BWM rats 23 weeks after surgery (cohort 1). B, Plasma levels of total NT in free-fed rats were increased in RYGB compared with sham rats 5 weeks after surgery before commencement of antagonist studies (cohort 2). C, GLP-1 levels in free-fed rats were increased in plasma in RYGB compared with sham rats at termination (cohort 2). D, Sham-operated rats had significantly higher body weight than RYGB rats (cohort 2). E and F, The NT antagonist SR142948A increased food intake transiently during the second hour of refeeding (cohort 2) (n = 5, A, and n = 8–10, C–F). Data were tested with a two-way ANOVA repeated measurements with Tukey post hoc test (A), unpaired, two-tailed t tests (B and C), a two-way ANOVA repeated measurements with Sidak post hoc test (D), and two-way ANOVA with Bonferroni-corrected paired t tests as post hoc tests (E and F). ####, P < .0001 (RYGB vs sham and sham BWM); *, P < .05, **, P < .01, ****, P < .0001 (RYGB vs sham). Error bars represent SEM..
The RYGB rats used for antagonist studies had significantly higher plasma NT (124.6 ± 14.5 pM for RYGB vs 35.4 ± 4.1 pM for sham) and GLP-1 (31.7 ± 2.5 pg/mL for RYGB vs 13.7 ± 2.5 pg/mL for sham) compared with sham-operated rats (Figure 6, B and C). RYGB-operated rats weighed significantly less than sham rats before NT antagonist studies were commenced (Figure 6D). After an overnight fast, the RYGB-operated rats had a lower food intake compared with sham-operated rats in the first 2 hours after refeeding (Figure 6, E and D). Interestingly, the selective NT receptor antagonist SR142948A increased the food intake to the same level as observed in sham-operated rats between the first and the second hour after injections in RYGB rats but had no effect in sham rats (P = .012; Figure 6, E and F).
Discussion
In the present study, we have shown that peripheral NT inhibits food intake in mice and rats in a NT receptor-dependent manner, shown by the effective blockade obtained with the NT antagonist SR142948A. We also showed that the vagus nerve contributes to the anorexigenic effects of NT, mainly during low concentrations of NT, which is in agreement with the increased c-Fos expression both in the brainstem and hypothalamic regions after NT administration. Furthermore, the pegylated NT peptide inhibited food intake for a longer time period compared with native NT. Pegylated NT up-regulated POMC in the ARC, suggesting that the anorexic effect of pegylated NT in the hypothalamus is mediated through this pathway. Finally, we have examined the importance of NT for gastric bypass surgery using a RYGB rat model. NT was increased in plasma from RYGB-operated rats and in the alimentary limb of the small intestine compared with sham and sham BWM rats, and the NT antagonist SR142948A induced a transient increase in food intake in RYGB rats, not observed in sham-operated rats. These data suggest that NT may contribute to the changes in appetite occurring after gastric bypass surgery (RYGB) and highlight the importance of peripherally administered NT in modulating appetite.
Previous studies, mainly conducted in the 1980s and early 1990s have demonstrated the anorexigenic potential of peripherally administered NT (4, 7, 12, 13). However, the studies have been inconsistent and the receptor-dependent mechanism and mode of action were not addressed. Furthermore, induction of aversion, as previously observed for other GI tract hormones such as GLP-1 and PYY, may under certain circumstances be responsible for the inhibited appetite (44, 45). Thus, we wanted to establish whether this was also the case for NT. In mice, LiCl produced taste aversion to saccharin, which was not seen after peripheral administration of the high dose of NT, suggesting a specific appetite-modulative effect of NT. Although not significant, NT did lower the saccharin preference ratio compared with saline-treated mice, and thus, we cannot exclude that aversion may contribute to NT induced satiety.
To investigate whether the vagus nerve is important for peripheral NT-induced reductions in feeding, we performed bilateral subdiaphragmatic vagotomies in mice. The vagus nerve constitutes an important link between the GI tract and the brain, and the afferent neurons often express hormone receptors including NT receptors (46). In addition, the GI tract hormones reach higher concentrations locally around vagal afferent terminals compared with plasma. This may be an important pathway in their mode of action due to the rapid degradation of these hormones, in particular for NT, which in rodents has an apparent half-life of around half a minute (14). When NT was administered to vagotomized mice, we observed a similar inhibition of food intake after 30 minutes in sham and vagotomized mice. However, after 2 hours, NT inhibited food intake only in sham-operated mice, indicating that the vagus nerve may be important when the plasma concentration of NT is low. This suggests that the elevated plasma concentration of NT secreted from enteroendocrine cells postprandially and after gastric bypass surgery (19, 21, 47, 48) may act directly on the appetite-regulating centers of the brain. The NT doses used in the present study are, however, pharmacological and give rise to supraphysiological NT concentrations in the blood at early time points after administration as evident from Figure 2. It is likely that the vagus nerve plays a more important role in mediating the effects of endogenous NT in which the plasma concentration is lower.
To further investigate the hypothesis that peripherally administered NT can activate the brain directly, we performed immunohistochemistry for the immediate early gene c-Fos and found increased neuronal activation in brainstem and hypothalamic regions after NT injections. c-Fos activation was increased in the NTS, which is the primary site for vagal afferent projections, supporting that NT acts through the vagus nerve. Contrarily, neuronal activation in the NTS could also be mediated through the AP. NT binding sites are present in the brainstem (49) and AP neurons are responsive to NT stimulation (50). Thus, the brainstem is likely to be an important target for NT-mediated feeding inhibition.
Receptors for neurotensin (NTS1) are also present in ARC (51) and NT stimulates ARC neuronal firing (52). Importantly, we observed increased POMC expression in ARC after treatment with PEG-NT, suggesting that these anorexigenic neurons are important for the decreased food intake observed after NT administration. The PVN is extensively innervated by POMC neurons, and it is an important effector region with control over pituitary hormone secretion as well as descending control over the autonomous nervous system. Thus, the increased PVN c-Fos could be induced through POMC projections from ARC, which, in turn, would result in decreased food intake. Although brain NT is closely associated with the dopaminergic system (53) and NT inhibits feeding when injected into the ventral tegmental area and substantia nigra (5, 6), peripheral NT did not affect c-Fos IR in the Nac. This indicates that peripheral NT inhibits feeding through brainstem and hypothalamic areas rather than brain regions regulating hedonic feeding.
Bariatric surgery is the only obesity intervention that currently gives rise to a large and sustained weight loss (54). The mechanisms mediating the beneficial effects of gastric bypass are incompletely understood, but altered signaling via the gut-brain axis has received considerable attention (55, 56). Several anorexigenic hormones including GLP-1 are increased in the circulation after RYGB (56). NT is likewise increased in humans after gastric bypass (19–21), and more enteroendocrine cells expressing NT in the GI tract has been described in a rat model of RYGB (22). In the present study, we found that NT was increased in the circulation and its expression was increased in the alimentary limb in RYGB rats. Using the NT antagonist SR142948A, we investigated the effect of blocking NT signaling on eating behavior in RYGB rats. When the RYGB and sham-operated rats were refed in the early light phase after an overnight fast, we observed an SR142948A-induced increase in food intake in RYGB rats, which was not seen in sham-operated rats. However, the effect of NT antagonism was transient, which is similar to results obtained using GLP-1 antagonism in RYGB rats (23). In general, in studies using transgenic mice or studies involving pharmacological manipulation of single hormones in rodents with respect to appetite and weight loss in gastric bypass, no or small effects have been described (23–28). This suggests that the effects of RYGB are unlikely to be mediated by a single hormone but rather that a combination of enteroendocrine and other factors may be responsible. In mice subjected to sleeve gastrectomy, the intracellular bile acid receptor, farnesoid X receptor, may be important for the weight loss associated with the operation (57). Interestingly, bile acids strongly stimulate NT secretion, even in low concentrations (58).
In conclusion, the present study showed that NT reduced feeding and sucrose intake and that these effects involved activation of the anorexigenic POMC neurons in ARC, which regulates homeostatic feeding behavior. NT was increased in the circulation and in the GI tract after RYGB, and NT antagonism resulted in a transient increase in food intake in RYGB rats. Thus, NT may contribute to the reduced feeding after RYGB. Taken together, our study showed that peripheral NT should be included among the appetite-regulating gut hormones, and that stable NT formulations such as PEG-NT may be useful antiobesity agents.
Appendix
Peptide/Protein Target . | Antigen Sequence (if Known) . | Name of Antibody . | Manufacturer, Catalog Number, and/or Name of Individual Providing the Antibody . | Species Raised (Monoclonal or Polyclonal) . | Dilution Used . |
---|---|---|---|---|---|
c-Fos | sc-52 | sc-52, Santa Cruz Biotechnology, Inc | Rabbit | 1:5000 |
Peptide/Protein Target . | Antigen Sequence (if Known) . | Name of Antibody . | Manufacturer, Catalog Number, and/or Name of Individual Providing the Antibody . | Species Raised (Monoclonal or Polyclonal) . | Dilution Used . |
---|---|---|---|---|---|
c-Fos | sc-52 | sc-52, Santa Cruz Biotechnology, Inc | Rabbit | 1:5000 |
Peptide/Protein Target . | Antigen Sequence (if Known) . | Name of Antibody . | Manufacturer, Catalog Number, and/or Name of Individual Providing the Antibody . | Species Raised (Monoclonal or Polyclonal) . | Dilution Used . |
---|---|---|---|---|---|
c-Fos | sc-52 | sc-52, Santa Cruz Biotechnology, Inc | Rabbit | 1:5000 |
Peptide/Protein Target . | Antigen Sequence (if Known) . | Name of Antibody . | Manufacturer, Catalog Number, and/or Name of Individual Providing the Antibody . | Species Raised (Monoclonal or Polyclonal) . | Dilution Used . |
---|---|---|---|---|---|
c-Fos | sc-52 | sc-52, Santa Cruz Biotechnology, Inc | Rabbit | 1:5000 |
For News & Views see page 3391
Acknowledgments
Christine Petersen (University of Copenhagen) and Moyra Belem Lemus (Monash University) are thanked for technical assistance. The Core Facility for Integrated Microscopy, Faculty of Health and Medical Sciences, University of Copenhagen, is thanked for access to and instruction in using the laser capture microdissector. We also thank our funding sources, the Novo Nordisk Foundation Center for Basic Metabolic Research and the German Science Foundation.
This work was supported by the Novo Nordisk Foundation Center for Basic Metabolic Research and the German Science Foundation (DFG), Grant SFB 1052/C2.
Disclosure Summary: The authors have nothing to disclose.
Abbreviations
- AP
area postrema
- ARC
arcuate nucleus
- BWM
body weight matched to RYGB
- DMX
dorsal motor nucleus of the vagus
- GI
gastrointestinal
- GLP-1
glucagon-like peptide-1
- IR
immunoreactivity
- ko
knockout
- Nac
nucleus accumbens
- NT
neurotensin
- NTS
nucleus of the solitary tract
- NTS1
NT receptor 1
- POMC
proopiomelanocortin
- PVN
paraventricular nucleus
- PYY
peptide YY
- qPCR
quantitative PCR
- RYGB
Roux-en-Y gastric bypass.