-
PDF
- Split View
-
Views
-
Cite
Cite
Kostja Renko, Carolin S. Hoefig, Corinne Dupuy, Lisbeth Harder, Christian Schwiebert, Josef Köhrle, Lutz Schomburg, A Nonradioactive DEHAL Assay for Testing Substrates, Inhibitors, and Monitoring Endogenous Activity, Endocrinology, Volume 157, Issue 12, 1 December 2016, Pages 4516–4525, https://doi.org/10.1210/en.2016-1549
- Share Icon Share
Iodotyrosine deiodinase (DEHAL1) is a crucial enzyme in iodine homeostasis. Unbound mono- and diiodotyrosines are indispensable byproducts of thyroid hormone biosynthesis. Their iodine needs to be recovered to avoid iodine deficiency, as observed in genetic defects in DEHAL1. Despite its importance, the enzyme is rarely studied. The deiodination process can be monitored by radioactive tracers or via techniques involving mass spectrometry. However, isotope-labeled molecules are expensive, not always commercially available, and their use is legally restricted, whereas mass spectrometry requires sophisticated, costly, and sensitive instrumentation. To circumvent these difficulties, we adapted the nonradioactive iodothyronine deiodinase assay to determine DEHAL1 activity by a colorimetric readout, based on the Sandell-Kolthoff reaction. DEHAL1 was recombinantly expressed and used to optimize the assay in microtiter format. We applied the setup to scenarios of alternative substrate screening or search for compounds potentially acting as endocrine disrupting compounds, without identifying novel readily accepted substrates or inhibitors yet. Next, the assay was adapted to ex vivo material, and activity was reliably determined from rodent kidney and other tissues. Analyzing two mouse models of hyperthyroidism, we observed a decreased renal Dehal1 activity and mRNA expression. Our results show that this nonradioactive DEHAL1 assay is suited to screen for potential endocrine disrupters and to monitor endogenous Dehal1 expression. We harmonized the assay protocols to enable iodothyronine deiodinase and DEHAL1 activity measurements from the same samples. Hereby, a more complete view on iodine metabolism by these predominant deiodinating activities can be obtained from a given sample by a similar process flow.
Iodotyrosine deiodinase (DEHAL1 or IYD) is an important enzyme in iodine homeostasis and thyroid function. It liberates iodine from its known substrates monoiodotyrosine (MIT) or diiodotyrosine (DIT), which are precursors and halogenated byproducts of thyroid hormone (TH) biosynthesis (1). Because iodide is an essential trace element for mammals, its loss through the excretion of iodinated byproducts needs to be avoided (2–4). The importance of this step is demonstrated by the consequences of a genetic DEHAL1 malfunction. Patients with DEHAL1 mutations have an elevated loss of iodine through the urine in the form of MIT and DIT and may suffer from hypothyroidism, compressive goiter and mental retardation, summarized as iodotyrosine deiodinase deficiency (OMIM entry *612025) (5, 6). Positive effects of iodide supplementation on thyroidal iodide uptake, serum T4 levels and goiter size have been observed in young patients (7). Besides in genetic iodotyrosine deiodinase deficiency, elevated levels of DIT in serum are also found in inflammation and infection (8). In chronic renal disease, elevated DIT is detected in the urine (9).
In the newborn screening program for congenital hypothyroidism, DEHAL1 mutations escape detection as most patients are initially without pathological findings but may develop serious consequences later in life (5). The affected individuals in the few families identified and molecularly characterized so far were homozygous for missense or frame shift mutations, dramatically reducing DEHAL1 enzymatic activity. A moderately reduced activity, eg, due to genotype, heterozygous mutations, or upon exposure to thyroid disrupting chemicals, reduces the capacity of iodine recycling and may cause clinical signs, depending on the residual activity and iodine supply.
Surprisingly, the number of experimental or clinical studies on this enzyme is very limited, likely due to a lack of suitable detection methods. Although the first description of its activity was published decades ago (10), almost in parallel to the identification of the iodothyronine deiodinases (DIOs), DEHAL1 was not extensively studied until the beginning of the 21st century. The identification of the human gene by Serial Analysis of Gene Expression and first data on its structure and tissue-specific expression were reported in 2003 (11). A detailed study on its cellular localization, expression pattern on protein level, and activity of the recombinant enzyme was published one year later (12). Collectively, DEHAL1 turned out to be a member of the NADH oxidase/flavin reductase superfamily, mainly expressed in the thyroid gland (apical membrane of the thyrocytes) and, to a lesser extent, in kidney, trachea, and liver. DEHAL1 is present in different isoforms (DEHAL1, DEHAL1B, and DEHAL1C), from which only DEHAL1 turned out to be an enzymatically active protein (13).
In thyroid tissue, DEHAL1 is up-regulated by TSH-dependent cAMP generation, and it decreases in response to acute and chronic iodide load (14). Analyzing human thyroid specimens from different pathologies, DEHAL1 protein expression was elevated in toxic thyroid nodules and Graves’ disease, which is consistent with an increased cAMP-protein kinase A signaling in thyrocytes and a generally elevated turnover of TH in these diseases. In contrast, DEHAL1 expression was found to be down-regulated in thyroid cancer (15).
Sun et al recently studied the influence of iodide supplementation on Dehal1 expression in rats. In this animal model, a tissue-specific regulation in thyroid was shown, based on qPCR and Western blot analyses (16). Notably, iodide deficiency increased and excessive iodide intake decreased Dehal1 expression on the mRNA or protein level in the thyroid gland, with only marginal effects on its mRNA and protein expression in liver or kidney.
The enzymatic Dehal1 activity is rarely monitored, even though analytical methods have been developed. In the classical approach, radioactively labeled DIT and/or MIT is used (10), and enzymatic activity is calculated from the quantification of the liberated iodide via radiometric analysis after separation of the tracer molecules by cation exchange chromatography. This technique was used to study thyroid Dehal1 regulation at the activity level in rats (14). Because there are, to our knowledge, no commercial sources for the radiolabeled MIT or DIT tracers, the assay is difficult to be set up and includes an isotopic exchange labeling of “cold” DIT, as well as cumbersome purification and calibration steps. An alternative method for quantifying MIT and DIT uses liquid or gas chromatography-mass spectrometry (MS) (17), both of which require intensive preanalytical sample preparation, expensive equipment, and high technical expertise.
Theoretically, the reaction can also be followed by a coupled enzymatic activity assay using simple photometry, because DEHAL1 uses NADPH as cosubstrate. Unfortunately, specificity is difficult to achieve, as many other enzymes use the same cosubstrate and may interfere with the measurements. Therefore, an extensive cleaning and purification of the DEHAL1 protein fraction before analysis would be required (18), making this method inconvenient for ex vivo samples and difficult to scale up to high sample numbers for screening purposes.
Recently, we developed a nonradioactive method to determine DIO activity, which was based on the Sandell-Kolthoff reaction to quantify the amount of iodide released by the enzymatic activities (19, 20). We succeeded in setting up a method in microtiter plate format as a screening assay for endocrine-disruptive compounds (EDCs) by using recombinant sources of DIO1, DIO2, or DIO3. Furthermore, the method proved suitable to measure endogenous activity from murine liver and kidney, as well as from human cell culture samples (eg, HepG2 hepatoma cells).
Here, we describe the successful adaptation of this assay principle to quantify recombinant and endogenous DEHAL1 activity, resulting in a simple, easily accessible, and time- and cost-effective method to determine Dehal1-dependent iodide release in screening approaches, for testing alternative substrates, potential inhibitors (21), and for analyzing ex vivo samples from animal experiments.
Materials and Methods
Materials
Chemicals were of analytical grade and obtained from Merck or Sigma-Aldrich. Iodothyronines were purchased from Sigma-Aldrich and Formula GmbH. Dibromotyrosine (DBT), MIT, and DIT were from Tokyo Chemical Industry. Propylthiouracil and flavin adenine dinucleotide disodium salt hydrate (FAD) were acquired from Sigma-Aldrich. NADPH tetrasodium salt was from Carl Roth GmbH. Origin, quality control and handling of the various test compounds (Table 1) and TH has been described previously (22, 23).
Abbreviation . | Compound . |
---|---|
GC1 | Synthetic Th receptor agonist |
4MBC | 4-Methylbenzyliden campher |
4NP | 4-Nonylphenol |
BP2 | Benzophenone 2 |
BP3 | Benzophenone 3 |
BPA | Bisphenol A |
GEN | Genistein |
RES | Resveratrol |
SIL | Silymarin |
NIT | Nitrofen |
DBP | Dibutylphthalate |
ACL | Acetochlor |
DMSO | Dimethylsulfoxide (control) |
H2O | ddH2O (control) |
DBT | DBT (control) |
Abbreviation . | Compound . |
---|---|
GC1 | Synthetic Th receptor agonist |
4MBC | 4-Methylbenzyliden campher |
4NP | 4-Nonylphenol |
BP2 | Benzophenone 2 |
BP3 | Benzophenone 3 |
BPA | Bisphenol A |
GEN | Genistein |
RES | Resveratrol |
SIL | Silymarin |
NIT | Nitrofen |
DBP | Dibutylphthalate |
ACL | Acetochlor |
DMSO | Dimethylsulfoxide (control) |
H2O | ddH2O (control) |
DBT | DBT (control) |
Abbreviation . | Compound . |
---|---|
GC1 | Synthetic Th receptor agonist |
4MBC | 4-Methylbenzyliden campher |
4NP | 4-Nonylphenol |
BP2 | Benzophenone 2 |
BP3 | Benzophenone 3 |
BPA | Bisphenol A |
GEN | Genistein |
RES | Resveratrol |
SIL | Silymarin |
NIT | Nitrofen |
DBP | Dibutylphthalate |
ACL | Acetochlor |
DMSO | Dimethylsulfoxide (control) |
H2O | ddH2O (control) |
DBT | DBT (control) |
Abbreviation . | Compound . |
---|---|
GC1 | Synthetic Th receptor agonist |
4MBC | 4-Methylbenzyliden campher |
4NP | 4-Nonylphenol |
BP2 | Benzophenone 2 |
BP3 | Benzophenone 3 |
BPA | Bisphenol A |
GEN | Genistein |
RES | Resveratrol |
SIL | Silymarin |
NIT | Nitrofen |
DBP | Dibutylphthalate |
ACL | Acetochlor |
DMSO | Dimethylsulfoxide (control) |
H2O | ddH2O (control) |
DBT | DBT (control) |
Animal experiments
C57BL/6J male mice at the age of 3–4 months were housed in single cages at 21°C–22°C on a 12-hour light, 12-hour dark cycle and had ad libitum access to food and water. Animal care procedures were in accordance with the guidelines set by the European Community Council Directives (86/609/EEC) and approved by Stockholm’s Norra Djurförsöksetiska Nämnd Animal Care Committee. The tissue samples used in this study were derived from the mice experiments described in recent articles of Hoefig et al (24, 25).
Oral replacement of T4 in hypothyroid mice
For oral T4 replacement studies, mice were pretreated for 2 weeks and subsequently maintained on 0.1% MMI/0.2% sodium perchlorate in drinking water for the duration of hormone replacement over the period of another 2 weeks (24). T4 was dissolved in 20mM NaOH and diluted in the MMI and NaClO4-containing water (12 mg/L f.c.). Animals were killed, dissected, and kidneys were frozen immediately on dry ice and stored at −80°C until used for RNA extraction and determination of Dehal1 as well as Dio1 enzyme activities. The immunological analysis of total T4 (EIA 1781; DRG Instruments GmbH) and total T3 (DNOV053; NovaTec Immundiagnostica GmbH) in serum confirmed that the mice were hypothyroid (TT4, 0.8 ± 0.11 μg/dL; TT3, 0.5 ± 0.04 ng/mL), whereas the T4-resubstituted animals were significantly hyperthyroid (TT4, 15.83 ± 1.19 μg/dL; TT3, 2.26 ± 0.01 ng/mL), as reported (24).
Mouse model for experimental thyrotoxicosis
In this experiment, animals were randomly assigned to a control (EU), T4, or T4-recovery group (n = 8). Mice were treated with T4-containing drinking water (1 mg/L in 0.1% BSA containing tap water; Sigma-Aldrich) for 14 days. Body weight, body temperature, food, and water intake were measured weekly in the T4-recovery group as reported (25). The comparison of TT4 levels in serum between control, T4, and T4-recovery animals confirmed that the treatment paradigm generated a hyperthyroid state with TT4 concentrations about 3-fold elevated and TT3 concentration 2.5-fold above control after 14 days. The elevated THs returned back to euthyroid levels (T4) or even below (T3) after another 14 days without treatment as described (25).
Expression of recombinant DEHAL1 enzyme
The expression plasmid for DEHAL1 was designed and cloned as described (12). HEK293 human embryonic kidney cells were transiently transfected with the construct containing the DEHAL1 open reading frame. Two days after transfection, cells were harvested. The cell layer was washed twice with ice-cold PBS, and cells were then detached by scraping from the culture vessels into homogenization buffer (250mM sucrose, 1mM EDTA, and 20mM HEPES; pH 7.0). Subsequently, the suspended cells were disrupted by sonication (2 × 10 pulses, 100% amplitude, 0.5 s; Labsonic M, Satorius AG), protein concentration was measured by Bradford assay (Bio-Rad), and the homogenate was frozen in aliquots at −80°C at a final concentration of 10 μg/μL.
Sample preparation for ex vivo measurements
Frozen solid tissue was homogenized on ice in homogenization buffer and membrane-bound protein was precipitated by centrifugation (14 000g, 10 min). After removal of the supernatant, the pellet was resuspended in another portion of homogenization buffer and protein concentration was determined by Bradford assay. The homogenates were adjusted to equal protein concentrations (2 and 5 μg/μL, respectively).
Nonradioactive iodine-release assay
The assay was developed during the course of this study and underwent some rounds of optimization. Below is a description of the final assay protocol; differences from this general scheme are indicated and specified in the respective figure legends, if details deviated from the given protocol. The protocol is summarized as a graphical workflow scheme in Figure 1.
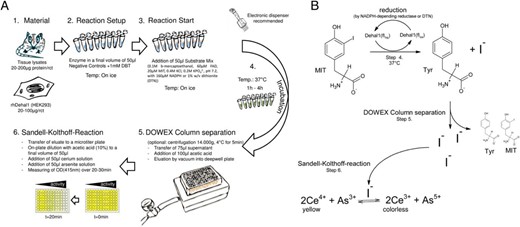
Schematic workflow diagram of the DEHAL1 assay. A, After preparing homogenous concentrations of the test samples in 50 μL (steps 1 and 2), the reaction is rapidly started by the addition of 50-μL substrate master mix (step 3) and incubated under specified conditions. After the separation of intact substrate molecules from the released iodide (step 5), the eluted iodide is transferred to relative quantification by the Sandell-Kolthoff reaction (step 6). B, Principle of coupling the enzymatic iodide release with the Sandell-Kolthoff reaction on a molecular level.
Each reaction is set up in a 0.2-mL PCR tube, to guarantee its compatibility to the microtiter format; 50–100 μg of total protein lysate (HEK293 with transient DEHAL1 expression), resuspended in 5–10 μL of homogenization buffer, are adjusted with homogenization buffer to a final volume of 40 μL. A total of 10 μL of water are added to the uninhibited samples (positive controls) and 10 μL of DBT (10mM) to the inhibited controls. To start the reaction, 50 μL of a master mix (0.1M β-mercaptoethanol, 60μM FAD, 20μM MIT, 0.4M KCl, and 0.2M KPO42−; pH 7.2), with 160μM NADPH or 1% wt/vol dithionite (DTN), are applied. The reactions are incubated at 37°C under constant shaking.
After an appropriate incubation time (1–2 h for recombinant protein, 2–4 h for tissue lysate), the samples were further processed as already described for the nonradioactive DIO assay (19, 20). In brief, the reactions were cleared by centrifugation (14 000g, 4°C, 5 min), and then 75 μL of supernatant were applied on microtiter columns prefilled with DOWEX 50 × 2 resin. After application of an additional 100 μL of acetic acid (10% vol/vol), the samples were eluted by vacuum into a deepwell plate. The eluate was further diluted with acetic acid (10% vol/vol) in a ratio of 1:2 to 1:8 to a final volume of 50 μL in a respective microtiter plate (TPP). The determination of DEHAL1-dependent iodide release was started by adding 50 μL of cerium solution (25mM (NH4)4Ce(SO4)4 and 0.5M H2SO4 in ddH2O) and 50 μL of arsenite solution (25mM NaAsO2, 0.2M NaCl, and 0.5M H2SO4 in ddH2O). Photometric measurement at 415nm was immediately started after completing the reaction mixture, and the OD was measured at time points t = 1 and t = 21 minutes, for the calculation of the time-dependent changes in absorption (dOD, 20 min). Background (BG) was defined as average dOD in samples inhibited by addition of 1mM DBT. Data are given as “dOD” or “dOD-BG,” depending on the experiment. In accordance to the DIO assay, this difference was taken as relative DEHAL1 activity.
For an estimation of the enzymatic activity, the iodide standard was diluted to the appropriate concentrations, added to the reaction mixtures without protein and processed as described before. Some of the resulting standard curves were used to determine a calculated iodide release per protein and time, corresponding to the dOD (415nm) values. The specific activity is calculated as picomole of released iodine per minute of incubation time and milligram of protein.
Gene expression analysis
RNA from tissue was extracted by the use of an RNA preparation kit (Bio-Rad), as described by the manufacturer. RNA yield was quantified via photometry (Nanodrop; Thermo Scientific). For cDNA synthesis, 1 μg of RNA was transcribed using a first-strand cDNA synthesis kit (iScript; Bio-Rad). qPCRs were conducted with the Absolute qPCR SYBR Green Fluorescein Mix (Thermo Scientific) on an iCycler system (Bio-Rad) or as described elsewhere (25).
For Dehal1 mRNA quantification, the following forward and reverse primers were used: 5′-GACCTGAAGAAACTGAGAACCA and 5′-TTTCCATTCGCAGCAAAACC, as deduced from the published sequence (NM_027391.3). For normalization purposes, hypoxanthin-guanin-phosphoribosyltransferase was chosen as housekeeper gene and its expression was determined in parallel to Dehal1 mRNA.
Statistics and software
Statistical analyses and nonlinear regression were performed using GraphPad Prism v.4.0 (GraphPad Software, Inc). The results are represented as mean ± SD. The test which was used for comparison, as well as the number of replicates is given in the figure legends. Figure 1B was created by using the freeware version of ACD/ChemSketch 2015 (Advanced Chemistry Development, Inc).
Results
Proof-of-concept experiments with recombinant DEHAL1
After transfection of HEK293 cells with a DEHAL1 expression plasmid, the resulting cell homogenate was tested for detectable deiodination by the protocol described above. Briefly, 100 μg of total cell lysate yielded an activity in the detection range (dOD ∼ 1.2) after 1 hour of incubation with 5μM MIT as substrate. The enzymatic activity of recombinant human DEHAL1 was approximately 22 pmol/mg per min, and in a protein dilution series, the expected decline in iodide release was observed (Figure 2A). In view of these results, 50–100 μg of this lysate were found to be appropriate for further screening approaches. A side-by-side comparison of MIT and DIT identified MIT as the better substrate, when presented to the enzyme at a concentration of 10μM. The Km value of the enzyme was determined with MIT as substrate and was calculated as 0.7μM (Figure 2B), which is in good accordance with the literature (12). The incubation of the enzyme under test conditions over a time period of 2 hours resulted in a constant release of iodide with a linear increase in the dOD readings, indicating a relatively high stability of DEHAL1 under these conditions (Figure 2C). The coincubation with DBT, a specific and competitive inhibitor of DEHAL1 activity (26), suppressed the iodide release below the detection limit of the assay.
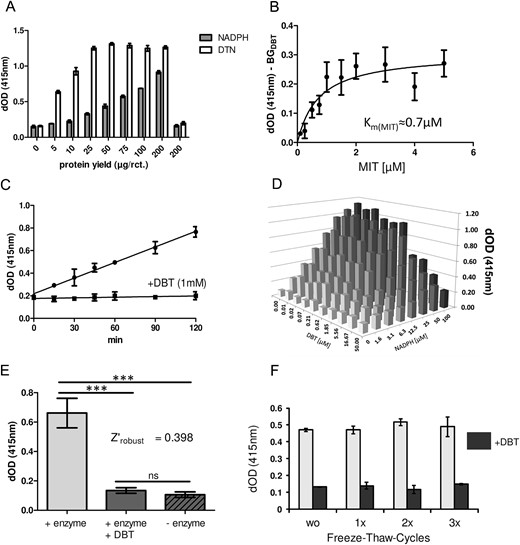
Nonradioactive detection of recombinant DEHAL1 activity. The photometric readout dOD at 415nm represents iodide release quantified by the Sandell-Kolthoff reaction. A, Iodide-release was concentration dependently related to the amount of added protein from DEHAL1-expressing HEK293 cells. A direct comparison indicates that DTN constitutes the superior reductant in comparison with NADPH with respect to the maximal velocity of iodide release (1 h of incubation, n = 4, mean ± SD). B, The Km value for MIT was determined in an experiment with various concentrations of MIT as substrate and incubation over a short time frame (1 h of incubation, 50-μg protein/reaction). C, Recombinant enzyme (50 μg/reaction, time course, n = 3, mean ± SD) was incubated with 20μM MIT with or without addition of DBT (f.c. 1mM). DBT inhibited the DEHAL1-dependent iodide release over the whole period of incubation time. The noninhibited reaction displayed an almost linear DEHAL1-dependent release of iodide from the substrate. D, Titrations of NADPH and DBT indicate that NADPH can serve as cosubstrate of the enzyme, whereas DBT inhibits DEHAL1-mediated iodide release (50 μg/reaction, 2 h of incubation, n = 1). E, Robustness of the assay was tested by comparing a high number of reactions with and without enzyme, as well as reactions with enzyme and surplus addition of DBT (f.c. 1mM). The DEHAL1-generated signal was distinguishable from BG with high significance. DBT in the given concentration was able to generate a BG signal without any significant difference from the reactions without enzyme (100-μg protein/reaction, 2 h of incubation, n = 32, mean ± SD, ANOVA with Bonferroni’s post hoc test). F, Repeated freeze-thaw cycles (without additional storage time) did not decrease enzymatic activity in murine kidney homogenate (100-μg protein/reaction, 3 h of incubation, n = 3).
A cross-titration of NADPH and DBT provided a positive correlation of NADPH concentration and DEHAL1-dependent iodide release, whereas the expected negative correlation was found for increasing DBT concentrations and DEHAL1 activity (Figure 2D).
Next, DTN was tested as an alternative reductive cosubstrate (27). In comparison with NADPH, the rate of MIT deiodination was strongly increased by the use of DTN (Figure 2A), which is in accordance with the literature (28). Maximum rate of DEHAL1-dependent deiodination was found in a range of 0.1mM–0.5mM NADPH, and in a range of 0.05%–0.1% wt/vol DTN, respectively (data not shown). Robustness of the assay was tested by conducting a large number (n = 32) of replicates. The Dehal1 activities were compared in noninhibited, DBT-inhibited, and enzyme-free reactions (Figure 2E). No significant differences between DBT-inhibited and enzyme-free samples were found, demonstrating the suitability of DBT to determine the BG control activity. Repeated freezing and thawing did not lead to a significant reduction of sample activity as tested for kidney protein and up to 3 cycles (Figure 2F).
Substrate specificity and search for inhibitors of DEHAL1 activity
To test the applicability of this new method in substrate and inhibitor screenings, we incubated the lysate containing recombinant DEHAL1 with a variety of iodine-containing molecules (16 compounds, see Figure 3 for details). Besides MIT and DIT as positive controls, we tested several TH and their derivatives, iodide-containing pharmaceuticals and also iopanoic acid, which was shown to be an alternative and isotype-unspecific substrate for DIO-dependent deiodination (19).
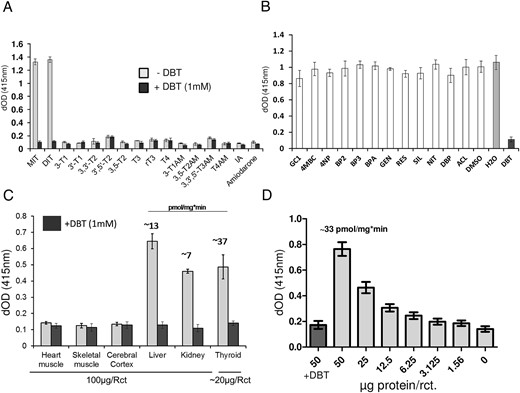
Test for alternative substrates, inhibitors, and applicability for ex vivo samples. A, A variety of TH metabolites, different thyronamines, and 2 iodine-containing drugs were incubated with recombinant DEHAL1 under standard reaction conditions (f.c. 10μM per substance, DTN as reductant), in the presence or absence of DBT as specific inhibitor. None of the tested compounds, except for the positive controls MIT and DIT, showed a DEHAL1-dependent iodide release (50-μg protein/reaction, 2 h of incubation, n = 3, mean ± SD). B, The coincubation of a number of compounds supposedly interacting with TR or other TH-binding sites was not strongly (decrease > 25%) affecting DEHAL1-mediated iodide release from MIT, except for the positive control substance DBT (50-μg protein/reaction, 2 h of incubation, DTN as cosubstrate, n = 4, mean ± SD). C, Various murine organs were compared regarding their endogenous Dehal1 activity. For heart muscle, skeletal muscle, cortex, liver, and kidney, 100 μg of protein homogenate were used to determine activity. Total thyroid glands were homogenized due to the low amount of material. An average amount of 20 μg of protein was available for the reactions. Three individual organs were analyzed for each tissue (3 h of incubation, n = 3). A detectible amount of Dehal1-dependent iodide release was found for liver, kidney and thyroid only, which is in accordance to the literature. By the use of potassium iodide standard curves, an estimation of specific activity for these 3 organs was achieved. Average activities are given in the figure above the respective bar graphs. D, Human thyroid tissue was homogenized and various amounts of resulting protein homogenates were used in the respective reactions (2 h of incubation, n = 4). For the highest protein concentration, another set of reactions was prepared including DBT (1mM) as negative control. DEHAL1-dependent iodide release declined, as expected, with decreasing amounts of protein. The addition of DBT suppressed DEHAL1 activity and BG signal in these samples was equal to control reactions without enzyme.
Although MIT and DIT were deiodinated efficiently, none of the other compounds showed a DEHAL1-dependent iodide release in the detectable range. DEHAL1 appears to have a high substrate specificity, without detectable substrate overlap with DIO-dependent deiodination (Figure 3A).
In addition, a small selection of compounds from industrial or natural sources was tested, which are known for TH receptor interaction, interference with other TH-binding sites and/or structural similarities to TH (Table 1). Again, no additional substance with inhibitory potential of DEHAL1 was identified, except of the positive control substance DBT (Figure 3B).
Endogenous regulation of Dehal1 and Dio1 activity by THs
The assay setup was tested for measuring endogenous Dehal1 activities by using a panel of murine tissue homogenates. A preselection was carried out by using data from the online protein atlas (http://www.proteinatlas.org/). Three tissues with a predicted high enzymatic activity vs three with low or no Dehal1 expression were compared. The predicted expression pattern was in agreement with the corresponding DBT-sensitive iodide release. In detail, high Dehal1 activity was found in thyroid tissue and, to a lesser extent, in kidney and liver. Neither heart muscle nor skeletal muscle and cerebral cortex did express any detectable activity (Figure 3C). A dilution series with homogenates prepared from a human thyroid tissue was analyzed and showed the expected, protein concentration-dependent decline in iodide release from the substrate in the enzymatic test (Figure 3D).
In the first animal experiment, mice were rendered hypothyroid by MMI/KClO4 treatment and were for 2 weeks additionally treated or not by oral application of T4 yielding a group of hypothyroid and a group of hyperthyroid animals. The T4-treated animals showed strongly elevated renal and hepatic Dio1 activities, as already reported (24). In parallel, Dehal1 expression and activity were significantly lower in the hyperthyroid mice, as compared with hypothyroid animals, highlighting an inverse regulation of Dio1 and Dehal1 activities in liver and kidney in hyperthyroidism (Figure 4, A and B).
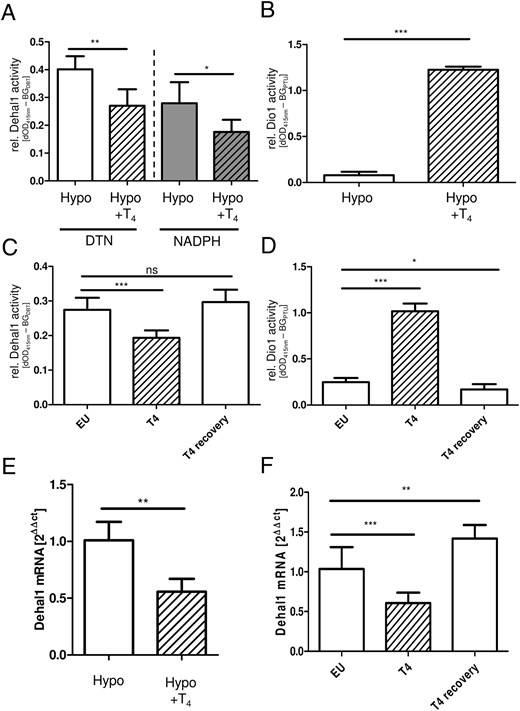
Regulation of Dehal1 and Dio1 activity and expression in hypothyroid and hyperthyroid male mice. T4 treatment of hypothyroid (Hypo) mice reduced renal Dehal1 activity and expression levels (A and E), whereas renal Dio1 activity increased under these conditions (B) (n = 4–5, mean ± SD, Student’s t test). This regulation pattern was confirmed in a second animal experiment comparing euthyroid (EU) and hyperthyroid (T4) mice. T4 treatment caused strongly reduced renal Dehal1 (C) and increased renal Dio1 (D) activities. After a washout phase, activities returned to BG levels in euthyroid animals. Changes in enzymatic activity were accompanied by respective differences in mRNA expression levels (F). C and D, n = 8, mean ± SD, ANOVA with Dunnet’s post hoc test.
In order to verify and refine these findings, a second analysis was conducted with euthyroid mice as control group in comparison with a group of T4-treated hyperthyroid mice. Again, Dehal1 expression and activities were significantly reduced in the kidneys of the hyperthyroid animals (Figure 4C), confirming the results from the first experiment. After a washout phase, and therefore upon normalization of blood T4 concentrations, also Dehal1 activity recovered to the range of control animals. In contrast, renal Dio1-dependent iodide release was again regulated inversely as compared with Dehal1 activity (Figure 4D). The results of both animal experiments are in agreement with the respective alterations of the Dio1 and Dehal1-transcript concentrations (Figure 4, E and F).
Discussion
In this study, we describe a novel nonradioactive test for DEHAL1 activity. The overall test principle is similar to the one described recently for the different DIO isozymes (20). And indeed, both deiodinating enzyme activities can be detected by a similar set up and process flow from the same sample. In order to establish the test, we used recombinant expression of DEHAL1 in HEK293 cells as a specified enzyme source. We succeeded in establishing a protocol which is suitable to identify potential modulators of DEHAL1 activity, alternative substrates, and endogenous expression levels (Figure 1, A and B).
In accordance with the literature, DIT and MIT both worked as substrates, with MIT being more readily accepted (7). Among the potential reductive cosubstrates, both NADPH and DTN were suitable compounds. Although NADPH represents the more “physiological” reductant, the use of DTN was leading to a higher maximal reaction velocity, which is of advantage in measurements close to the detection limit and furthermore allows a more cost-efficient test setup (Figure 2A). Because DTN-dependent assay follows a nonphysiological and nonlinear kinetics (29), this compound appears unsuitable to determine kinetic attributes of the enzyme. On the other hand, the use of DTN uncouples the DEHAL1 activity from the preconditioned NADPH-dependent FAD reductase activity, which might be a limiting factor depending on the sample type.
From a technical point of view, the assay allows the determination of both Dio1 and Dehal1 from the same homogenate. Because these activities were counterregulated in the murine tissue samples analyzed, the results also indicate the absence of interference between these activities within the optimized assay setup and their activity ratio might provide a more sensitive readout for the TH status of the corresponding tissue.
We concluded that this method is suitable for screening purposes with chemicals that might influence DEHAL1 activity as inhibitors or as alternative (co)substrates. DEHAL1 is considered as one crucial endpoint of the TH axis being potentially targeted by relevant EDCs (30). We conducted a respective experiment with a selection of compounds and included potential EDCs and alternative substrates. Despite the fact that DBT proved as a reliable inhibitor control, no novel hits were yet identified. Nevertheless, the experimental setup turned out to be a reliable tool for more extensive studies on this topic, as it is highly reproducible, cost effective, fast and already adopted to microtiter format. As previously described for the DIO1 assay (11, 12), one technician should be able to process approximately 500 single reactions per day. Moreover, it should be possible to adapt this assay for an automated format suitable for a high-throughput screening platform. This novel approach will thus help to identify and characterize relevant compounds of known or suspected interference with Dehal1 activity, and it allows an evaluation of specificity in comparison with DIO inhibition (18).
Because the radioactive and MS-based assays are usually characterized by high accuracy and sensitivity, we were wondering whether the novel method would also be suitable for analyzing ex vivo samples from animal experiments. Working with this type of biosamples poses some inherent challenges. Firstly, the lysate composition is more complex and inhomogenous regarding other enzymatic activities, and secondly, the specific activity of Dehal1 is presumably lower than from recombinant sources.
A panel of murine organs and tissues with a predicted high (thyroid, liver, kidney) vs low or no (heart muscle, skeletal muscle, cerebral cortex) Dehal1 expression was tested in the enzymatic assay. DBT-suppressible Dehal1-dependent deiodination was detected only in the tissues with predicted Dehal1 expression, with thyroid having the highest activity (Figure 3C).
Dehal1 activity of rat thyroid was recently studied and shown to be up-regulated in hypo- and down-regulated in hyperthyroidism as compared with an euthyroid control group (14). Thyroid Dio1 activity was similarly elevated in hypothyroidism but, in contrast to Dehal1, increased even further in hyperthyroidism. The authors concluded that the observed response of thyroid Dehal1 expression is related to the dominant effects of TSH, ie, elevated expression in hypothyroidism due to high TSH concentrations and TSH-dependent signaling raising intracellular cAMP levels, and reduced expression in hyperthyroidism as a response to low TSH. In contrast, Dio1 is induced in hypo- and hyperthyroidism and therefore also in the presence of reduced TSH levels, because the T3-activated TH receptor binds and activates the Dio1 promotor directly (31, 32). Indeed, the expression of DEHAL1 is highest in the thyroid gland among the different tissues, as studied so far (12) and also reflected by our own results (Figure 3C). A local recycling of the MIT- and DIT- bound iodine is thereby efficiently enabled, especially because the enzyme is located at the apical pole of thyrocytes facing the follicular lumen (12). DEHAL1 is also expressed in other tissues except for the thyroid gland. Because MIT and DIT are secreted via the urine (17), a renal expression makes sense for avoiding urinary iodide loss. Kidney DEHAL1 expression could thus be considered as a physiological fail-safe mechanism in case dehalogenation of the iodinated DIT and MIT molecules was inefficient within the thyroid gland and the organism is facing their glomerular filtration and urinary loss.
For these reasons, we decided to test the activity of Dehal1 in kidneys of hypo- and hyperthyroid mice, challenging the notion that TSH constitutes the dominant regulator of Dehal1 expression under hypo- and hyperthyroid conditions. Indeed, Dehal1 activity was highly expressed in kidneys and readily detectable by our novel enzyme assay. DTN proved again to be the superior cosubstrate, qualitatively leading to comparable results as obtained by using NADPH. Our data indicate that renal Dehal1 activity and mRNA expression were significantly reduced in the hyperthyroid mice, similar to the regulation in thyroid as described by Solis-S et al (14). This negative feedback regulation of renal Dehal1 expression was verified in a second animal experiment, again demonstrating decreased activity upon supraphysiological T4 injection. Notably, the underlying mechanism might differ from the TSH-dependent regulation in the thyroid gland, as kidney does not constitute a classical TSH target tissue. However, an expression of TSH receptor mRNA in kidney has been demonstrated (33), which may contribute to the findings reported. Additional intervention experiments and location studies of the enzyme in relation to the TSH receptor and its signaling are needed to test this hypothesis.
Putting our results from the animal experiments in the context of recent literature, we conclude that Dehal1 expression in hyperthyroidism is regulated by tissue-specific mechanisms, not solely depending on TSH concentrations. The efficient down-regulation in the kidney upon T4 administration indicates that it may represent a direct target of TH. On the other hand, some studies have shown extrathyroidal expression of the TSH receptor, which might lead to a more or less pronounced sensitivity against this pituitary-derived hormone. Therefore, the results provided are not sufficient to draw a firm conclusion on the endocrine regulation of renal Dehal1 expression.
As iodide availability is not a limiting factor in hyperthyroidism, the observed down-regulation of renal Dehal1 may cause iodide loss, thereby alleviating surplus TH biosynthesis and contributing to disease control.
The use of DTN as an alternative in vitro cosubstrate is a promising modification to the classical paradigm, because it “overrides” the dependency on endogenous and still unknown NADPH-dependent reductase activity, which is an additional modifying and probably limiting factor for Dehal1 activity.
Published data on the DTN-related mode of action point out that this reaction is not a linear and continuous process and more likely represents an endpoint determination. Therefore, it is probably not adequate to determine specific activities or any other kinetic parameters of Dehal1 using DTN as cosubstrate. However, in the setting where comparative or relative quantification is sufficient, DTN successfully reproduced readouts of altered enzyme activities that were obtained with NADPH but on a higher signal level and with a better signal to noise ratio.
Together, this set of data presents a novel nonradioactive and easily adoptable method to determine DEHAL1 activity from recombinant sources or ex vivo biosamples.
By using the optimized protocol, Dio1 and Dehal1 activities can be determined from the same lysates by a similar and cost-effective assay setup without the need of isotopes or MS equipment.
Acknowledgments
We thank Professor Dr Jens Mittag and Dr Eva K. Wirth for providing animal tissues and support, Dr Nada Rayes and Dr Peter Kühnen for providing human thyroid tissue, and the staff of the CMB animal facility and Gabriele Böhm for technical assistance.
This work was supported by Deutsche Forschungsgemeinschaft Grants RE 3038/1-1 (to K.R.); Scho849/4-1 (to L.S.); SPP 1629 Thyroid Trans Act, KO 922/17-1, and GRK 1208/2 TP 3 (to J.K.); HO 5096/1-1 and (SPP1629 Thyroid Trans Act) HO 5096/2-1 (to C.S.H.); and GRK1957 Adipocyte-Brain Crosstalk (to L.H.) and by Charité funds.
Disclosure Summary: The authors have nothing to disclose.
Abbreviations
- BG
background
- DBT
dibromotyrosine
- DEHAL1
iodotyrosine deiodinase
- DIO
iodothyronine deiodinase
- DIT
diiodotyrosine
- dOD
time-dependent change in absorption
- DTN
dithionite
- EDC
endocrine-disruptive compound
- FAD
flavin adenine dinucleotide disodium salt hydrate
- f.c.
final concentration
- MMI
Methimazole
- MIT
monoiodotyrosine
- MS
mass spectrometry
- NADPH
Nicotinamide adenine dinucleotide phosphate
- qPCR
quantitative real-time polymerase chain reaction
- TH
thyroid hormone
- TT3
total T3
- TT4
total T4.