-
PDF
- Split View
-
Views
-
Cite
Cite
Aya Honma, Yoshiko Yamada, Yuji Nakamaru, Satoshi Fukuda, Ken-ichi Honma, Sato Honma, Glucocorticoids Reset the Nasal Circadian Clock in Mice, Endocrinology, Volume 156, Issue 11, 1 November 2015, Pages 4302–4311, https://doi.org/10.1210/en.2015-1490
- Share Icon Share
The symptoms of allergic rhinitis show marked day-night changes that are likely to be under the control of the circadian clock, but the mechanism of this control is poorly understood. Because most peripheral tissues have endogenous circadian clocks, we examined the circadian rhythm of the clock gene product PERIOD2 (PER2) in the nasal mucosa of male mice using a luciferase reporter and demonstrated for the first time the phase-dependent effects of dexamethasone (DEX) on nasal PER2 rhythm in vivo and ex vivo. The phase shifts in PER2 rhythm caused by DEX were observed around the peak phase of serum glucocorticoids, suggesting that the circadian rhythm of endogenous glucocorticoids regulates the peripheral clock of the mouse nasal mucosa. From the viewpoint of circadian physiology, the best time to administer intranasal steroid treatment for allergic rhinitis would be when no phase shift is caused by DEX: in the early evening in diurnal humans.
Allergic rhinitis (AR) is one of the most common diseases worldwide. In Japan, the current prevalence of AR is approximately 40%, and this has gradually increased since the 1970s (1–3). Allergic symptoms occur at specific times of the day (4, 5). For instance, urticaria worsens before bedtime, whereas asthma worsens in the mid-to-late period of sleep. In patients with AR, allergic symptoms such as sneezing, rhinorrhea, and nasal congestion arise at night and outbreaks occur in the early morning (6, 7). Circadian rhythms in local circulation, vasomotor tone, and in immune reactivity to antigens are considered possible triggers of these symptoms (8–10). A day-night change in the nasal sensitivity to external stimuli was reported in patients with AR. Aerosol methacholine, a muscarinic receptor agonist, induced significantly greater inflammatory responses after a challenge at 6 am than at 3 pm (11). Such time-of-day differences in the efficacy and adverse effects of drug therapy have been reported since the 1980s. For example, mequitazine, an H1-receptor antagonist, was most effective when administered in the evening; indeed, dry mouth, a common adverse effect of antihistamines, was minimized or completely abolished by the evening treatment (12). However, little is known about the role of circadian rhythms in the response of nasal mucosa, which may be responsible for the time-dependent occurrence of AR symptoms.
Circadian rhythms in mammalian physiology and behavior are regulated by the endogenous clock system, which has a hierarchic, multioscillator structure. The master clock is located in the hypothalamic suprachiasmatic nucleus (SCN), and peripheral clocks are distributed throughout the body (13). The SCN master clock is entrained to the day-night cycle by photic signals transmitted via the retinohypothalamic tract and coordinates the peripheral clocks via neural and humoral mechanisms (14). The circadian rhythm is generated in cells by an autoregulatory transcription and translation feedback loop, comprising the clock genes Period (Per)1, Per2, Cryptochrome (Cry) 1, Cry2, Clock, and Bmal1 and their protein products (13). The protein products of Clock and Bmal1 form heterodimers that activate the transcription of Per(s) and Cry(s). In turn, the protein products of Per(s) and Cry(s) suppress the transactivation of CLOCK/BMAL1. One cycle of this feedback loop lasts approximately 24 hours.
The mechanisms underlying circadian variations in AR symptoms may be partly explained by circadian rhythms in these neural and humoral systems. For example, the level of plasma cortisol, which inhibits the production of inflammatory cytokines, is elevated in the morning and declines in the late evening (15). Serum catecholamine, which removes nasal congestion through vasoconstrictor action, also exhibits circadian rhythm: its lowest level occurs between midnight and the early morning (16, 17). Besides the circadian changes in these circulating signals, a putative circadian clock in the nasal tissue may also contribute to day-night changes in AR symptoms from the perspective of reactivity to antigens.
Several peripheral clocks are synchronized by glucocorticoids (18). Blood levels of endogenous glucocorticoids are regulated by the hypothalamus-pituitary-adrenocortical (HPA) axis and are high in the morning in humans. The HPA axis is under the control of the SCN circadian clock and is modulated by stressful stimuli. Despite the fact that glucocorticoids are widely used as an intranasal treatment for AR, the effects of glucocorticoids on the nasal circadian clock remain largely unknown. In this study, we examined the ability of glucocorticoids to reset the nasal circadian clock in vivo and ex vivo. Resetting the circadian clock is experimentally demonstrable by measuring phase-dependent phase shifts of a circadian rhythm of interest after the application of a synchronizer or time cue, which is illustrated as a phase-response curve (PRC). The shape of PRC reflects the steady-state phase relationship of the circadian rhythm with the synchronizer; for example, the time at which the circadian rhythm is at its peak. PRC also predicts dynamic changes in circadian rhythm in response to a phase-shifted synchronizer, such as in the case of air travel across multiple time zones (19). In this study, using mice carrying a luciferase reporter for PER2, we demonstrate circadian rhythms in PER2 levels in the mouse nasal mucosa and phase-dependent phase responses to glucocorticoids in both ex vivo and in vivo experiments.
Materials and Methods
Animals
In this study, we used male homozygous PER2::LUC knock-in mice and wild-type (WT) mice of the C57BL/6J background (8–12 wk old; CLEA Japan). PER2::LUC mice were originally produced at Northwestern University. After receiving them in our laboratory, these mice were backcrossed with WT C57BL/6J mice for more than 10 generations. The mice were reared in our animal facility under controlled environmental conditions: a 12-hour light, 12-hour dark (LD) cycle, with lights on at 6 am local time and a light intensity of approximately 100 lux at the bottom of the cage; temperature of 22 ± 2°C; and humidity of 60 ± 10%. Water and food pellets were available ad libitum. Experiments were conducted in compliance with the rules and regulations established by the Animal Care and Use Committee of Hokkaido University, Sapporo, Japan, under the ethical permission of the Animal Research Committee of Hokkaido University (approval number 13-0064).
Histologic examination
WT mice were euthanized by cervical dislocation at Zeitgeber time (ZT)10, where time of lights-on was defined as ZT0. After decapitation, the skull was cut off coronally behind the eye line. The nose was removed and fixed in 10% formalin for 48 hours, then decalcified in 10% disodium EDTA solution (pH 7.4) for 10 days. The specimen was embedded in paraffin, cut to yield serial coronal sections 6 μm thick, and stained with hematoxylin and eosin. To visualize the localization of PER2-positive cells in the nasal mucosa and the day-night variation in PER2 signal, we performed immunohistochemical examinations. Mice were deeply anesthetized with isoflurane either at ZT0 or ZT12 and transcardially perfused with chilled PBS followed by chilled 4% paraformaldehyde in PBS. After decapitation, the nose was removed and fixed in 4% paraformaldehyde overnight and decalcified in 10% disodium EDTA solution (pH 7.4) for 10 days. The solution was then replaced with 20% sucrose in PBS, and the specimens were stored at −80°C until use. After sectioning coronally at 12 μm thick using a cryostat (Leica), serial slices were placed on aminopropylsilane-precoated glass slides, and immunostaining was performed using the standard Avidin-Biotin complex method (Vector Lab) with polyclonal antimouse PER2 antibody (Alpha Diagnostic) as described elsewhere (20, 21). The product of the enzymatic reaction was detected using 3′3-diaminobenzidine tetrahydrochloride (Sigma-Aldrich).
Quantitative real-time PCR
WT mice were euthanized by cervical dislocation at 4-hour intervals from ZT0 for 24 hours, and the bilateral nasal mucosa was sampled under a stereoscopic microscope (n = 6–8 for each time point). The specimen was submerged in RNA stabilization solution containing RNAlater (Sigma-Aldrich) and stored at −80°C until use. Total RNA was extracted using an RNeasy Micro kit (QIAGEN). RNA (0.35 μg) was reverse transcribed using SuperScript III First-Strand Synthesis SuperMix (Life Technologies). Quantitative PCR was performed using an ABI PRISM 7700 (Applied Biosystems) with a DyNAmo HS SYBR Green quantitative PCR kit (Finnzymes). The housekeeping gene GAPDH was measured in all samples as the internal standard. The relative expressions of Per1, Per2, and glucocorticoid receptor (GR) were calculated relative to the expression of GAPDH mRNA. mRNA expression was quantified using the next specific primers: Per1, 5′-CGTCCTACCTCCTTTATCCAGA-3′ and 5′-TGTTTGCATCAGTGTCATCAGC-3′; Per2, 5′-CATTGAACTTGAGACTGAGGT-3′ and 5′-AAGGGAACACACTGAGAGGAT-3′; GR, 5′-ATGGCGTGAGTACCTCTGGA-3′ and 5′-TCCAGACCCTTGGCACCTAT-3′; and GAPDH, 5′-TGCGACTTCAACAGCAACTC-3′ and 5′-ATGTAGGCCATGAGGTCCAC-3′. The conditions of real time PCR were as follows: 95°C for 15 minutes followed by 45 cycles of 10 seconds at 94°C, 20 seconds at 60°C, and 30 seconds at 72°C.
Tissue preparation for organotypic culture of nasal mucosa
Mice were euthanized by cervical dislocation at ZT9–ZT10. After separation of the nasal bone, the bilateral nasal mucosa was removed from the septum and trimmed to approximately 2 × 2 mm2. Dissection was performed in chilled Hanks’ balanced salt solution under a stereoscopic microscope. The nasal mucosa was placed on a Millicell CM Culture Insert (EMD Millipore Corp) in a 35-mm petri dish and cultured in air at 36.5°C with 1.3-mL DMEM (Invitrogen) supplemented with 10mM N-2-hydroxyethylpiperazine-N′-2-ethane sulfonic acid (HEPES), 2.7mM NaHCO3, 20-mg/L kanamycin (Gibco), 100-μg/mL Apotransferrin (Sigma-Aldrich), 5-μg/mL insulin (Sigma-Aldrich), 100μM putrescine (Sigma-Aldrich), 20nM progesterone (Sigma-Aldrich), 30nM sodium selenite (Gibco), and 0.1mM d-luciferin potassium salt (Dojindo).
Real-time bioluminescence recording and analysis of circadian rhythms
Bioluminescence emitted from the cultured tissue was measured for 1 minute at 10-minute intervals using a photomultiplier tube (LumiCycle; Actimetrics) as previously described (22). Measurement continued for at least 5 consecutive days. The recorded data were detrended using a 24-hour running average subtraction method and further smoothed using a 5-point moving average technique (23). Using a detrended circadian rhythm that intersected the x-axis, the midpoint of 2 intersecting phases in a circadian cycle on the first culture day was defined as the first peak phase. The amplitude (peak-trough difference) of the circadian rhythm was standardized by dividing the amplitude by the peak value because the amplitude and peak value showed a strong positive correlation (r = 0.997). The circadian period was calculated from a regression line fitted to the first to fourth peak phases.
PRC construction. Ex vivo effects of dexamethasone (DEX)
Thirteen microliters of DEX (final concentration, 10−7M; Sigma-Aldrich) or vehicle was added to the culture medium at 1 of the next 4 phases on the fifth culture day: the circadian peak, the following circadian trough, the middle points between the m and the middle point between the peak phase and the preceding trough. After a DEX challenge, bioluminescence was recorded continuously for at least 10 cycles. The amount of phase shift was calculated on the fifth culture day from the phase difference between the 2 regression lines fitted to the 4 consecutive circadian peaks before and after the DEX challenge, respectively. The effects of a lower dose of DEX (final concentration, 10−8M) were also examined at the phases at which 10−7M DEX caused maximum phase delay and phase advance in PER2::LUC rhythm.
PRC construction. In vivo effects of DEX
To examine the effects of DEX in vivo, either DEX (300 μL/mL in PBS) or PBS was ip injected (2 mg/kg) at 1 of the 4 circadian phases (ZT0, ZT6, ZT12, or ZT18). Mice were sacrificed at ZT9–ZT10 after injection (on the same d for ZT0 and ZT6 and on the next d for ZT12 and ZT18), and the nasal mucosa was cultured as described earlier. The first circadian peak phases were used as the reference phase for phase shift.
Adrenalectomy (ADX)
Bilateral ADX was performed using a dorsal surgical approach under isoflurane anesthesia. After surgery, mice were provided 0.9% NaCl solution ad libitum instead of water. Sham operation was performed in exactly the same manner except that the adrenal glands were not removed. Tissue sampling for PER2::LUC rhythm analysis was performed 2 weeks after the surgery.
Statistical analysis
Group data were expressed as the mean ± SEM. For statistical analysis of the circadian rhythm, we used one-way ANOVA with a post hoc Bonferroni test. The difference between the control and experimental groups was evaluated by two-way ANOVA with a post hoc Bonferroni test (Prism 6; GraphPad Software, Inc). The Student’s t test was used to compare means between the 2 groups.
Results
Localization of PER2-positive cells and day-night variation of gene expression in the nasal mucosa
Immunohistochemical examination revealed PER2-positive cells in both the respiratory and olfactory epithelia of the nasal mucosa (Figure 1). In both epithelia, PER2 was faintly expressed in epithelial cells alone at ZT0, whereas PER2 was substantially expressed at ZT12 in epithelial cells, vascular endothelial cells, and the fasciculi of submucosal nerve termini (Figure 1, B and C). In the present study, we examined clock gene expression in the respiratory epithelium of the nasal mucosa, the site most affected by AR (24).

Localization of and day-night variation in PER2-positive cells in the mouse nasal mucosa. Hematoxylin and eosin (HE) staining of the respiratory (A) and olfactory (B) epithelia in the mouse nasal cavity. Immunostaining of the respiratory (C and E) and olfactory epithelia (D and F) collected both during the day (at ZT0; C and D) and night (ZT12; E and F) with anti-PER2 antibody (dark staining of 3,3′-diaminobenzidine) (C–F). PER2 was highly expressed in the epithelial cells, vascular endothelial cells (arrow), and nerve termini (arrowhead). Scale bars, 100 μm.
mRNA expression in the nasal mucosa
In the nasal mucosa, significant circadian variations in the levels of mPer1 and mPer2 mRNA were detected by quantitative real-time PCR (mPer1, P < .05; mPer2, P < .01; n = 6–8) (Figure 2, A and B). Measurement of mPer2 mRNA levels at 4-hour intervals revealed that the circadian peak of mPer2 occurred in the late light period of an LD cycle, and the trough occurred in the late dark period. The amplitude of circadian mPer1 rhythm was not as high as that of mPer2 rhythm, suggesting that its circadian peak occurred between ZT8 and ZT12. Thus, the circadian rhythm of mPer1 mRNA was slightly phase-advanced relative to that of mPer2 mRNA in the nasal mucosa, similar to other areas of the body (25). In contrast, there were no significant circadian changes in the mRNA level of GR (n = 6–8) (Figure 2C).
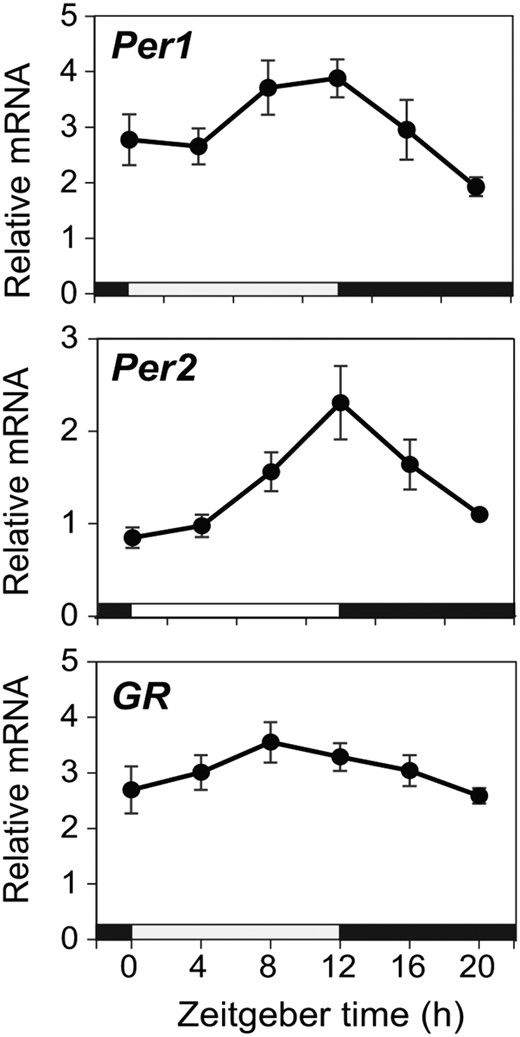
mRNA expression in the mouse nasal mucosa. WT mice were sacrificed at 4-hour intervals, and quantitative real-time PCR analysis was performed for Per1, Per2, and GR mRNA in the nasal mucosa (mean ± SEM, n = 6–8 for each time point). Significant circadian variation was detected in Per1 peaking at ZT8–ZT12 and in Per2 peaking at ZT12 but not in GR. Each value was normalized to that of GAPDH mRNA. Significance was assessed by one-way ANOVA with a post hoc Bonferroni test. Horizontal white and black bars on the abscissa indicate the light and dark times of the LD cycle, respectively.
Circadian PER2::LUC rhythm in cultured nasal mucosa
Cultured nasal mucosa exhibited a robust circadian rhythm in PER2::LUC expression and peaking at the phase corresponding to the early dark period (ZT14.2 ± 0.3) on the first day of culture. We standardized the circadian amplitude in individuals by dividing it by the peak level. The standardized amplitude was 1.7 ± 0.02 on the first culture day, and the circadian period, calculated using 4 consecutive peak phases, was 22.7 ± 0.1 hour. The PER2::LUC rhythm gradually damped over the course of culture at a damping rate of 0.1 ± 0.01, where damping rate was defined as a ratio of the circadian amplitude on the fourth culture day to that on the first culture day (n = 15, mean ± SEM) (Figure 3).
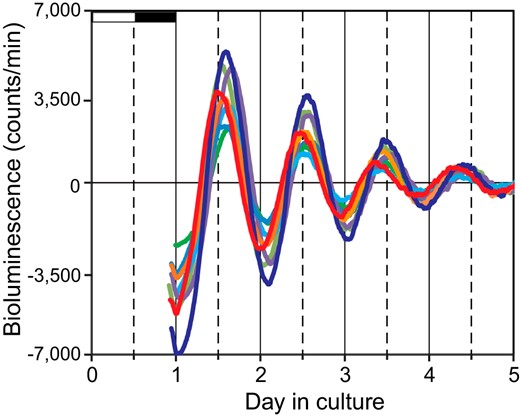
Circadian PER2::LUC rhythm in the cultured nasal mucosa. Eight representative bioluminescence records are shown of 15 cultured nasal mucosa examined for 5 days. The different colors indicate data from different mice. White and black horizontal bars on the abscissa on the first day of culture indicate the light and dark phases of the LD cycle to which the animals were exposed.
Phase-dependent phase shifts in PER2 rhythm caused by DEX in the nasal mucosa ex vivo (ex vivo PRC)
In the ex vivo experiment, the time of circadian peak of PER2::LUC rhythm in culture was defined as circadian time (CT)12, where CT was a time unit obtained by dividing a free-running period by 24 hours. In the ex vivo experiment, a DEX challenge (final concentration, 10−7M) produced phase-dependent phase shifts in the circadian PER2::LUC rhythm (one-way ANOVA, P < .01; n = 4–5 for each time point) (Figures 4 and 5A). The largest phase-delay shift (−10.8 ± 0.7 h) was observed at CT12, and the largest phase-advance shift (7.2 ± 0.8 h) was at CT18. No significant phase shift was detected at CT0. In contrast, there was no significant phase-dependent phase shift in the control group (treated with vehicle alone) (Figure 5A). To construct a PRC for DEX, phase shifts caused by DEX were calculated by subtracting the mean phase shift from the corresponding phase of the control group. The PRC for DEX is asymmetrical, with a large phase-delay portion and a small phase-advance portion (Figure 5B). We also examined phase shifts caused by a lower dose of DEX (10−8M) at the time points at which 10−7M DEX caused maximum phase delay (CT12) and phase advance (CT18). This lower dose of DEX (10−8M) induced significant phase-delay shifts at CT12 to an extent that was similar to those induced by a higher dose (10−7M) (one-way ANOVA with a post hoc Bonferroni test, P < .01, vs vehicle). But 10−8M DEX did not induce significant phase shifts at CT18 (Figure 5E).
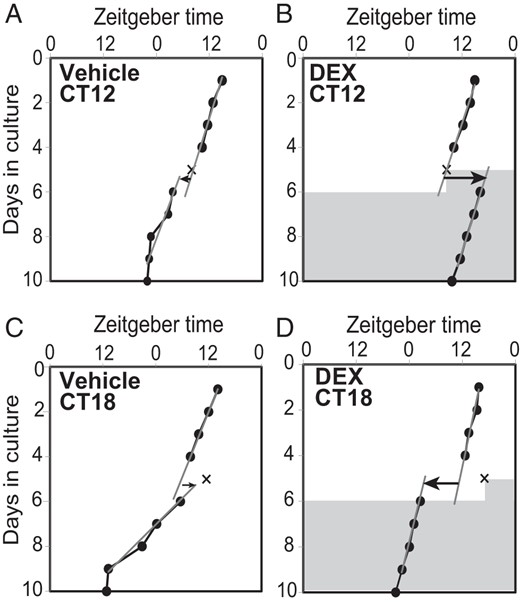
Phase shifts in PER2 rhythm caused by DEX. The circadian peak phases of PER2 rhythm (closed circles and solid lines) in cultured mouse nasal mucosa before and after the application of vehicle (A and C) or DEX (B and D). One representative phase plot is shown for vehicle or DEX applied on the fifth culture day in the peak phase (CT12; A and B) or at CT18 (C and D). The timing of application is indicated by the X. DEX application induced a large phase-delay (B) or phase-advance shift (D), but vehicle did not cause a shift at either phase (A and C). Shadowed areas indicate DEX in the culture media at a concentration of 10−7M. Gray oblique lines in the graph indicate regression lines fitted to 4 consecutive peak phases before and after the application of DEX or vehicle. Arrows on the sixth culture day indicate the direction and amount of phase shifts. The abscissa indicates the time to which mice were entrained.
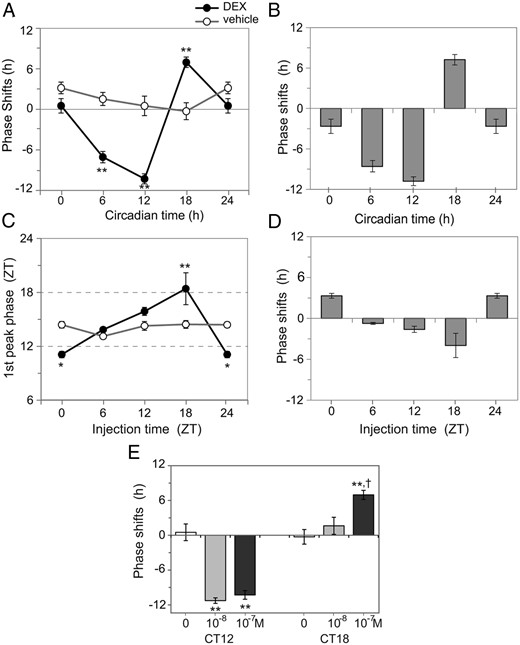
Construction of ex vivo and in vivo PRCs. Phase shifts after the addition of DEX (closed circles with a black line) or vehicle (open circles with a gray line) into the culture media were plotted against the phase of their addition (A). Data are expressed as mean ± SEM (n = 4–5 for each time point). The ex vivo PRC for DEX was constructed by calculating the difference in the phase shift caused by DEX from that of the respective mean value obtained from the control group (B). The phase-dependent effect of DEX on the nasal circadian clock in vivo were examined by injecting DEX (closed circles with a black line) or PBS (open circles with a gray line) at 4 different circadian phases and measuring the first peak phases of circadian PER2 rhythm in cultured nasal mucosa (C). Data are expressed as mean ± SEM (n = 5 for each time point). The in vivo PRC for DEX was constructed by calculating the difference in first circadian peak phases between the DEX and control groups (D). In both the ex vivo and in vivo experiments, phase shifts were phase dependent, with statistical significance in the DEX group but not in the control group (two-way ANOVA with a post hoc Bonferroni test; *, P < .05 and **, P < .01). Dose dependency of phase shifts induced by DEX was examined ex vivo at the maximum phase-delaying phase (CT12) and phase-advancing phase (CT18) (E). Abscissa shows final concentration of DEX. The data representing 0M (vehicle control) and 10−7M are the also shown in A. Data are expressed as mean ± SEM (n = 4–6 for each time point). Statistically significant differences were detected in phase shifts at CT12 and CT18 (one-way ANOVA with a post hoc Bonferroni test; **, P < .01 vs vehicle; †, P < .05 10−7M vs 10−8M).
Phase-dependent phase shifts in PER2 rhythm caused by DEX in the nasal mucosa in vivo (in vivo PRC)
DEX was ip injected at 4 different ZTs to construct a PRC of the nasal mucosa for DEX in vivo. The nasal mucosa was cultured, and the first circadian peak was compared with that of the control group, which was injected with PBS alone. The first circadian peak in the control group was observed at a fixed time of the day (ZT14.1 ± 0.3; n = 20) regardless of the injection time, whereas in the DEX group, the peak phase shifted depending on the injection time (P < .01; n = 5) (Figure 5C). A significant difference was evident in phase response between the DEX and control groups (P < .01; n = 5). The amount of phase shift caused by DEX injection was calculated by subtracting the mean circadian peak phase of the control group at the corresponding ZT from the individual peak phases of the DEX group. DEX injection yielded phase-dependent phase shifts in circadian PER2::LUC rhythm, with the largest phase-delay shift (−4.0 ± 1.8 h) at ZT18, and the largest phase-advance shift (3.3 ± 0.4 h) at ZT0 (Figure 5D). There was no significant difference in the amplitude of rhythm or the circadian period between the DEX group (amplitude, 1.7 ± 0.03; circadian period, 22.8 ± 0.1; n = 20) and the control group (amplitude, 1.7 ± 0.02; circadian period, 22.7 ± 0.1; n = 20). In addition, neither the first circadian peak phase nor its amplitude differed between the PBS-injected control group (first circadian peak phase, ZT14.1 ± 0.3; amplitude, 1.7 ± 0.02; n = 20) and the untreated control group (first circadian peak phase, ZT14.2 ± 0.3; amplitude, 1.7 ± 0.02; n = 15).
The effects of endogenous glucocorticoids on the peripheral circadian clock in nasal mucosa were examined in ADX mice. The first circadian peak of the PER2::LUC rhythm in cultured nasal mucosa was located at ZT14.8 ± 0.4, (n = 6), indicating a delay, although not a statistically significant one, when compared with the peak phase in the sham operation group (ZT13.9 ± 0.6, n = 6). There was also no significant difference between the ADX and sham operation groups in the amplitude (ADX, 1.7 ± 0.03; sham, 1.7 ± 0.01) or the period (ADX, 23.0 ± 0.2 h; sham, 23.1 ± 0.1 h) of PER2::LUC rhythms.
Effects of DEX on circadian properties other than phase
A DEX challenge dramatically restored the dampened circadian PER2::LUC rhythm in the ex vivo experiment (Figure 6A). The recovery rate of the circadian amplitude caused by DEX was 3.3 ± 0.4 (n = 17), where the recovery rate was defined as the ratio of the circadian amplitude on the sixth day of culture (a d after the DEX challenge) to that on the fourth day of culture (a d before the DEX challenge). Such recovery of the circadian amplitude was not observed in the control group (recovery rate, 0.2 ± 0.03; n = 16). There was no significant phase dependency in recovery rate (n = 4–5) (Figure 6B) after the administration of either DEX or vehicle alone. On the other hand, the amplitude damping rate of consecutive circadian rhythms did not differ significantly between before (d 2/d 1) and after (d 7/d 6) DEX challenge (before, 0.6 ± 0.03; after, 0.5 ± 0.01; n = 17).
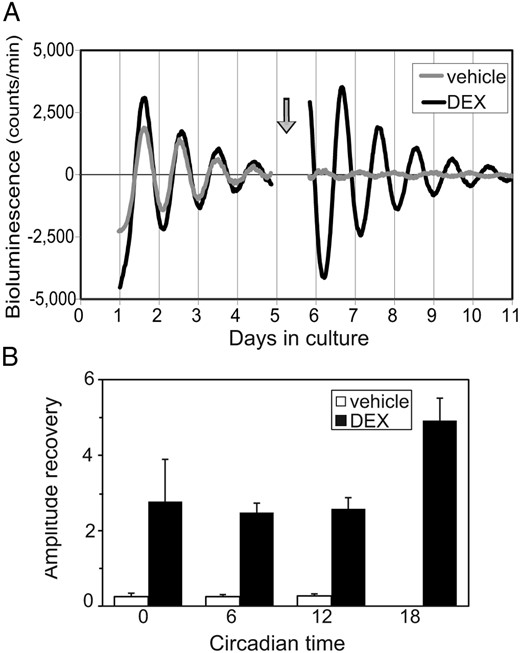
Effects of DEX on the amplitude of circadian PER2 rhythm. Representative PER2::LUC rhythms before and after the application of DEX (black) or vehicle (gray) at the time indicated by an arrow (A). Rates of recovery of the circadian amplitude after the addition of DEX (closed column) or vehicle (open column) are expressed as mean ± SEM (n = 4–5 for each time point) (B). In both the DEX and control groups, statistically significant phase dependency in recovery rate was not detected. The control data at CT18 was too small for visualization (0.2 ± 0.1).
Discussion
The mammalian circadian clock comprises the master clock in the SCN and peripheral clocks in a variety of tissues (13). We identified the peripheral circadian clock in the mouse nasal mucosa, and demonstrated for the first time phase-dependent phase shifts caused by DEX. The peak of circadian PER2::LUC rhythm in the nasal mucosa was observed to occur in the early subjective night, consistent with PER2 immunohistochemistry and mRNA levels as measured by real-time PCR. This circadian rhythm was reminiscent of the rhythm in the lower respiratory tissues (26). Gibbs et al (27) reported that the pulmonary circadian clock is located in epithelial club (Clara) cells, the physiologic function of which is to protect the bronchiolar epithelium from external hazardous agents by secreting various substances similar to lung surfactant. They also showed that club cells, acting through a single neutrophil chemokine, CXCL5, mediate the circadian variation in lung inflammation (28). In the nasal mucosa, immunohistochemical staining identified PER2-immunopositive cells in the epithelium. Epithelial cells, the front line in the protection of the body from physical, chemical, and pathogenic microbial challenges, may be the key component of the nasal circadian clock.
AR symptoms exhibit prominent circadian variation, arising at night and worsening in the early morning (6, 7). Recent studies have suggested involvement of the circadian clock in IgE/mast cell-mediated allergic reaction. Nakamura et al (29) reported that the passive cutaneous anaphylactic reaction showed circadian variation in WT mice, with a nadir around the beginning of the subjective night, whereas the reaction remained constantly at the peak level of WT mice throughout the day in Per2 mutant mice, which are unable to produce Per2 protein. They also showed that ADX abolishes circadian rhythm. These results support the hypothesis that IgE/mast cell-mediated allergic reaction is regulated by the clock gene Per2 via the rhythmic secretion of glucocorticoids from the adrenal glands and/or the time-dependent response of mast cells to glucocorticoids.
Glucocorticoids effectively suppress inflammatory reactions in the nasal mucosa (30). They also regulate immune functions by inducing regulatory T cells, which function in immune tolerance and homeostasis (31). The temporal order of glucocorticoid secretion is regulated by the SCN circadian pacemaker and is regarded as an important humoral messenger that communicates circadian signals from SCN to the peripheral clocks, in turn synchronizing the peripheral clocks to the master pacemaker (18). Our findings also demonstrated that glucocorticoids have a strong synchronizing effect on circadian rhythms in the nasal mucosa. Both the ex vivo and in vivo PRCs exhibit a large phase-delay portion and a relatively small phase-advance portion, consistent with the PRCs of behavior rhythms in response to photic signals in mice (32). However, the amplitude of the ex vivo PRC was much larger than that of the in vivo PRC, which appears to be a general feature of PRCs (18, 33). In addition, entraining circadian signals from the SCN may have counteracted the phase-shifting effect of DEX because animals were entrained by LD. The point of crossover from phase-delay to phase-advance (between ZT12 and ZT18 for the ex vivo PRC and between ZT18 and ZT24 for the in vivo PRC) is also slightly different, which could be an effect of culture (34, 35). The in vivo PRC suggests that endogenous glucocorticoids control the peripheral clock in the mouse nasal mucosa. Serum levels of endogenous glucocorticoids in mice are high from the late light to the early dark periods (ZT8–ZT14), in which phase-delay shifts can be induced by DEX. Because the mouse circadian clock has a period shorter than 24 hours, it needs to undergo a phase-delay shift to synchronize with a 24-hour LD cycle (32). In addition, PRC of the circadian rhythm in the nasal mucosa predicts the degree and direction of phase shift produced by exogenous glucocorticoids.
In the present study, DEX was bath applied. Although the exact half-life of DEX in the culture medium is not known, the phase-shifting effect of DEX must have taken place instantaneously after DEX application because even the largest phase shift was accomplished within a day, and steady-state free-runs followed (Figure 4). Phase shifts were induced by DEX in a dose-dependent manner. A lower dose of DEX did not induce significant phase shifts when applied at the point at which a higher dose of DEX induced maximum phase-advance shift (CT18). By contrast, the lower dose of DEX produced a full phase-delay shift of nearly 12 hours when applied at the time at which a higher dose induced maximum delay shift (CT12). The asymmetric dose-response in the PRC suggests that different mechanisms are involved in the phase-delay and phase-advance shifts induced by DEX. Consistent with the present results, several previous studies have suggested that different mechanisms are involved in the process of light-induced phase-delay and phase-advance shifts (36–38).
To our surprise, ADX had little effect on the circadian parameters of PER2::LUC rhythms in the cultured nasal mucosa. The effects of ADX on circadian rhythms are reported to differ among various tissues and among genes. ADX has no effect on peripheral clocks in the pituitary gland, lung, and some extra SCN brain structures (39–41). Although ADX abolishes circadian rhythms in the expression of a variety of genes involved in metabolism in the liver, it has no effects on those of clock or clock-related genes (42). These results suggest that signals other than endogenous steroids, such as norepinephrine released from the sympathetic nerve, may exert compensatory effects on resetting the clock in the absence of glucocorticoids, as suggested in the liver (43). The redundancy of time cues would be advantageous for the circadian clock in the nasal mucosa to protect the body from external perturbations.
In the present study, we found that the expression of GR mRNA in the nasal mucosa remained unchanged throughout the day (Figure 2). The phase-dependent phase-shifting effect of DEX can be attributed to the molecular feedback loop of circadian rhythm generation. Circadian rhythms in the GR functions and their downstream factors may also have a complementary role in phase dependency. So et al (44) demonstrated that the glucocorticoid-response element located in the Per2 promoter was continuously occupied by GR during rhythmic Per2 expression and was essential for glucocorticoid regulation of the clock gene in vivo. Glucocorticoid-activated GR moves into the nucleus and binds to glucocorticoid-response element in the Per2 promoter, enhancing Per2 transcription. Changes in the rate of Per2 transcription can accelerate or decelerate the turnover of the molecular feedback loop of circadian rhythm generation, resulting in a phase shift (45).
In the present study, the circadian amplitude increased after the DEX challenge, but the increment of amplitude was the same throughout the circadian day. The mechanism of amplification caused by DEX is unknown, but the amplification seems to subside within 1 cycle, because the amplitude damping rate did not differ before and after DEX challenge. Furthermore, the amplification is unlikely to be mediated through the circadian clock, because the increase in rhythm amplitude was not correlated with the extent of phase shift. A lack of phase dependency in DEX-induced amplification suggests an absence of oscillating component in the intracellular signal transduction pathways of DEX.
Intranasal steroids (INSs) are potent agents for treatment of AR, and some of currently available INSs are efficacious with once-daily use (46–48). INSs are not absorbed easily into the systemic circulation and are metabolized rapidly, and no significant adverse effect on the HPA axis has been reported (49). From the viewpoint of circadian physiology, the best time for INS would be from ZT0–ZT6, the early-to-mid light phase, when no phase shift is caused by DEX. In the case of diurnal humans, this corresponds to the early evening. This is consistent with findings that aerosol corticosteroid administered at this time was most effective for the treatment of asthma (50, 51). Inappropriately timed INS administration would shift the circadian clock of the nasal mucosa to desynchronize it from other circadian rhythms in the body as well as from the SCN central clock. Repeated internal desynchronization was reported to increase the risk of a number of medical problems in humans and was associated with mortality in aged mice (52, 53). In addition, phase shifts of the nasal clock to an undesired direction may obscure the optimal timing of following treatments.
In conclusion, our results provide evidence of a mechanism of resetting the circadian clock in the nasal mucosa for the first time. The circadian PER2 rhythm in the mouse nasal mucosa shows phase-dependent phase shifts after a pulse of DEX both ex vivo and in vivo. The PRC obtained suggests that endogenous glucocorticoids are potent synchronizers of the peripheral circadian clock in the nasal mucosa.
Acknowledgments
We thank J. S. Takahashi for supplying PER2::LUC mice and M. Shindo and T. Kitamura for providing technical advice.
This work was supported in part by Creation of Innovation Centers for Advanced Interdisciplinary Research Areas Program, Ministry of Education, Culture, Sports, Science and Technology, Japan and by the Grant-in-Aid for Scientific Research from Japan Society for the Promotion of Science (Grant No. 24390055).
Disclosure Summary: The authors have nothing to disclose.
Abbreviations
- ADX
adrenalectomy
- AR
allergic rhinitis
- Cry
Cryptochrome
- CT
circadian time
- DEX
dexamethasone
- GR
glucocorticoid receptor
- HPA
hypothalamus-pituitary-adrenocortical
- INS
intranasal steroid
- LD
12-hour light, 12-hour dark
- Per
Period
- PRC
phase-response curve
- SCN
suprachiasmatic nucleus
- WT
wild type
- ZT
Zeitgeber time.