-
PDF
- Split View
-
Views
-
Cite
Cite
Muraly Puttabyatappa, Catharine A. VandeVoort, Charles L. Chaffin, hCG-Induced Down-Regulation of PPARγ and Liver X Receptors Promotes Periovulatory Progesterone Synthesis by Macaque Granulosa Cells, Endocrinology, Volume 151, Issue 12, 1 December 2010, Pages 5865–5872, https://doi.org/10.1210/en.2010-0698
- Share Icon Share
An ovulatory stimulus induces the rapid and dramatic increase in progesterone synthesis by the primate ovarian follicle. However, little is known about the early events leading to the shift from estrogen to progesterone production. Because steroidogenesis represents an aspect of cholesterol metabolism, it was hypothesized that transcription factors regulating cholesterol balance would be among the earliest to change in response to an ovulatory stimulus. Granulosa cells were isolated from rhesus monkey follicles following controlled ovarian stimulation protocols before or up to 24 hr after an ovulatory human chorionic gonadotropin (hCG) bolus. The peroxisome proliferator-activated receptor-γ (PPARG) and the liver X receptors [nuclear receptor (NR)1H2, NR1H3] decreased within 3 hr of hCG, as did the reverse cholesterol transporters ATP-binding cassette (ABC)A1 and ABCG1. Treatment of granulosa cells isolated before an ovulatory stimulus with hCG and rosiglitizone resulted in an increase in NR1H3 and ABCG1, and decreased steroidogenic acute regulatory (STAR) protein and scavenger receptor-BI (SCARB1). A liver X receptor agonist attenuated hCG-induced progesterone synthesis in vitro and increased the expression of ABCA1 and ABCG1, and suppressed STAR, P450 side-chain cleavage A1, hydroxysteroid dehydrogenase 3B, and SCARB1. These data suggest that an initial action of LH/CG on the primate preovulatory follicle is to rapidly reduce the expression of PPARG, resulting in reduced NR1H3 with the consequence shifting the balance from cholesterol efflux via ABCA1 and ABCG1 to cholesterol uptake (SCARB1) and metabolism (STAR, P450 side-chain cleavage A1, hydroxysteroid dehydrogenase 3B). That the regulation of PPARG and the liver X receptors occurs within 3 hr strongly indicates that early events in the primate luteinizing follicle are critical to successful ovulation and luteal formation.
The midcycle surge in LH initiates a series of events leading to ovulation and differentiation of the follicular remnant into the corpus luteum (1, 2). One of the key events in this process is the rapid and dramatic increase in progesterone production (1). The concentration of serum progesterone doubles within 30 min of an ovulatory human chorionic gonadotropin (hCG) bolus given to rhesus macaques undergoing controlled ovarian stimulation (COS) cycles, whereas intrafollicular progesterone levels increase 40-fold to approach 2000 ng/ml within 3 h of hCG (3, 4).
Macaque granulosa cells have sufficient stores of cholesterol ester to support progesterone synthesis in vitro for about 24 h; thereafter, progesterone production returns to baseline unless an exogenous source of cholesterol ester is supplied (5). The uptake of cholesterol esters from lipoproteins (specifically low-density lipoprotein) is hypothesized to be via hCG-induced low-density lipoprotein receptor (LDLR) and scavenger receptor-BI (SCARB1) (5–8). Therefore, it seems likely that in luteal phase species such as macaques (and humans), there are two distinct phases of progesterone synthesis: a potentially nongenomic action of LH/CG directly at the level of lipid droplets and a second step leading to changes in gene expression necessary for prolonged steroidogenesis, including steroidogenic acute regulatory (STAR) protein, P450 side-chain cleavage A1 (CYP11A1), and 3βHSD (HSD3B2).
An ovulatory stimulus also modulates the expression and activity of an array of transcription factors important in the expression of genes involved in steroidogenesis (reviewed in Ref. 9). Peroxisome proliferator-activated receptor-γ (PPARG) and liver X receptors [LXRα/nuclear receptor (NR)1H3 and LXRβ/NR1H2] are transcription factors that regulate genes involved with cholesterol homeostasis such as the ATP-binding cassette (ABC) family of cholesterol transporters, including ABCA1 and ABCG1 (10–12). Whereas PPARG is generally thought to be down-regulated after ovulatory stimulus in the rats (13–16), the activity of PPARG may be cell and/or species specific because it can either augment or inhibit steroidogenesis (17–19). NR1H2 and NR1H3 are expressed by luteinized human granulosa cells derived from in vitro fertilization cycles and in the primate corpus luteum (20, 21), although the expression during the critical transition from nonluteinized to luteinized is not known. Importantly, treatment of human granulosa-luteal cells with an LXR agonist reduces progesterone synthesis (20). Although these data highlight the role of PPARG, NR1H2, and NR1H3 in luteinizing granulosa cell steroidogenesis, intermediary gene targets are not known.
Because cholesterol uptake and metabolism are critical steps in progesterone synthesis, and because PPARG and NR1H2 and -3 promote cholesterol efflux as a means to maintain intracellular cholesterol balance, it was hypothesized that an ovulatory stimulus rapidly decreases the expression of both genes in macaque granulosa cells. The reduction in PPARG, NR1H2, and NR1H3 was further expected to be associated with increased expression of genes key for steroidogenesis, as well as progesterone synthesis overall. Finally, a direct regulatory link between PPARG and NR1H2 was determined. The overall hypothesis of this work is that an ovulatory stimulus induces progesterone production in part by reducing the expression PPARG, NR1H2, and NR1H3, the gene products of which are predicted to repress genes involved in steroidogenesis and cholesterol uptake and promote the expression of genes associated with cholesterol efflux in macaque granulosa cells.
Materials and Methods
Animals
Adult female rhesus macaques (Macaca mulatta) were housed at the California National Primate Research Center as described previously (22). After the onset of menstruation, adult female rhesus monkeys were treated with recombinant human FSH [Ares-Serono (Randolph, MA) or Organon (West Orange, NJ); 37.5 IU, im, twice daily] for 7 d. Antide (Ares-Serono; 5 mg/kg body weight, sc, single injection daily) was administered daily to prevent endogenous gonadotropin secretion. Follicles were aspirated the morning after the last dose of recombinant human FSH by an ultrasound-guided procedure as described previously (22), and the characteristics of the follicular cohort in this model have been described (23). The resulting cells are referred to as nonluteinized granulosa cells. A subset of animals received an ovulatory bolus of recombinant human CG (1000 IU, sc, Ares-Serono) on the morning of d 8, and follicles were aspirated before (0 h) or 3, 6, 12, and 24 h after hCG (n ≥ 3/ time point). Aspirates representing the pooled contents of multiple follicles from each animal were maintained at approximately 35 C within a temperature-controlled isolette at all times. Oocytes were removed by transferring the aspirate to a 24-mm diameter, 70-μm pore size filter (Netwell Inserts 3479, Corning, Inc., Acton, MA), and the tube was rinsed with fresh Tyrode’s lactate (TL)-HEPES/0.1 mg/ml polyvinyl acetate (PVA) that was also poured onto the filter. This rinse was repeated until blood cells were removed from the filter. Granulosa cells were recovered by centrifugation of the cell suspension for 5 min at 300 × g to pellet the red cells and then increased to 500 × g for an additional 5 min, resulting in a thin layer of granulosa cells over the red cell pellet. The layer of granulosa cells was transferred to a 40% Percoll gradient in medium 199 (Sigma-Aldrich Corp., St. Louis, MO) and centrifuged for 30 min at 500 × g. The granulosa cells were recovered from the surface of the Percoll with a Pasteur pipette and washed twice with TL-HEPES-PVA and centrifuged at 500 × g for 10 min. The cell pellet was resuspended in 1 ml TL-HEPES-PVA in a 15-ml centrifuge tube, and total cell number was determined on a hemocytometer. An additional 14 ml TL-HEPES-PVA was added to the cell suspension, and tubes were capped and sealed with Parafilm. The cells were shipped in a biohazard shipping container by overnight delivery at ambient temperature from September to June. All animal procedures were performed in accordance with the NIH Guide for the Care and Use of Laboratory Animals and were approved by the University of California Davis and the University of Maryland Baltimore animal care and use committees.
Cell culture and treatments
Nonluteinized granulosa cells were seeded at a density of 2 × 105 viable cells per well in 24-well fibronectin-coated plates (Biocoat; BD Biosciences, Bedford, MA) in 0.4 ml DMEM/Ham’s F-12 medium supplemented with penicillin/streptomycin (50 U/ml), and 25 ng/ml hFSH with or without 20 IU/ml hCG to induce luteinization and incubated at 37 C in 5% CO2 cell culture incubator (5). Addition of agonists and/or antagonists was done as per the requirement of the experiment. PPARG agonist rosiglitazone (RGT) potassium salt and PPARG antagonist GW9662 were from Cayman Chemicals (Ann Arbor, MI); LXR agonist T0901317 was from Sigma-Aldrich. These were dissolved in DMSO vehicle and used at 1% (vol/vol) as the control or with pharmacological agents as detailed in Results and figure legends.
RT-PCR
Total RNA was extracted using RNaqueous Micro RNA isolation kit (Applied Biosystems, Foster City, CA) and reverse transcribed with Moloney murine leukemia virus reverse transcriptase (Invitrogen, Carlsbad, CA). Real-time RT-PCR by TaqMan method was performed for NR1H3 (Hs00172885_m1), NR1H2 (Hs01027215_g1), ABCA1 (Hs00194045_m1), and ABCG1 (Hs01555189_g1) using primer-probe combinations available as TaqMan Gene Expression Assays (Applied Biosystems). Real-time PCR primers and probes for the other genes (Table 1) were custom designed using the PrimerExpress software (Applied Biosystems) and synthesized by Applied Biosystems custom primer-probe synthesis service. Real-time RT-PCR was carried out for both the target gene and the endogenous control in the same reaction as previously reported (5). For relative quantification of mRNA levels, a standard curve was generated using a pool of previously validated cDNA prepared from luteinized granulosa cells. The target gene mRNA levels were normalized to RPL19.
Gene . | Forward primer . | Reverse primer . | Probe . |
---|---|---|---|
PPARG | AAAGGCGAGGGCGATCTT | CCCATCATTAAGGAATTCATGTCATA | 6FAM-CAGGAAAGACAACAGACAAAT CACCATTCGT |
SCARB1 | TTCCTCGAGTACCGCACCTT | ATGTTGGGCATGACGATGTAGTC | 6FAM-CCTCCAAGTCCCACGGCTCGG |
LDLR | GGCAGTGTGACCGGGAATAT | AGTCTCTAGCCATGTTGCAGACTTT | 6FAM-ACACTCTGCGAGGGACCCAACAAGTTC |
STAR | CCACCCCTAGCACGTGGAT | TCCTGGTCACTGTAGAGAGTCTCTTC | 6FAM-CGGAGCTCTCTACTCGGTTCTC |
CYP11A1 | CTTCTTCGACCCGGAAAATTT | ATCCGCCGTCCCAGACA | 6FAM-ACCCAACCCGATGGCTGAGCAA |
HSD3B | CCAGAACGGCCACGAAGA | AGCTTTTTGCTGTACGGGTATG | 6FAM-AGCCTCTGGAAAACACATGGCCCA |
RPL19 | CCCCAATGAGACCAATGAAATC | CAGCCCATCTTTGATGAGCTT | VIC-ATGCCAACTCCCGTCAGCAGATCC |
Gene . | Forward primer . | Reverse primer . | Probe . |
---|---|---|---|
PPARG | AAAGGCGAGGGCGATCTT | CCCATCATTAAGGAATTCATGTCATA | 6FAM-CAGGAAAGACAACAGACAAAT CACCATTCGT |
SCARB1 | TTCCTCGAGTACCGCACCTT | ATGTTGGGCATGACGATGTAGTC | 6FAM-CCTCCAAGTCCCACGGCTCGG |
LDLR | GGCAGTGTGACCGGGAATAT | AGTCTCTAGCCATGTTGCAGACTTT | 6FAM-ACACTCTGCGAGGGACCCAACAAGTTC |
STAR | CCACCCCTAGCACGTGGAT | TCCTGGTCACTGTAGAGAGTCTCTTC | 6FAM-CGGAGCTCTCTACTCGGTTCTC |
CYP11A1 | CTTCTTCGACCCGGAAAATTT | ATCCGCCGTCCCAGACA | 6FAM-ACCCAACCCGATGGCTGAGCAA |
HSD3B | CCAGAACGGCCACGAAGA | AGCTTTTTGCTGTACGGGTATG | 6FAM-AGCCTCTGGAAAACACATGGCCCA |
RPL19 | CCCCAATGAGACCAATGAAATC | CAGCCCATCTTTGATGAGCTT | VIC-ATGCCAACTCCCGTCAGCAGATCC |
Gene . | Forward primer . | Reverse primer . | Probe . |
---|---|---|---|
PPARG | AAAGGCGAGGGCGATCTT | CCCATCATTAAGGAATTCATGTCATA | 6FAM-CAGGAAAGACAACAGACAAAT CACCATTCGT |
SCARB1 | TTCCTCGAGTACCGCACCTT | ATGTTGGGCATGACGATGTAGTC | 6FAM-CCTCCAAGTCCCACGGCTCGG |
LDLR | GGCAGTGTGACCGGGAATAT | AGTCTCTAGCCATGTTGCAGACTTT | 6FAM-ACACTCTGCGAGGGACCCAACAAGTTC |
STAR | CCACCCCTAGCACGTGGAT | TCCTGGTCACTGTAGAGAGTCTCTTC | 6FAM-CGGAGCTCTCTACTCGGTTCTC |
CYP11A1 | CTTCTTCGACCCGGAAAATTT | ATCCGCCGTCCCAGACA | 6FAM-ACCCAACCCGATGGCTGAGCAA |
HSD3B | CCAGAACGGCCACGAAGA | AGCTTTTTGCTGTACGGGTATG | 6FAM-AGCCTCTGGAAAACACATGGCCCA |
RPL19 | CCCCAATGAGACCAATGAAATC | CAGCCCATCTTTGATGAGCTT | VIC-ATGCCAACTCCCGTCAGCAGATCC |
Gene . | Forward primer . | Reverse primer . | Probe . |
---|---|---|---|
PPARG | AAAGGCGAGGGCGATCTT | CCCATCATTAAGGAATTCATGTCATA | 6FAM-CAGGAAAGACAACAGACAAAT CACCATTCGT |
SCARB1 | TTCCTCGAGTACCGCACCTT | ATGTTGGGCATGACGATGTAGTC | 6FAM-CCTCCAAGTCCCACGGCTCGG |
LDLR | GGCAGTGTGACCGGGAATAT | AGTCTCTAGCCATGTTGCAGACTTT | 6FAM-ACACTCTGCGAGGGACCCAACAAGTTC |
STAR | CCACCCCTAGCACGTGGAT | TCCTGGTCACTGTAGAGAGTCTCTTC | 6FAM-CGGAGCTCTCTACTCGGTTCTC |
CYP11A1 | CTTCTTCGACCCGGAAAATTT | ATCCGCCGTCCCAGACA | 6FAM-ACCCAACCCGATGGCTGAGCAA |
HSD3B | CCAGAACGGCCACGAAGA | AGCTTTTTGCTGTACGGGTATG | 6FAM-AGCCTCTGGAAAACACATGGCCCA |
RPL19 | CCCCAATGAGACCAATGAAATC | CAGCCCATCTTTGATGAGCTT | VIC-ATGCCAACTCCCGTCAGCAGATCC |
RIA assay
Progesterone concentrations were determined using commercially available coated-tube RIA kits (Siemens Healthcare Diagnostics, Inc., Deerfield, IL). All samples were determined in a single assay, and the within-assay variability was 0.50%.
Statistical analysis
All data were tested for heterogeneity of variance using a Bartlett’s χ2. Data not normally distributed were transformed to log+2 before analysis by ANOVA with one repeated measure followed by Bonferroni post tests. Differences were considered significant when P < 0.05. Data for steroid measurements are presented as the percentage of FSH controls to normalize the typical variability existing between nonhuman primates. All data are presented as mean ± sem from three to four samples unless otherwise stated.
Results
Expression profile of PPARG, NR1C3, NR1C2, ABCA1, and ABCG1 during luteinization in vivo
Before an hCG bolus in vivo, PPARG, NR1H3, NR1H2, ABCA1, and ABCG1 mRNAs were all highly expressed in the granulosa cells (Fig. 1). PPARG, NR1H3, ABCA1, and ABCG1 mRNA decreased 3 h after hCG (10-, 9-, 5-, and 95-fold, respectively; P < 0.05), whereas NR1H2 mRNA was reduced significantly 12 h after hCG (12-fold; P < 0.05) (Fig. 1C). The expression of all these mRNAs remained reduced through 24 h.
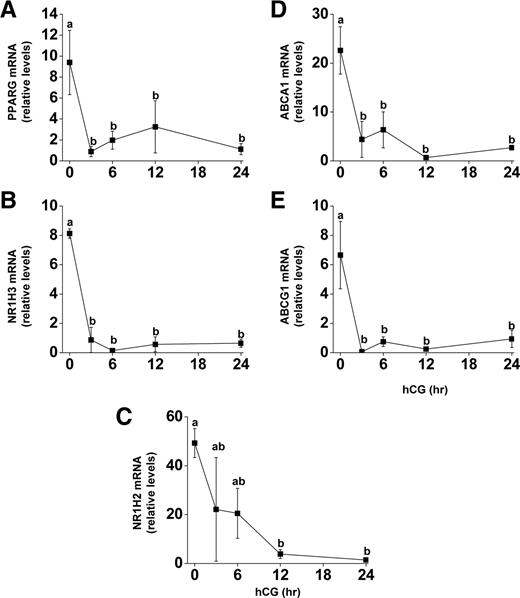
mRNA expression of PPARG, NR1H2, NR1H3, ABCA1, and ABCG1 during luteinization in vivo. Follicles were aspirated before (0) or up to 24 h after an ovulatory bolus of hCG was administered to rhesus macaques undergoing COS protocols. Granulosa cells were processed for RNA and real time RT-PCR analysis for (A) PPARG, (B) NR1H3, (C) NR1H2, (D) ABCA1, and (E) ABCG1 was performed. Expression levels of all genes were normalized to the internal standard RPL19 and plotted as relative mRNA levels. Time points with different lowercase letters are significantly different (P < 0.05; n = 3–5 per time point).
Effect of PPARG agonist treatment on LXR and steroidogenic gene expression
To examine the effects of PPARG on the expression of NR1H3 and NR1H2, nonluteinized granulosa cells were treated with FSH or FSH+hCG in the absence or presence of 0.25–1.0 μm RGT. NR1H3 mRNA was not different after hCG treatment compared with FSH alone (Fig. 2). In the presence of hCG, 1.0 μm RGT increased NR1H3 mRNA expression, and the PPARG antagonist GW9662 was able to prevent this induction (Fig. 2). In contrast, hCG reduced mRNA levels of NR1H2 whereas RGT was without effect (Fig. 2). Based on these data, 1.0 μm RGT was used to further characterize PPARG regulation of gene expression. Because NR1H3 mRNA expression increased after RGT treatment at 24 h, treatment was continued for an additional 24 h to allow for NR1H3 activity. RGT increased the expression of NR1H3 and ABCG1 at both 24 and 48 h, whereas ABCA1 mRNA was not clearly changed by RGT (Fig. 3, A–C). STAR, SCARB1, and LDLR mRNA were increased by hCG at 24 and 48 h, whereas RGT attenuated this induction in STAR and SCARB1 (but not LDLR) only at 48 h (Fig. 3, D–F).
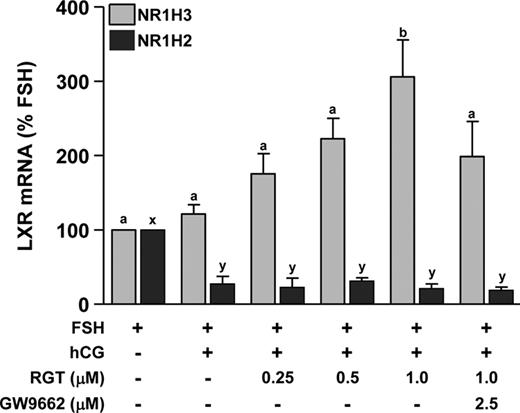
Effect of PPARG agonist treatment on NR1H2 and NR1H3 mRNA expression during in vitro luteinization. Granulosa cells isolated before hCG as in Fig. 1 were plated in fibronectin-coated 24-well plates and treated with FSH to maintain the nonluteinized phenotype or FSH+hCG for 24 h to induce luteinization. The PPARG agonist RGT (0–1.0 μm) was added to cultures with or without the PPARG antagonist GW9662 (2.5 μm). NR1H3 (gray bars) and NR1H2 (black bars) mRNAs were measured by real time RT-PCR. Data are presented as mean ± sem. Bars with different lowercase letters are significantly different (n = 3; P < 0.05).
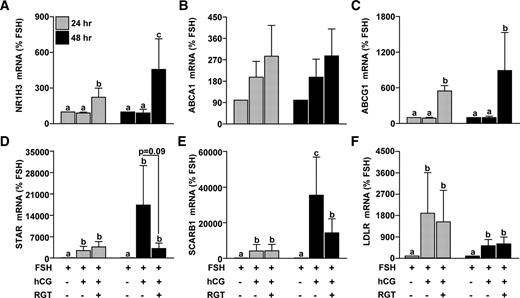
Effect of PPARG agonist treatment on NR1H3, ABCA1, ABCG1, STAR, and SCARB1 mRNA expression during in vitro luteinization. Granulosa cells isolated before hCG following a COS protocol were plated in a fibronectin-coated 24-well plate and treated with FSH or FSH+hCG with or without RGT (1.0 μm) for 24 or 48 h. The 48-h treatment group received an additional media change after the first 24 h of treatment. The mRNA expression of (A) NR1H3, (B) ABCA1, (C) ABCG1, (D) STAR, (E) SCARB1, and (F) LDLR was determined by real time RT-PCR as per Fig. 1. Data are presented as mean ± sem of percent change over time-matched FSH treatment. Bars with different lowercase letters are significantly different (n = 3; P < 0.05).
Effect of LXR agonist treatment on ABCA1, ABCG1 mRNA expression, and progesterone production
The role of NR1H2 and NR1H3 was determined using the LXR agonist T0901317. Nonluteinized granulosa cells were treated with FSH or FSH + hCG ± T0901317 (0–10 μm) for 24 or 48 h. Progesterone was induced (P < 0.05) after the addition of hCG at 24 and 48 h. The LXR agonist T0901317 attenuated the hCG-induced progesterone production starting at 5 μm (Fig. 4, A and D). T0901317 (1 μm) increased ABCA1 and ABCG1 mRNA at 24 and 48 h of treatment (Fig. 4, B, C, E, and F).
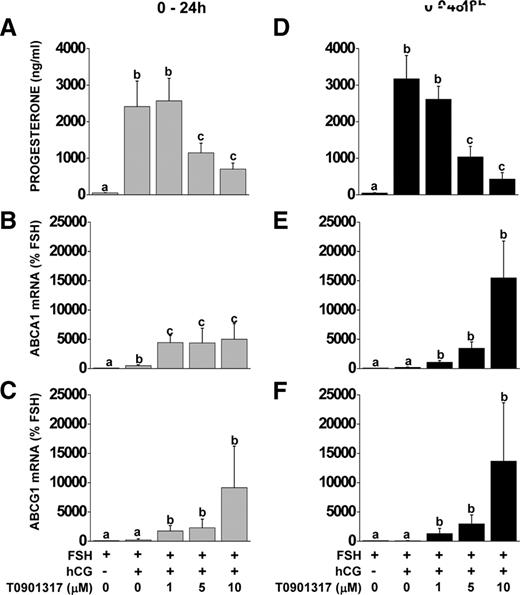
Effect of LXR agonist treatment on progesterone production and ABCA1 and ABCG1 mRNA expression during in vitro luteinization. Nonluteinized granulosa cells were isolated and treated with FSH or FSH+hCG with or without the LXR agonist T0901317 (0–10 μm) for 24 or 48 h. Progesterone concentrations in culture media were determined by RIA (A and D), ABCA1 (B and E) and ABCG1 (C and F) mRNA expression were measured by real-time RT-PCR. Data for mRNA expression are presented as mean ± sem of percent change over time-matched FSH treatment. Bars with different lowercase letters are significantly different (n = 3; P < 0.05).
Effect of LXR agonist treatment on steroidogenic gene expression
Because addition of LXR agonist in the presence of hCG reduced progesterone levels, additional relevant gene targets (STAR, CYP11A1, HSD3B, SCARB1, and LDLR) were measured after the addition of the LXR agonist T0901317. Addition of hCG increased the expression of all four genes at 24 h and STAR, CYP11A1, SCARB1, and LDLR at 48 h (Fig. 5). T0901317 reduced the expression of STAR (Fig. 5, A and F) and SCARB1 (Fig. 5, D and I) mRNA starting at 5 μm at 24 or 48 h of treatment. CYP11A1 mRNA expression was reduced at 24 h of treatment with 5 and 10 μm doses of T0901317 and was reduced to basal (FSH) levels by 48 h (Fig. 5, B and G). HSD3B mRNA levels were reduced in a dose-dependent manner after 24 h of treatment, while no effect was seen after 48 h of treatment (Fig. 5, C and H). LDLR was unaffected by T0901317 at 24 h, whereas there was a tendency (not significant) for T0901317 to reduce LDLR mRNA at 48 h.
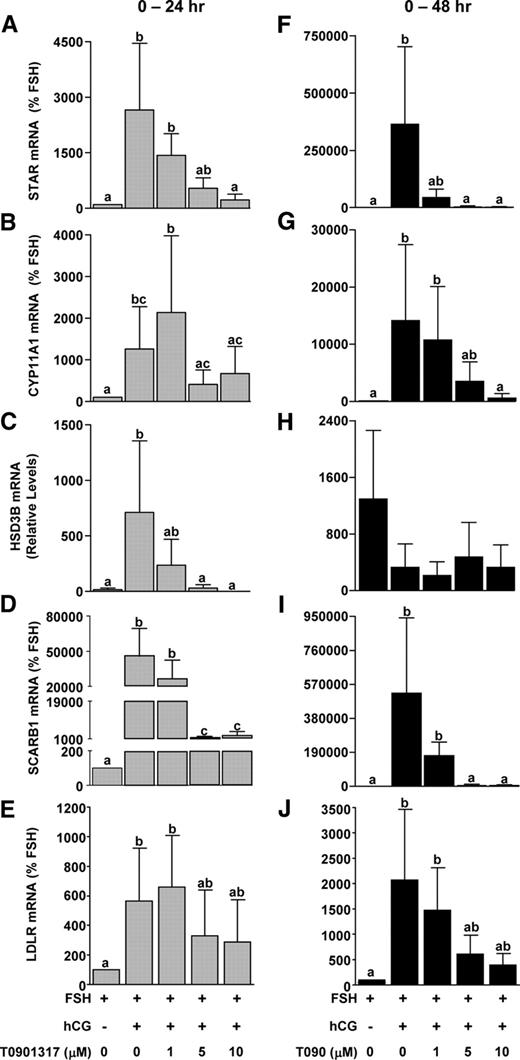
Effect of LXR agonist treatment on mRNA expression of steroidogenic genes during in vitro luteinization. Nonluteinized granulosa cells were isolated as and treated with FSH or FSH+hCG with or without the LXR agonist T0901317 (0–10 μm) for 24 or 48 h. The mRNA expression of STAR (A and F), CYP11A1 (B and G), HSD3B2 (C and H), SCARB1 (D and I), and LDLR (E and J) was determined by real time RT-PCR. Data are presented as mean ± sem of percent change over time-matched FSH treatment. Bars with different lowercase letters are significantly different (n = 3; P < 0.05).
Discussion
Progesterone production after an ovulatory stimulus is rapidly and dramatically induced such that the serum concentrations double within 30 min (24). One way by which the ovulatory LH surge ensures such a rapid increase in progesterone production is by increasing the expression of steroidogenic genes (3, 5, 25, 26). This increase in gene expression is mediated by several transcription factors, including steroidogenic factor-1, liver receptor homolog-1, cAMP response element-binding protein, and others (9). However, the role of transcription factors that regulate genes involved in cholesterol uptake and metabolism in the ovary has received relatively little attention; these include PPARG and liver X receptors (NR1H2, NR1H3) (10–12). PPARG, NR1H2, and NR1H3 are highly expressed before the ovulatory stimulus but are down-regulated within 3 h of an ovulatory stimulus. In vitro, PPARG promotes the expression of NR1H3, which in turn suppresses hCG-induced StAR, HSD3B, CYP11A, SCARB1, and progesterone while increasing mRNA levels of ABCA1 and ABCG1. Collectively, these data suggest that PPARG, NR1H2, and NR1H3 negatively regulate steroidogenesis and that hCG-induced down-regulation of these transcription factors is essential to maintain periovulatory steroidogenesis.
An ovulatory stimulus to macaques undergoing COS rapidly and profoundly reduces PPARG mRNA levels in luteinizing granulosa cells. Whereas PPARG mRNA in the rat ovary is also reduced after hCG, the changes in expression are less in magnitude and occur over a longer time frame (15, 27, 28). It is not currently clear how PPARG mRNA drops so quickly in macaque granulosa cells, although Jennewein et al. (29) showed that micro-RNA-27b destabilizes PPARG mRNA within 3 h in human macrophages. Interestingly, recent evidence indicates important changes in micro-RNA expression after an ovulatory stimulus in mice, and although miR-27b was not specifically noted, this model does provide an intriguing hypothesis to explain the rapid reduction in PPARG (30). Additional studies are clearly needed to elucidate the mechanisms leading to the rapid reduction in PPARG mRNA after an ovulatory stimulus.
PPARG appears to be a strong regulator of steroidogenesis. For example, a PPARG agonist promotes steroidogenesis by increasing STAR expression in cultured human granulosa luteal cells or MA-10 Leydig tumor cells (17, 18), whereas a similar treatment reduces progesterone synthesis via diminished HSD3B activity in porcine granulosa cells (19), suggesting context-specific activity. However, whether PPARG acts directly on genes such as STAR and HSD3B or through an intermediary is unclear. Possible PPARG targets are the liver X receptors, NR1H2 and NR1H3. A peroxisome proliferator response element is present in both the rat and human NR1H3 gene promoter (31, 32), and PPARG agonists increase the expression of NR1H3 in rat liver, human and murine macrophages, the leukemia cell line THP-1, and 3T3 preadipocytes (11, 32–34). Similarly, RGT increases the expression of NR1H3 mRNA, but not NR1H2, in luteinizing macaque granulosa cells, an effect reversed by concurrent treatment with the PPARG-specific antagonist GW9662. Because PPARG and NR1H3 are rapidly and coordinately down-regulated in vivo and activation of PPARG in vitro increases NR1H3 expression, we hypothesize that loss of PPARG expression after hCG leads to the specific loss in expression of NR1H3 whereas a different mechanism is required to reduce the mRNA expression of NR1H2.
NR1H2 and NR1H3 are intracellular cholesterol sensors that regulate an array of genes, including many that participate in cholesterol homeostasis (12). Among the main genes increased by the LXRs are those participating in reverse cholesterol transport such as ABCA1 and ABCG1 (members of the ABC family) (12). Levels of ABCA1 and ABCG1 decrease rapidly in response to an ovulatory stimulus, and a direct regulation of both genes by NR1H2/3 is evident in vitro, where an LXR agonist increases expression even in the presence of hCG. The high levels of ABCA1 and ABCG1 before hCG support the hypothesis that nonluteinized granulosa cells do not store cholesterol, whereas their down-regulation after hCG suggests that cholesterol is retained for progesterone production. ABCA1 and ABCG1 actively transport cholesterol to an APOA1 or APOE moiety of high-density lipoprotein; thus, the high levels of high-density lipoprotein in follicular fluid could derive, in part, from cholesterol effluxed by nonluteinized granulosa cells (35). LXR agonists promote cholesterol efflux and reduce progesterone synthesis by human granulosa luteal cells in culture (20) and block hCG-induced progesterone synthesis by macaque granulosa cells with a concurrent increase in ABCA1 and ABCG1 expression. Further, the decreased progesterone levels during the late luteal phase in rhesus macaques are associated with increased expression of NR1H3, ABCA1, and ABCG1 (21). Thus, decreased NR1H2 and NR1H3 lead to reduced ABCA1 and ABCG1, presumably preventing cholesterol efflux.
NR1H2 and NR1H3 are also known to regulate genes involved in steroid hormone biosynthesis (36, 37). In macaque granulosa cells, an LXR agonist attenuates the hCG-induced increase in STAR, CYP11A1, and HSD3B, as well as the lipoprotein receptor SCARB1. Drouineaud et al. (20) also showed a reduction in STAR and CYP11A1 but not SCARB1 in human luteinized granulosa cells treated with LXR agonist. During the latter stages of the primate luteal phase, there is reduced expression of STAR, CYP11A1, HSD3B, SCARB1, and LDLR and an associated increase in NR1H3 expression and transcriptional activity as evidenced by increase in ABCA1 (21). Whether LDLR is a target of NR1H2 and/or NR1H3 remains unclear; results from the current student are ambiguous, but do suggest an effect of the LXRs in maintaining (but possibly not inducing) LDLR expression. It is interesting that even after hCG, when NR1H2 and NR1H3 expression is quite low, an agonist still provokes marked changes in target gene expression, suggesting either that protein levels do not change as rapidly as mRNA or, more likely, that even low levels of NR1H2 and NR1H3 are incredibly strong regulators of gene expression. Thus, NR1H2 and NR1H3 repress the expression of steroidogenic genes in the primate ovary; the reduction in NR1H2 and NR1H3 via hCG-mediated reduction in PPARG thus derepress genes leading to increased steroidogenesis, including STAR, CYP11A1, HSD3B, SCARB1, and possibly LDLR.
In conclusion, data from the current study suggest that in the primate, nonluteinized granulosa cells have gene expression that is consistent with cholesterol efflux (e.g. high levels of ABCA1). An ovulatory stimulus reverses this and promotes the expression of genes involved in cholesterol uptake and metabolism (e.g. SCARB1). Because nonluteinized primate granulosa cells have rather high levels of HSD3B activity (24), this may represent a mechanism to limit preovulatory progesterone production by maintaining available cholesterol at low levels. The data also suggest that hCG-represses PPARG, which in turn leads to the loss of NR1H3 expression (and NR1H2 expression in a PPARG-independent manner). The reduction in NR1H2 and NR1H3 appears to derepress key periovulatory genes such as SCARB1 and STAR. Thus PPARG, NR1H2, and NR1H3 are very early and central regulators of periovulatory processes in luteinizing primate follicle.
Acknowledgments
We thank Organon, Inc. (West Orange, NJ) for the generous supply of recombinant human FSH and Serono Laboratories (Ares Advanced Technology, Randolph, MA) for the gift of recombinant hCG. We are grateful to Dr. Rebecca Brogan (Loyola University Maryland, Baltimore, MD) and Dr. Kavita Bhalla (Greenbaum Cancer Center, University of Maryland School of Medicine, Baltimore, MD) for technical assistance.
This research was supported, in part, by National Institutes of Health Grants HD043358 (to C.L.C.), RR13439 (to C.A.V.), and RR00169 (to California National Primate Research Center).
Disclosure Summary: The authors have nothing to declare.
Abbreviations
- ABC
ATP-binding cassette
- COS
controlled ovarian stimulation
- CYP11A1
P450 side-chain cleavage
- hCG
human chorionic gonadotropin
- LDLR
low-density lipoprotein receptor
- LXR
liver X receptors
- NR
nuclear receptor
- PPARG
peroxisome proliferator-activated receptor-γ
- PVA
polyvinyl acetate
- RGT
rosiglitazone
- SCARB1
scavenger receptor-BI
- STAR
steroidogenic acute regulatory protein
- TL
Tyrode’s lactate