-
PDF
- Split View
-
Views
-
Cite
Cite
Wesley W. Weathers, Charisse L. Davidson, Martin L. Morton, Energetics of Altricial Nestlings in Cold Climates: Insights from the Mountain White-Crowned Sparrow, The Condor: Ornithological Applications, Volume 105, Issue 4, 1 November 2003, Pages 707–718, https://doi.org/10.1093/condor/105.4.707
- Share Icon Share
Abstract
We determined the energy budget of nestling Mountain White-crowned Sparrows (Zonotrichia leucophrys oriantha) at a 2900-m-high subalpine site by combining growth data with measurements of field metabolic rate (doubly labeled water technique) and resting metabolic rate made on different-aged nestlings. Nestling sparrows grow rapidly (their logistic growth rate constant is 129% of the allometric prediction) and they fledge at 9 days of age weighing 20.6 g (75% of adult mass). Relatively rapid growth in a cool montane environment (mean daytime air temperature = 16.5 ± 1.4°C) is associated with high daily and total nestling energy requirements. During the 9 days between hatching and fledging, each nestling metabolized a total of 443 kJ of energy; a value 25% higher than expected for an open-nesting passerine bird. The relative cost of producing a fledgling White-crowned Sparrow (21.5 kJ per g body mass) exceeds that of the three other open-nesting passerine species that have been measured with doubly labeled water (range 16.5–19.3 kJ g−1). The energy that nestling sparrows accumulated as new tissue (115 kJ) constituted 26% of the total energy metabolized; substantially less than the 37% allocated to activity and thermoregulation combined. Nestling White-crowned Sparrows allocated more energy to activity and thermoregulation than nestlings of most other parent-fed species, but much less than the 50–53% of total metabolizable energy allocated by precocial shorebird chicks.
La Energética de Pichones Altriciales en Climas Fríos: Lecciones de Zonotrichia leucophrys oriantha
Resumen. Determinamos el presupuesto energético de pichones de Zonotrichia leucophrys oriantha en un sitio subalpino a 2900 m de altitud combinando datos de crecimiento y de tasa metabólica en el campo (medida con la técnica de agua doblemente marcada) y tasa metabólica en reposo en pichones de diferentes edades. Los pichones crecen rápidamente (sus tasas de crecimiento logístico son el 129% de las tasas alométricas) y empluman a los 9 días de edad, pesando 20.6 g (75% de su peso adulto). El crecimiento relativamente rápido en un ambiente de montaña fresco (temperatura diaria promedio = 16.5 ± 1.4°C) está asociado con altas exigencias energéticas diarias y totales. Durante los 9 días entre la eclosión y el emplumamiento, cada pichón metabolizó un total de 443 kJ de energía, un valor 25% más alto del esperado para un ave paserina de nido abierto. El costo relativo de producir un volantón de Zonotrichia leucophrys oriantha (21.5 kJ por g de peso corporal) es más alto que el de otras tres especies de paserinos de nido abierto que se han estudiado con la técnica de agua doblemente marcada (rango 16.5 a 19.3 kJ g−1). La energía que los pichones acumularon como tejido nuevo (115 kJ) representó el 26% de su metabolismo total, un valor sustancialmente menor que el 37% asignado a la actividad y a la termorregulación combinadas. Los pichones de Z. leucophrys oriantha asignaron más energía a la actividad y a la termorregulación que los pichones de la mayoría de las demás especies alimentadas por los padres, pero menos que los pichones precoces de aves playeras (50 a 53%).
Introduction
One of the main evolutionary options birds have for balancing food supply and production is to vary nestling growth rate (Ricklefs et al. 1998). The energetic consequence of growth rate variation depends, however, upon whether one considers the total energy metabolized during growth or the energy metabolized per day. In general, a reciprocal relationship exists between daily and total metabolized energy, such that nestlings that spend longer in the nest than predicted from their mass have lower daily but higher total energy requirements than nestlings that fledge sooner (Weathers 1992, 1996). Lengthening the nestling period decreases the amount of food that parents must provide their nestlings on a daily basis, but increases the total energy required per offspring. This reciprocal relation between growth rate and energy demand yields a remarkable symmetry. Time to fledging and fledging mass explain 97–99% of the interspecific variation in both total metabolizable energy (TME) and peak daily metabolizable energy (DME) requirement in nestlings of 30 species, with an average variation in TME from predicted of only ±14% (Weathers 1992). Although Weathers' analysis permits predictions, it provides only limited insight into adaptive radiation of postnatal energetics because many of the species included are from the temperate zone and 28 of the 30 species produce nestlings that remain in the nest and are fed by their parents. Furthermore, it is unclear from Weathers' analysis whether an explicit link exists between fast growth and a higher than predicted TME, although such a circumstance is implied by his figure 6.
Growth rate is not the only variable that may affect total metabolizable energy. In two self-feeding precocial shorebird species, the Northern Lapwing (Vanellus vanellus) and Black-tailed Godwit (Limosa limosa), total metabolizable energy is 29% and 39% higher than predicted allometrically. Schekkerman and Visser (2001) attribute these species' high energy demand to the cost of activity and thermoregulation, which combined accounted for 50–53% of their TME. In contrast with shorebirds, nestlings in a group of seven parent-fed species allocated only 19 ± 6% (range 12–30%) of their TME to activity plus thermoregulation (Schekkerman and Visser 2001). Does precocial development thus represent a fundamentally different energy paradigm, or might altricial nestlings from cold environments have similarly high thermoregulatory costs? To examine this question, we used the doubly labeled water (DLW) technique to measure daily metabolizable energy of nestlings in a population of White-crowned Sparrows (Zonotrichia leucophrys oriantha) that breeds in a climatically harsh 2900-m-high subalpine meadow. We also measured nestling resting metabolic rates (RMR) and used those data, together with previous determinations of growth rate (Morton et al. 1972), to construct nestling energy budgets and calculate the energy allocated to growth, activity, and thermoregulation. Additionally, we used data of Hodum and Weathers (2003) to calculate activity plus thermoregulatory costs for nestlings of four species of Antarctic fulmarine petrels that grow twice as fast as predicted allometrically, despite encountering the coldest conditions endured by any growing bird (air temperatures as low as −25°C, Bech et al. 1988). Our data reveal that these cold-climate species incur higher activity–thermoregulatory costs than many parent-fed species, but still allocate less of their total metabolizable energy (30 ± 10%) to this category than the precocial shorebirds studied by Schekkerman and Visser (2001).
Methods
Study Site and Species
We studied nestling energetics in a population of Mountain White-crowned Sparrows that has been under continuous study since 1968 (for detailed life history and site description, see Morton 2002). The study site, a 2900-m-high subalpine meadow near Tioga Pass (38°55′N, 119°16′W) Mono County, California, is climatically harsh and unpredictable. Late spring storms with high winds, subfreezing temperatures, and snow often delay or temporarily disrupt breeding. Even during more temperate periods, adults and young often encounter freezing temperatures, especially at night. At this site, breeding usually lasts from late May though early August. Broods typically consist of 3–4 young, and nests are often located on the ground in willow thickets (Salix sp.) or above ground in the branches of small pines (Pinus sp.). Nestlings spend 9–10 days in the nest and are fed by both parents. Young are brooded continuously by the female at night until they fledge and for about 50% of the daylight hours during the first three days after hatching. Thereafter, daytime brooding drops off rapidly, reaching about 14% of the day by 6 days of age (Morton 2002).
Meteorology
We monitored the nestlings' thermal environment during determinations of their field metabolic rate (FMR, see Appendix for abbreviations and symbols) (July 1994) by recording the following parameters with a centrally placed weather station: air temperature (Ta; shaded 36-gauge type-T thermocouple), operative temperature (Te) 0.5 m above ground inside a willow thicket (3.5-cm-diameter metal-sphere thermometer painted flat gray, Bakken et al. 1985, Walsberg and Weathers 1986), windspeed (u) 1 m above the ground in the open (Thornthwaite model 901 cup anemometer, Elmer, New Jersey) and 0.5 m above ground inside a willow thicket (hotball anemometer; Roer and Kjölsvik 1973). We also recorded ground-level solar radiation with a Li-Cor model 200 pyranometer (Lincoln, Nebraska). Sensor outputs were assessed every 60 sec, averaged every 30 min, and recorded with a Campbell Scientific 21X data logger (Logan, Utah). Because we were interested in the potential effect of weather on nestling energy requirements, we evaluated meteorological conditions by calculating mean half-hour values (weighted for the number of nestlings measured per day) over the 10 days that FMR was measured. The cup-anemometer and pyranometer were factory calibrated and the various thermocouples were calibrated against a National Bureau of Standards certified mercury thermometer. We calibrated the hotball anemometer in a large laminar-flow wind tunnel at the UC Davis hydraulics laboratory against a HanDar 2-dimensional sonic anemometer (Sunnyvale, California) and a Campbell CSAT 3-dimensional anemometer, both previously calibrated against primary standards.
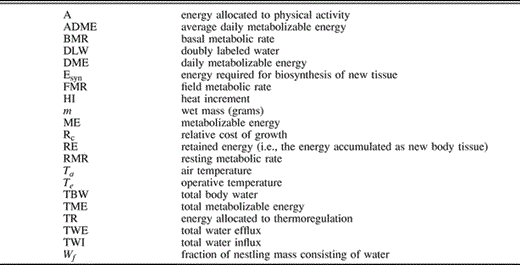
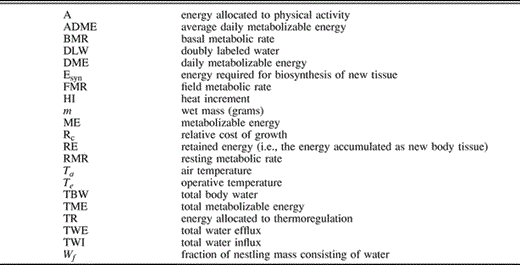
Field Metabolic Rate
We determined FMR of 35 nestlings during July 1994 using the doubly labeled water (DLW) technique. Nestlings were removed from their nest, weighed to the nearest 0.05 g (K-TRON electronic balance, Sunnyvale, California), and given a subcutaneous injection of 20–40 μL of water (depending upon nestling mass) that contained 97 atom percent 18O and ca. 0.2–0.46 MBq 3H. After injection, nestlings were returned to their nest for 60 min to allow the DLW to equilibrate with body water. We then reweighed each nestling and drew a 50–70 μL blood sample from the brachial vein before returning the nestling to its nest. Twenty-four hours later, nestlings were again removed from the nest, weighed, and a second 50–70 μL blood sample drawn. Hematocrit tubes were flame-sealed and stored at 4°C until microdistilled to obtain pure water, which was assayed for tritium activity (duplicate samples in toluene-Triton X100-PPO scintillation cocktail, Packard model 1600 CA liquid scintillation counter, Meriden, Connecticut) and for 18O content (triplicate samples) by cyclotron-generated proton activation of 18O to fluorine-18 with subsequent counting of the positron-emitting 18F in a Packard model 5210 gamma counter (Wood et al. 1975). Background 18O level of three uninjected nestlings averaged 0.2002 ± 0.0006 atom percent.
We calculated body water volumes, rates of CO2 production, and water efflux using the equations of Nagy (1980, 1983) and Nagy and Costa (1980). Recent validation studies (Visser and Schekkerman 1999, Visser et al. 2000) found that these equations (which are single-pool models and assume no evaporative water loss) overestimate CO2 production by about 5–7% in precocial chicks. These authors recommend calculating CO2 production using equation 7.17 of Speakman (1997). It is unclear whether Speakman's equation applies to altricial chicks, however, and the proportion of water loss occurring evaporatively in altricial chicks is likewise unknown. Accordingly, we adopted a conservative approach and used the traditional equations to calculate CO2 production and water efflux. We calculated nestling field metabolic rate from CO2 production assuming 26.2 kJ per L CO2 (Weathers and Sullivan 1989). Initial isotope samples of three nestlings were lost during processing and their CO2 production was calculated by the single-sample technique (Webster and Weathers 1989). Water flux determined by the single-sample method is less reliable than flux determined by the double-sample method and we excluded flux values for these three nestlings from subsequent analyses.
Resting Metabolic Rate
Between 30 June and 7 July 1993, we measured O2 consumption (VO2) of twenty-five 2- to 8-day-old nestlings during both the day (10:30–15:00) and night (22:00–01:00) with a closed-circuit respiratory system. Six of the 25 nestlings were measured at both times of day. We transported nestlings two at a time from the field to a research site located about 1 mile away (Tioga Pass Lodge) and placed them in an incubator at 36–37°C. After a 30-min acclimation period, we weighed the nestlings and syringe-fed them with Gerber's® baby cereal (7% protein, 7% fat, 86% carbohydrate) until their crops were bulging. We then placed the nestlings in a respirometry system consisting of three airtight 400-mL glass chambers painted flat black. Each chamber was equipped with a small, felt-lined nest cup (suspended above a 60-mL 10% NaOH solution), a glass manometer, and a flush valve. One chamber contained an artificial chick (to adjust for the volume occupied by the live chick) and served as a thermobarometer to correct for changes in temperature and atmospheric pressure. The chambers were submerged in a water bath at 36–37°C to maintain nestlings within their thermoneutral zone. Once submerged, the system was allowed to equilibrate for 30 min with the chambers open to the atmosphere. The chambers were then flushed with O2, closed, and the time required to consume 0.5 to 2 mL of O2 recorded. This process was repeated a minimum of four times to establish each chick's average rate of oxygen consumption. Atmospheric pressure was measured with a precision aneroid barometer (Tayama & Co., Ltd., Tokyo) and VO2 corrected to standard temperature and pressure of dry gas. Once the measurements were completed, nestlings were either fed and returned to their nests or held for nighttime measurements. Nighttime VO2 measurements followed a similar protocol except that nestlings fasted for a minimum of 2 hr before we placed them in the metabolic chamber. We calculated metabolic rates assuming an energy equivalent of 20.1 kJ per L O2 for fasted nestlings and 19.8 kJ per L O2 for fed nestlings (Blaxter 1989).
Nestling Energy Budget
To evaluate whether self-feeding chicks allocate more energy to activity plus thermoregulation than parent-fed chicks, we assembled published data and calculated combined activity plus thermoregulatory costs for nestlings of four Antarctic fulmarine petrel species using data of Hodum and Weathers (2003) and recalculated data for nestling Acorn Woodpeckers (Melanerpes formicivorus; Weathers et al. 1990) and four species of nestling terns (Klaassen 1994) by adjusting the original RMR measurements, which were made on fasted birds, to their fed equivalents assuming HI increases RMR by 30% during the daytime, which was 14 hr for Acorn Woodpeckers, 24 hr for Antarctic Terns (Sterna vittata) and Arctic Terns (Sterna paradisaea) on Spitsbergen, and 17 hr for Arctic and Common Terns (Sterna hirundo) in the Netherlands. Because all these studies used DLW to measure FMR, the energy allocated to activity plus thermoregulation was calculated as FMR − RMR for each species.
Statistical Analyses
All statistical analyses were performed using SAS (SAS Institute Inc. 2000). We used least-squares regression to characterize the relationship between nestling body mass and energy metabolism (FMR and RMR), total body water (TBW), the fraction of nestling mass consisting of water (Wf), and water flux. We compared the relation of RMR to nestling mass during rest and active phases using ANCOVA and paired two-tailed t-tests. Relationships between bivariate data sets were determined using product-moment correlation. Except where indicated, values are presented as means ± SD.
Results
Meteorology
Wide year-to-year variation characterizes weather in the Sierra Nevada mountains (Morton 2002) and although long-term weather data are lacking for our study site, air temperature data for a comparable montane site located 40 km northeast of Tioga Pass (Bodie, California; 2538 m elevation) support our impression that July 1994 was relatively warm. Maximum daily July shade air temperature at Bodie averaged 25.2 ± 1.6°C (range 23.2–27.6°C) for the period 1985–1997 (NOAA 2002). Mean shade air temperature at Bodie for July 1994 (27.4°C) was the second warmest for the 13-year period and fell outside the 95% confidence interval of the long-term mean (24.3–26.2°C).
Despite July 1994 being relatively warm, the high Sierra is still a cold and windy place. Minimum nighttime Ta at our study site during DLW measurements ranged from −1.2°C to 6.3°C (mean 2.3 ± 2.1°C) and maximum daytime Ta ranged from 19.1°C to 25.3°C (mean 23.2 ± 1.8°C). Mean 24-hr shade Ta during FMR measurements averaged 12.5 ± 1.4°C (range 9.6–14.5°C), whereas daytime shade Ta averaged 16.5 ± 1.4°C (range 13.8–18.8°C).
Both shade Ta in the open and Te measured inside a willow thicket increased rapidly following sunrise, contributing to a marked daily temperature cycle (Fig. 1). Solar radiation is intense at high altitude, and generally clear skies at Tioga Pass during our study contributed to the spike in daytime temperature. Midday irradiance (12:00–14:00) averaged 1037 ± 19 W m−2. Windspeed measured 1 m above ground in the open also exhibited a daily cycle, and tended to be higher during the daylight hours (Fig. 1). Nestlings ensconced in their nests would have experienced lower windspeeds than those measured in the open, however. Windspeed measured 0.5 m above ground inside a willow thicket, which would more closely approximate that encountered by nestlings, averaged 10 ± 0.2% of that in the open.
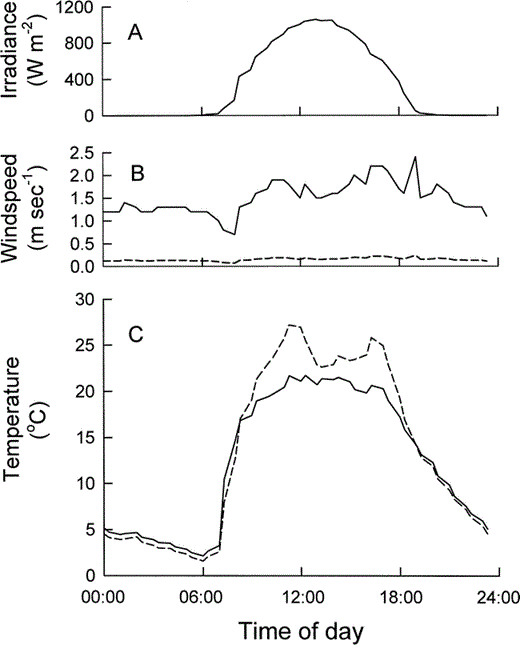
Mean weather conditions at Tioga Pass Meadow, California, July 1994, when field metabolic rates of nestling Mountain White-crowned Sparrows were determined with doubly labeled water. (A) Solar irradiance, (B) windspeed measured 1 m above ground in the open (solid line), and 0.5 m above ground inside a willow thicket (dashed line), (C) operative temperature measured 0.5 m above ground inside a willow thicket (dashed line), and shade air temperature measured 1 m above ground (solid line).
Doubly Labeled Water
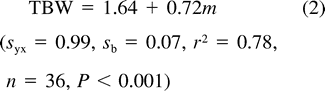
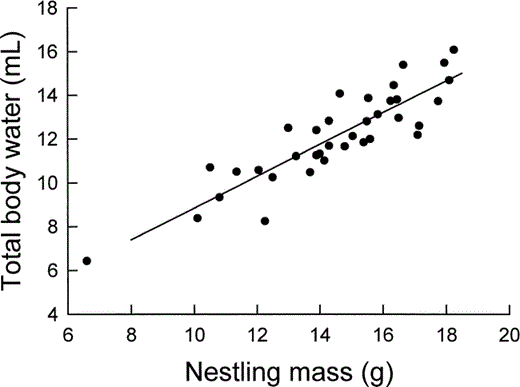
Relationship between the total body water content of nestling Mountain White-crowned Sparrows, as determined by 18O dilution, and nestling body mass. The least-squares regression line is described by text equation 2.
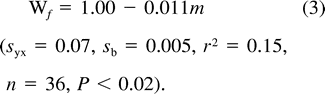
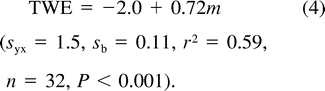
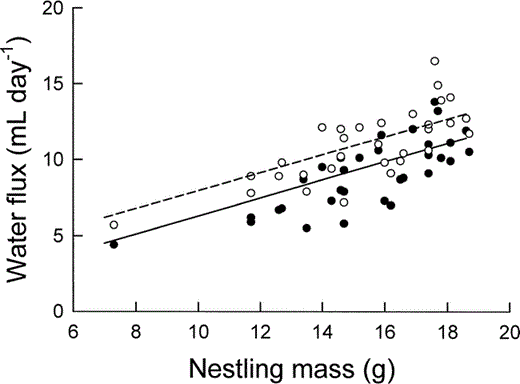
The relationship between water influx (unfilled circles and dashed line) and water efflux (filled circles and solid line) of nestling Mountain White-crowned Sparrows, as determined by doubly labeled water, and nestling body mass. The least-squares regression lines are described by text equations 4 and 5.
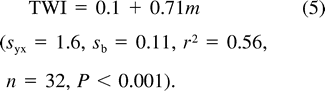
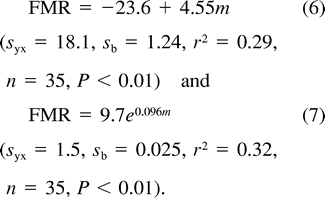
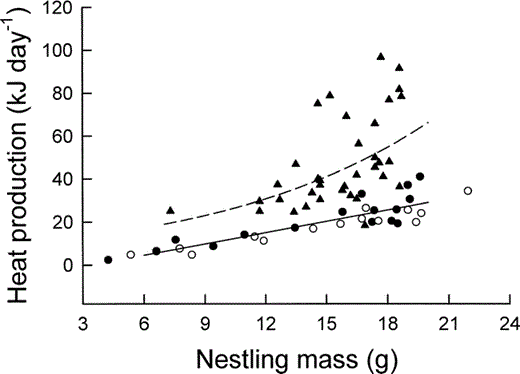
Relationship between nestling Mountain White-crowned Sparrow field metabolic rate (triangles and dashed line) and resting metabolic rate (solid line) measured during the daytime (filled circles) and nighttime (unfilled circles) and nestling body mass. Least-squares regression lines described by text equations 7 and 8
Resting Metabolic Rate
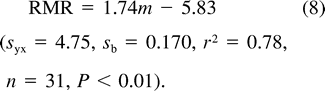
Nestling Energy Budget
In nestling Mountain White-crowned Sparrows, DME increased sigmoidally with age (Fig. 5), reaching 97% of its maximum value (68.8 kJ day−1) by 6 days of age (Table 1). Summing DME over the nestling period yields the total energy metabolized by nestlings. Most Mountain White-crowned Sparrows fledge at 9 days of age, although the last-hatched chick often fledges at 8 days of age and a few chicks fledge on day 10 (Morton 2002). For nestlings that fledge in 9 days, TME is 443 kJ (Table 1). Of this total, RMR comprises about 38%, whereas activity and thermoregulation combined account for about 37%. The proportion of TME attributable to thermoregulation and activity increased throughout the nestling period, as is apparent from the divergence with age of the lines for RMR and FMR in Figure 5, and is higher than that of most other parent-fed nestlings (Table 2).
Calculation of the daily metabolizable energy (DME) of a nestling Mountain White-crowned Sparrow based on values for carcass energy content (EC), energy retained as new tissue (RE), laboratory resting metabolic rate (RMR, O2 consumption), and field metabolic rate (FMR, doubly labeled water technique) derived from regression equations fitted to measurements of different-aged nestlings measured at Tioga Pass Meadow, California

Calculation of the daily metabolizable energy (DME) of a nestling Mountain White-crowned Sparrow based on values for carcass energy content (EC), energy retained as new tissue (RE), laboratory resting metabolic rate (RMR, O2 consumption), and field metabolic rate (FMR, doubly labeled water technique) derived from regression equations fitted to measurements of different-aged nestlings measured at Tioga Pass Meadow, California

Proportion of the total metabolizable energy (TME) between hatching and fledging that is allocated to thermoregulation plus physical activity (TR + A) in 13 species. Within each category, species are arranged in order of ascending mass
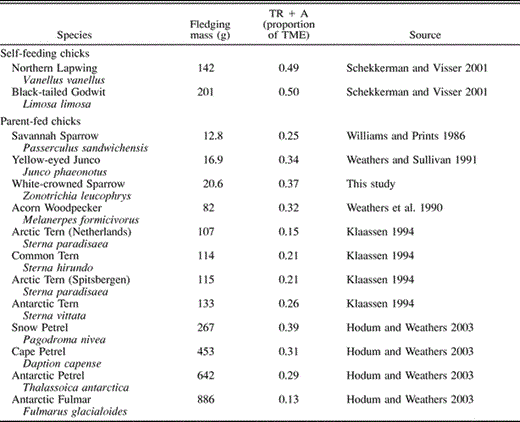
Proportion of the total metabolizable energy (TME) between hatching and fledging that is allocated to thermoregulation plus physical activity (TR + A) in 13 species. Within each category, species are arranged in order of ascending mass
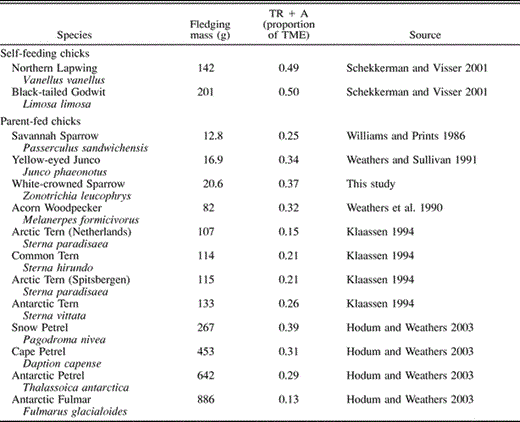
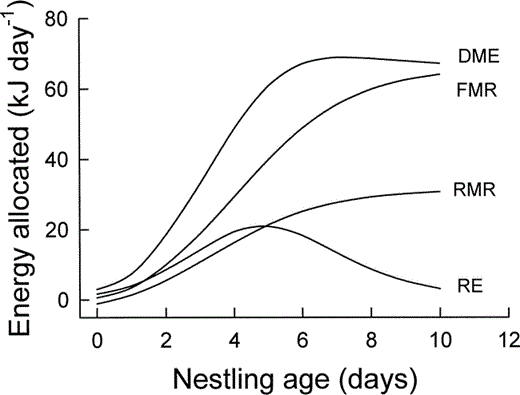
Energy expenditure of nestling Mountain White-crowned Sparrows as a function of age. RE = energy retained as new tissue, RMR = resting metabolic rate, FMR = field metabolic rate, and DME = daily metabolizable energy
Discussion
The total energy metabolized by nestling White-crowned Sparrows is 6% greater than predicted for birds in general based on age and body mass at fledging (Weathers 1992). Although the agreement is close, a more meaningful comparison is with the TME of other temperate-zone open-nesting passerine birds. Such a comparison (Table 3) reveals the White-crowned Sparrow's TME to be relatively high (a circumstance that also applies to the average daily rate at which it metabolized energy). The life-history or environmental correlates that underlie the relatively high postnatal energy requirement are unclear, but one potential factor is the White-crowned Sparrow's cool montane environment. Mean shade air temperature during doubly labeled water measurements of nestling sparrows averaged 12.5 ± 1.4°C, well below the 23°C lower critical temperature of adult sparrows (King 1964). A cool environment presumably contributed to nestling sparrows allocating 37% of their TME to activity plus thermoregulation. Yet data for nestling Yellow-eyed Juncos (Junco phaeonotus) from a montane (2560 m elevation) site in Arizona's Chiricahua Mountains (Weathers and Sullivan 1991) suggest that other factors are involved in the Mountain White-crowned Sparrow's relatively high TME. Nestling juncos encounter similarly cool temperatures (mean shade Ta = 16.9 ± 1.1°C) and allocate 34% of their TME to thermoregulation and activity, yet they do not possess a higher average daily metabolizable energy (ADME) or TME than other open-nesting passerines (Table 3). Differences in growth rates may underlie this seeming paradox. The logistic growth rate constant of Mountain White-crowned Sparrows (k = 0.606) is 129% of the value predicted for an altricial bird of 21.3 g asymptotic mass (Ricklefs 1968), whereas the growth rate constant of juncos (k = 0.471) is 96% of predicted (Weathers and Sullivan 1991). Thus when differences in body size are accounted for, Mountain White-crowned Sparrows grow 33% faster than juncos.
Body mass (derived from logistic growth equations) and age at fledgling, total metabolizable energy (TME), and average daily metabolizable energy (ADME) of temperate-zone, open-nesting passerines. Value in parentheses after mass is fledgling mass as a percentage of adult mass. Values in parentheses for Age, TME, and ADME denote percentage of that variable's predicted value, based respectively on equations 6, 8, and 9 of Weathers (1992)
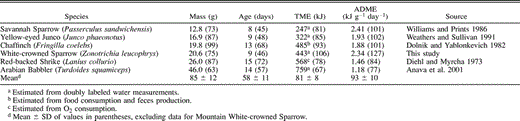
Body mass (derived from logistic growth equations) and age at fledgling, total metabolizable energy (TME), and average daily metabolizable energy (ADME) of temperate-zone, open-nesting passerines. Value in parentheses after mass is fledgling mass as a percentage of adult mass. Values in parentheses for Age, TME, and ADME denote percentage of that variable's predicted value, based respectively on equations 6, 8, and 9 of Weathers (1992)
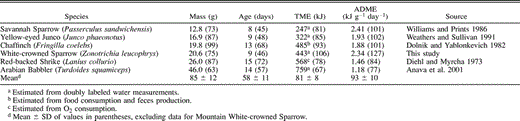
Schekkerman and Visser (2001) derived energy budgets for Northern Lapwings and Black-tailed Godwits based on doubly labeled water measurements of FMR and laboratory RMR determinations, methods comparable to ours. They concluded that these self-feeding precocial chicks have high energy requirements (TMEs were 29–39% higher than predicted), compared with parent-fed species, due to the high cost of activity and thermoregulation while foraging. For their shorebird chicks, activity plus thermoregulation accounted for 50–53% of TME, more than twice as much as in seven species of parent-fed chick also measured with doubly labeled water. On average, the 12 parent-fed nestlings in Table 2 allocated 0.27 ± 0.08 of their TME to thermoregulation and activity combined. Chicks of 5 of the 11 parent-fed species (45%) allocated over 30% of their TME to activity and thermoregulation (range 31–39%), yet all are well below the 50–53% allocated by self-feeding chicks (Table 2). The data, therefore, support Schekkerman and Visser's (2001) conclusion that the TME of self-feeding shorebird chicks is high due to the high cost of activity and thermoregulation while foraging. The Table 2 species are not representative of birds in general, however, as most are from high-altitude or -latitude study sites where thermoregulatory costs should be elevated. Additional data are needed on both parent-fed and self-feeding nestlings from temperate or even tropical habitats, where thermoregulatory costs are lower, to evaluate whether precocial self-feeding young represent a fundamentally different energy paradigm than parent-fed modes of development.
Interspecific comparisons of postnatal metabolizable energy requirements can be confounded by differences in the age at which various ontogenetic milestones occur across species. Two commonly used milestones are the nestling period, denoted by the interval between hatching and leaving the nest, and the fledging period, the interval between hatching and flight (Skutch 1976). Some altricial species fly when they leave the nest, whereas others do not, and thus attain fledging status later. When Mountain White-crowned Sparrow chicks leave the nest at 9 days of age they weigh only 75% of mean adult mass (27.4 g, n = 3775 measurements during June and July; Morton 2002) and are unable to fly. They do not attain adult mass until they are 30 days old (Morton et al. 1972). Clearly, calculating postnatal energy requirements over the period from hatching to attaining flight would yield a much higher TME for Mountain White-crowned Sparrows and would increase the proportion of TME allocated to activity and thermoregulation. Whether the gap in the proportion of TME allocated to thermoregulation and activity in shorebird versus Mountain White-crowned Sparrow chicks would close is unclear.
Data for Antarctic fulmarine petrels (Table 2) suggest that body size influences activity and thermoregulatory costs. Petrel chicks are inactive in the nest and most of the energy they allocate to the activity + thermoregulation category presumably represents thermoregulation. Because the four petrel species were studied at the same time and site (Hop Island, East Antarctica), and thus encountered similar thermal environments, one might expect the larger chicks to allocate less energy to thermoregulation. The proportion of TME that petrel chicks allocated to thermoregulation and activity was negatively correlated with body size (r2 = −0.97, P = 0.04); the smallest species allocated 39% of its TME to activity + thermoregulation, the largest just 13%.
The relatively high TME of Antarctic petrel chicks is associated with fast growth in a cold environment and is made possible by abundant food during the Antarctic summer. Might abundant food likewise support fast growth and high TME in nestling Mountain White-crowned Sparrows? Several considerations support this possibility. There is a rich pulse of plant and insect food during the brief alpine summer (Morton 2002) and adult White-crowned Sparrows at our montane study site rear larger broods (3.7 versus 2.9 nestlings) than adults that breed at a warmer coastal site (Weathers et al. 2002). Furthermore, although adult Mountain White-crowned Sparrows have higher FMRs when feeding nestlings than coastal adults, adults of neither population work to their physiological capacity when feeding young (their field metabolic rate is only 2.1 to 2.6 times basal metabolic rate, Weathers et al. 2002), implying that food is not generally limited. Clearly, factors such as predation risk may affect nestling growth rate and energy demand, but ultimately food energy determines the physiological boundaries within which species operate.
Early analyses of avian postnatal energy requirements emphasized the importance of growth and focused on the energy required to produce new tissue (Drent and Daan 1980, O'Connor 1984). Yet because only 13–28% of the total energy metabolized by growing birds is deposited as new tissue, postnatal energy requirements will be determined mainly by basal metabolism, thermoregulation, and physical activity (Wijnandts 1984, Drent et al. 1992, Weathers 1996). Indeed, nestling Mountain White-crowned Sparrows allocate substantially less of their TME to the production of new tissue (26%) than to activity and thermoregulation (37%). Clearly, although variation in nestling growth rate is one evolutionary option for balancing food supply and production (Ricklefs et al. 1998), factors that affect thermoregulatory costs, such as nest-site placement or nest insulation, may be energetically more important. As interest shifts away from growth per se to a partitioning of metabolizable energy into its components, studies of precocial species and parent-fed species from temperate and tropical habitats will be especially illuminating.
Acknowledgments
White-crowned Sparrows were captured and metabolic rates in captivity determined under authority of U.S. Department of Interior Fish and Wildlife Service permit nos. 9316 and 8400 and University of California Animal Use and Care Protocol No. 3607. Field metabolic rate measurements using tritiated water were authorized by University of California Radiation Use Authorization No. 0942, State of California Department of Health Services Radioactive Material License No.1334-57, and U.S. Forest Service Special Use Permit No. 2720, Inyo National Forest. We thank Rodney Siegel for field assistance, Mitch Allen for helping calibrate hotball anemometers, Thomas Famula for statistical advice, and Robert Sainz for preparing our Spanish abstract. This study was supported by grant 5598–95 from the National Geographic Society.
Literature Cited