-
PDF
- Split View
-
Views
-
Cite
Cite
Lukas Hensel, Jana Lüdtke, Katia O Brouzou, Simon B Eickhoff, Daniel Kamp, Leonhard Schilbach, Noninvasive brain stimulation in autism: review and outlook for personalized interventions in adult patients, Cerebral Cortex, Volume 34, Issue 13, May 2024, Pages 8–18, https://doi.org/10.1093/cercor/bhae096
- Share Icon Share
Abstract
Noninvasive brain stimulation (NIBS) has been increasingly investigated during the last decade as a treatment option for persons with autism spectrum disorder (ASD). Yet, previous studies did not reach a consensus on a superior treatment protocol or stimulation target. Persons with ASD often suffer from social isolation and high rates of unemployment, arising from difficulties in social interaction. ASD involves multiple neural systems involved in perception, language, and cognition, and the underlying brain networks of these functional domains have been well documented. Aiming to provide an overview of NIBS effects when targeting these neural systems in late adolescent and adult ASD, we conducted a systematic search of the literature starting at 631 non-duplicate publications, leading to six studies corresponding with inclusion and exclusion criteria. We discuss these studies regarding their treatment rationale and the accordingly chosen methodological setup. The results of these studies vary, while methodological advances may allow to explain some of the variability. Based on these insights, we discuss strategies for future clinical trials to personalize the selection of brain stimulation targets taking into account intersubject variability of brain anatomy as well as function.
Introduction
Autism spectrum disorder (ASD) is a pervasive developmental disorder characterized by impairments of social interaction and communication as well as stereotyped, repetitive behavior. Patients often present with attentional, mnestic, empathetic, cognitive, and social cognitive deficits (American Psychiatric Association 2013; World Health Organization 2022). There is currently no one standard treatment for ASD, but a range of options that differs according to age and in light of intellectual abilities. More recently, noninvasive brain stimulation (NIBS) techniques have been proposed as a potential treatment option. The increasing knowledge about the brain networks that underlie social cognition and social interaction (Redcay and Schilbach 2019) gives rise to new opportunities to modulate pathophysiological mechanisms involved in ASD to achieve a favorable outcome.
An increasing number of laboratories have begun to examine the effects of NIBS in patients with ASD using different stimulation methods and protocols (Oberman et al. 2015; Oberman, Enticott, et al. 2016a; Khaleghi et al. 2020). These studies either used transcranial direct current stimulation (tDCS) or repetitive transcranial magnetic stimulation (rTMS) to stimulate brain tissue. While rTMS induces axonal currents via magnetic pulses in relatively focal regions, tDCS induces weaker but more extensive currents between anodal and cathodal poles. While these studies have repeatedly shown that NIBS could be applied safely in patients, the available evidence fails to show a clear treatment effect across studies. There is consensus that the effectiveness of NIBS in ASD should be tested by larger randomized, controlled trials (Oberman, Enticott, et al. 2016a; Cole et al. 2019; García-González et al. 2021). Finding an effective treatment further relies on identifying relevant outcome measures that can be aligned with pathophysiological hypotheses, accessible by NIBS (Oberman, Enticott, et al. 2016a). In particular, measuring stimulation success will require quantitative measures of social abilities (Lahnakoski et al. 2020, 2022) related to neural systems (Polanía et al. 2018). Taken together, the increased efforts of larger-scale randomized controlled trials present investigators with the challenge of choosing the optimal strategy for applying NIBS.
The goal of the present review is to generate a synopsis of previous trials applying NIBS in ASD, focusing on treatment rationale and methodological implications. Aiming at reducing heterogeneity, we restricted our review on neuromodulatory effects examined in late adolescence and adulthood. After reviewing the available evidence, we discuss future strategies from a clinician scientist’s perspective, comparing available pathophysiological models, choices of stimulation protocols, and potential targeting methods. We identify the main strategies of previous NIBS approaches in ASD and discuss how methodological advances may be implemented to develop personalized study protocols in the future.
Materials and methods
We reviewed publications in peer-reviewed journals, which have tested the effects of NIBS during mid to late adolescence and adulthood in patients with ASD. Only clinical cohorts with a confirmed diagnosis were included (for details on diagnostics for each study see Table 1). A selective search of the literature was performed in the scientific databases PubMed, Cochrane, Google Scholar, and Web of Science using the search terms “Autism,” “Autism Spectrum Disorder,” “Asperger,” as well as “noninvasive brain stimulation,” “tDCS,” “TMS,” “rTMS,” “Theta Burst,” and “TBS.” All search results published within the last 15 years were included in the selection process. After eliminating duplicate findings, titles and abstracts were filtered for potential relevance regarding the research question of the present review, based on predefined inclusion and exclusion criteria, aiming to include studies that reported (i) data on patients with ASD and (ii) applying NIBS, such as rTMS and tDCS to alter autism-related symptoms (Fig. 1). Studies that used TMS to test neural plasticity (Oberman, Ifert-Miller, et al. 2016b; Jannati et al. 2021) or to measure motor evoked potentials instead of inducing lasting neuromodulatory effects (Théoret et al. 2005; Minio-Paluello et al. 2009) were not included. After this selection process, 24 of the initial 631 studies were reviewed in full. In this second review process, studies were excluded which (i) were performed in clinical populations with a mean age lower than 18 years, (ii) insufficient sample size (case studies or case series without sufficient data for testing NIBS effects), and studies which (iii) did not report primary data. To ensure that all aforementioned criteria were met in the final selection of studies, two experienced psychiatrists (JL and DK) independently extracted data on authors, year of publication, study design, cohort sizes, and age of patients. Since the present review aimed at providing an overview of NIBS strategies, the full-text articles were further reviewed for details on methodology, including hardware, stimulation protocols, and potential navigation systems as well as additional information analogous to the PRISMA guidelines.
Publication . | Study design . | Stimulation . | Randomized . | Blinding . | Control group . | Diagnostic criteria . | n (total) . | Mean age . |
---|---|---|---|---|---|---|---|---|
Sokhadze et al. 2009 | Quasi-Experiment | rTMS | no | no | waiting-list (n = 5) | DSM IV, ADI-R | 13 (5 controls) | 18.3 + −4.8 |
Fecteau et al. 2011 | Crossover | rTMS | yes | double | sham-rTMS | DSM IV, AAA | 10 | 36.6 + −16 |
Enticott et al. 2014 | RCT | rTMS | yes | double | sham-rTMS (n = 15) | DSM IV | 30 (15 controls) | 33.87 + −13.07 |
D Urso et al. 2015 | Open label | tDCS | no | no | none | clinical record | 10 | 20.4 + −2.8 |
Ni et al. 2017 | Crossover | rTMS | yes | no | sham-rTMS | DSM IV, ICD-10 | 19 | 20.8 + −1.4 |
van Steenburg et al. 2017 | Crossover | tDCS | yes | single | sham-tDCS | clinical record, ADOS | 12 | 32.1 + −12.4 |
Publication . | Study design . | Stimulation . | Randomized . | Blinding . | Control group . | Diagnostic criteria . | n (total) . | Mean age . |
---|---|---|---|---|---|---|---|---|
Sokhadze et al. 2009 | Quasi-Experiment | rTMS | no | no | waiting-list (n = 5) | DSM IV, ADI-R | 13 (5 controls) | 18.3 + −4.8 |
Fecteau et al. 2011 | Crossover | rTMS | yes | double | sham-rTMS | DSM IV, AAA | 10 | 36.6 + −16 |
Enticott et al. 2014 | RCT | rTMS | yes | double | sham-rTMS (n = 15) | DSM IV | 30 (15 controls) | 33.87 + −13.07 |
D Urso et al. 2015 | Open label | tDCS | no | no | none | clinical record | 10 | 20.4 + −2.8 |
Ni et al. 2017 | Crossover | rTMS | yes | no | sham-rTMS | DSM IV, ICD-10 | 19 | 20.8 + −1.4 |
van Steenburg et al. 2017 | Crossover | tDCS | yes | single | sham-tDCS | clinical record, ADOS | 12 | 32.1 + −12.4 |
AAA, Adult Asperger Assessment; ADI-R, Autism Diagnostic Interview—Revised; ADOS, The Autism Diagnostic Observation Schedule; DSM-IV, Diagnostic and Statistical Manual of Mental Disorders IV; ICD-10, International Classification of Diseases, Tenth Revision.
Publication . | Study design . | Stimulation . | Randomized . | Blinding . | Control group . | Diagnostic criteria . | n (total) . | Mean age . |
---|---|---|---|---|---|---|---|---|
Sokhadze et al. 2009 | Quasi-Experiment | rTMS | no | no | waiting-list (n = 5) | DSM IV, ADI-R | 13 (5 controls) | 18.3 + −4.8 |
Fecteau et al. 2011 | Crossover | rTMS | yes | double | sham-rTMS | DSM IV, AAA | 10 | 36.6 + −16 |
Enticott et al. 2014 | RCT | rTMS | yes | double | sham-rTMS (n = 15) | DSM IV | 30 (15 controls) | 33.87 + −13.07 |
D Urso et al. 2015 | Open label | tDCS | no | no | none | clinical record | 10 | 20.4 + −2.8 |
Ni et al. 2017 | Crossover | rTMS | yes | no | sham-rTMS | DSM IV, ICD-10 | 19 | 20.8 + −1.4 |
van Steenburg et al. 2017 | Crossover | tDCS | yes | single | sham-tDCS | clinical record, ADOS | 12 | 32.1 + −12.4 |
Publication . | Study design . | Stimulation . | Randomized . | Blinding . | Control group . | Diagnostic criteria . | n (total) . | Mean age . |
---|---|---|---|---|---|---|---|---|
Sokhadze et al. 2009 | Quasi-Experiment | rTMS | no | no | waiting-list (n = 5) | DSM IV, ADI-R | 13 (5 controls) | 18.3 + −4.8 |
Fecteau et al. 2011 | Crossover | rTMS | yes | double | sham-rTMS | DSM IV, AAA | 10 | 36.6 + −16 |
Enticott et al. 2014 | RCT | rTMS | yes | double | sham-rTMS (n = 15) | DSM IV | 30 (15 controls) | 33.87 + −13.07 |
D Urso et al. 2015 | Open label | tDCS | no | no | none | clinical record | 10 | 20.4 + −2.8 |
Ni et al. 2017 | Crossover | rTMS | yes | no | sham-rTMS | DSM IV, ICD-10 | 19 | 20.8 + −1.4 |
van Steenburg et al. 2017 | Crossover | tDCS | yes | single | sham-tDCS | clinical record, ADOS | 12 | 32.1 + −12.4 |
AAA, Adult Asperger Assessment; ADI-R, Autism Diagnostic Interview—Revised; ADOS, The Autism Diagnostic Observation Schedule; DSM-IV, Diagnostic and Statistical Manual of Mental Disorders IV; ICD-10, International Classification of Diseases, Tenth Revision.
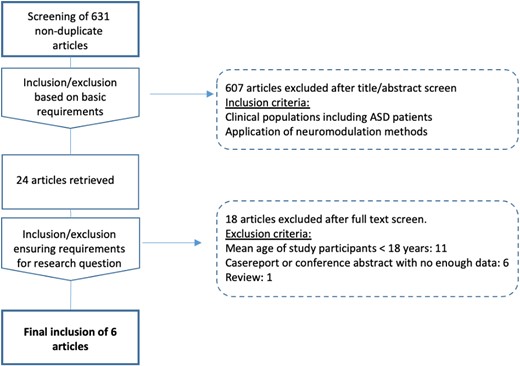
Data screening and selection. Flow diagram illustrating the process of identification, screening, and final inclusion of studies on noninvasive brain stimulation applied in adolescents and adults with ASDs.
Results
Six publications met the selection criteria, examining the effects of NIBS in adults with ASD (Sokhadze et al. 2009; Fecteau et al. 2011; Enticott et al. 2014; D’Urso et al. 2015; Ni et al. 2017; Steenburgh et al. 2017). An overview of stimulation targets is shown in Fig. 2. In total, 74 patients with ASD with a mean age of 27.09 years underwent NIBS. Four studies used rTMS (Sokhadze et al. 2009; Fecteau et al. 2011; Enticott et al. 2014; Ni et al. 2017), including one study using iTBS (Ni et al. 2017) and two studies applied tDCS (D’Urso et al. 2015; Steenburgh et al. 2017). As the most frequently used target, four studies aimed to modulate the dorsolateral prefrontal cortex (DLPFC) (Sokhadze et al. 2009; D’Urso et al. 2015; Ni et al. 2017; Steenburgh et al. 2017), one study applied rTMS on the dorsomedial prefrontal cortex (Enticott et al. 2014), and one study targeted the inferior frontal gyrus, in pars triangularis and pars opercularis, corresponding to Brodman areas 44 and 45 (Fecteau et al. 2011). Stimulation was applied unilaterally (Sokhadze et al. 2009; Fecteau et al. 2011; D’Urso et al. 2015) and bilaterally (Enticott et al. 2014; Ni et al. 2017; Steenburgh et al. 2017). Stimulation protocols were found to be highly heterogeneous regarding intensity, duration, and number of sessions. Details are provided in Table 2. Moreover, the main behavioral outcome measures which were expected to be altered by applying NIBS are summarized in Table 2, showing marked differences between studies. For example, perceptual information processing was assessed using an oddball task (Sokhadze et al. 2009), word retrieval was tested using the Boston Naming Test (BNT) (Fecteau et al. 2011), mentalizing was assessed using a “reading the mind in the eyes”-task and an “animations mentalizing test” (Enticott et al. 2014). Attention and executive function were measured using the Conner’s Continuous Performance Test (CCPT), the Wisconsin Card Sorting Test (WCST) (Ni et al. 2017), and the n-back task as a brief test of attention (BTA). Behavioral assessment, including repetitive behaviors, social communication, and overall clinical presentation, was conducted using the Aberrant Behavior Checklist (ABC), the revised Repetitive Behavior Scale (RBS-R), the Social Responsiveness Scale (SRS) (Sokhadze et al. 2009; D’Urso et al. 2015), Clinical Global Impression (CGI) the Ritvo Autism Asperger’s Diagnostic Scale (RAADS), the Autism-Spectrum-Quotient (AQ), and the Interpersonal Reactivity Index (IRI), as well as the Yale-Brown Obsessive-Compulsive Scale (Y-BOCS) (D’Urso et al. 2015).
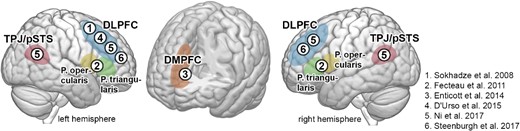
The two studies modulating left DLPFC followed the rationale that an upregulation of inhibitory frontal systems leads to the amelioration of stereotypical behavior (for details see Table 2) (Sokhadze et al. 2009; D’Urso et al. 2015). Moreover, two studies aiming to increase the function of bilateral DLPFC reported improvements in sustained attention (Ni et al. 2017) and executive function (Steenburgh et al. 2017) after stimulation. It remains unclear why both inhibitory and excitatory protocols could lead to beneficial effects in different functional domains. That is, low-frequency rTMS and cathodal tDCS are considered to result in overall inhibitory aftereffects, which both—applied on the left DLPFC—improved stereotypical behavior and working memory (Sokhadze et al. 2009; D’Urso et al. 2015). On the other hand, although iTBS and anodal tDCS are considered to induce excitatory effects, i.e. opposite effects to the aforementioned protocols, they also led to an improvement of cognitive function when targeting bilateral DLPFC (Ni et al. 2017; Steenburgh et al. 2017). Steenburgh et al. (2017) applied both anodal and cathodal tDCS on each (left and right) DLPFC, with the opposite pole connected to the respective other DLPFC, thereby testing the effects of DLPFC excitation in each hemisphere while presumably inhibiting the contralateral DLPFC in the same patient population. Interestingly, in both conditions, tDCS improved working memory compared with sham tDCS. The authors interpreted this finding as pointing toward the potential recruitment of more distant brain regions involved in the network mediating cognitive control.
Such network-wide effects were also expected in studies targeting other regions, including bilateral DMPFC (Enticott et al. 2014) and bilateral pSTS (Ni et al. 2017). The so-called mentalizing network, which is known to subserve mental state attribution and successful participation in social interaction (Redcay and Schilbach 2019), shows abnormally low activation in patients with ASD (Castelli et al. 2002; Mason et al. 2008; Redcay et al. 2013), which led to the hypothesis that excitatory rTMS of these regions may ameliorate social cognitive deficits. This idea could be partly confirmed by the studies’ outcomes, showing that 10 sessions of 5 Hz rTMS over the DMPFC could improve social relating assessed by the social relatedness subscore of the RAADS (Enticott et al. 2014). Further obsessive-compulsive symptoms were ameliorated in patients with ASD after iTBS of bilateral pSTS (Ni et al. 2017). Yet, other social cognitive tasks implemented in these studies failed to show significant effects. While there is proof-of-concept data that the mentalizing network can be targeted to treat social impairments in ASD, the specific processes that can be thereby modulated require further investigation.
Publication . | NIBS placement . | Spatial precision . | Hz . | MT . | Pulses* Session . | mA . | Outcome assessment . | NIBS effects . | Discussed rationale . |
---|---|---|---|---|---|---|---|---|---|
Sokhadze et al. 2009 | left DLPFC | landmark-oriented, 5 cm anterior to motor hotspot | 0.5 | 90% rMT | 150 * 6 | oddball task, ABC, SRS, CGI, RBS-R | Improved repetitive behaviors (RSB-R) | supporting frontal systems for cortical inhibition | |
Fecteau et al. 2011 | pars opercularis (left, right), pars triangularis (left, right) | stereotactic neuronavigation, structural MRI Targets | 1 | 70% rMT | 1800 * 5 | Boston Naming Test | pars opercularis left: faster naming; pars triangularis left: slower naming | inhibiting frontal abnormally connected regions may aid or disrupt function | |
Enticott et al. 2014 | bilateral DMPFC | landmark-oriented, 7 cm anterior to M1 | 5 | 100% rMT | 1500 * 10 | Reading the mind in the eyes test, animations mentalizing test, RAADS, AQ, IRI | improvement in social relatedness subscore of RAADS and personal distress subscale of IRI | enhancing reduced activity in frontal aspects of the mentalizing network | |
D Urso et al. 2015 | left cathodal DLPFC/right arm | landmark-oriented (10–20 electrode system) | 10 Sessions | 1.5 | ABC | Improved autistic symptoms including irritability, lethargy, hyperactivity subscales | Enhancing frontal inhibitory circuits by reduction of cortical excitability | ||
Ni et al. 2017 | bilateral pSTS, bilateral DLPFC | stereotactic neuronavigation, group-derived functional targets, transformed into single-subject anatomical MRI | 50 (iTBS) | 80% aMT, sham: 60% aMT | 600 * 3 | CCPT, WCST, Y-BOCS, SRS | DLPFC: increased sustained attention (CCPT) and social communication subscale of SRS; pSTS: decreased compulsive behaviors (Y-BOCS) | supporting frontal systems for cortical inhibition | |
van Steenburg et al. 2017 | left anodal/right cathodal DLPFC, right anodal/left cathodal DLPFC | landmark-oriented (10–20 electrode system) | 3 Sessions | 1.5 | n-back, BTA | Improved working memory | enhancing function of hypoactive prefrontal systems underlying working memory |
Publication . | NIBS placement . | Spatial precision . | Hz . | MT . | Pulses* Session . | mA . | Outcome assessment . | NIBS effects . | Discussed rationale . |
---|---|---|---|---|---|---|---|---|---|
Sokhadze et al. 2009 | left DLPFC | landmark-oriented, 5 cm anterior to motor hotspot | 0.5 | 90% rMT | 150 * 6 | oddball task, ABC, SRS, CGI, RBS-R | Improved repetitive behaviors (RSB-R) | supporting frontal systems for cortical inhibition | |
Fecteau et al. 2011 | pars opercularis (left, right), pars triangularis (left, right) | stereotactic neuronavigation, structural MRI Targets | 1 | 70% rMT | 1800 * 5 | Boston Naming Test | pars opercularis left: faster naming; pars triangularis left: slower naming | inhibiting frontal abnormally connected regions may aid or disrupt function | |
Enticott et al. 2014 | bilateral DMPFC | landmark-oriented, 7 cm anterior to M1 | 5 | 100% rMT | 1500 * 10 | Reading the mind in the eyes test, animations mentalizing test, RAADS, AQ, IRI | improvement in social relatedness subscore of RAADS and personal distress subscale of IRI | enhancing reduced activity in frontal aspects of the mentalizing network | |
D Urso et al. 2015 | left cathodal DLPFC/right arm | landmark-oriented (10–20 electrode system) | 10 Sessions | 1.5 | ABC | Improved autistic symptoms including irritability, lethargy, hyperactivity subscales | Enhancing frontal inhibitory circuits by reduction of cortical excitability | ||
Ni et al. 2017 | bilateral pSTS, bilateral DLPFC | stereotactic neuronavigation, group-derived functional targets, transformed into single-subject anatomical MRI | 50 (iTBS) | 80% aMT, sham: 60% aMT | 600 * 3 | CCPT, WCST, Y-BOCS, SRS | DLPFC: increased sustained attention (CCPT) and social communication subscale of SRS; pSTS: decreased compulsive behaviors (Y-BOCS) | supporting frontal systems for cortical inhibition | |
van Steenburg et al. 2017 | left anodal/right cathodal DLPFC, right anodal/left cathodal DLPFC | landmark-oriented (10–20 electrode system) | 3 Sessions | 1.5 | n-back, BTA | Improved working memory | enhancing function of hypoactive prefrontal systems underlying working memory |
Neuromodulation techniques and rationale of selected studies. Stimulation parameters: aMT, active motor threshold; mA, milliampere; rMT, resting motor threshold. Outcome assessment: ABC, Aberrant Behavior Checklist; AQ, Autism-Spectrum Quotient; BTA, Behavior Test A; CCPT, Continuous Performance Test; CGI, Clinical Global Impression; IRI, Interpersonal Reactivity Index; RAADS, Ritvo Autism Asperger Diagnostic Scale-Revised; RSB-R, Repetitive Behaviors Scale-Revised; SRS, Social Responsiveness Scale; WCST, Wisconsin Card Sorting Test; Y-BOCS, Yale-Brown Obsessive-Compulsive Scale.
Publication . | NIBS placement . | Spatial precision . | Hz . | MT . | Pulses* Session . | mA . | Outcome assessment . | NIBS effects . | Discussed rationale . |
---|---|---|---|---|---|---|---|---|---|
Sokhadze et al. 2009 | left DLPFC | landmark-oriented, 5 cm anterior to motor hotspot | 0.5 | 90% rMT | 150 * 6 | oddball task, ABC, SRS, CGI, RBS-R | Improved repetitive behaviors (RSB-R) | supporting frontal systems for cortical inhibition | |
Fecteau et al. 2011 | pars opercularis (left, right), pars triangularis (left, right) | stereotactic neuronavigation, structural MRI Targets | 1 | 70% rMT | 1800 * 5 | Boston Naming Test | pars opercularis left: faster naming; pars triangularis left: slower naming | inhibiting frontal abnormally connected regions may aid or disrupt function | |
Enticott et al. 2014 | bilateral DMPFC | landmark-oriented, 7 cm anterior to M1 | 5 | 100% rMT | 1500 * 10 | Reading the mind in the eyes test, animations mentalizing test, RAADS, AQ, IRI | improvement in social relatedness subscore of RAADS and personal distress subscale of IRI | enhancing reduced activity in frontal aspects of the mentalizing network | |
D Urso et al. 2015 | left cathodal DLPFC/right arm | landmark-oriented (10–20 electrode system) | 10 Sessions | 1.5 | ABC | Improved autistic symptoms including irritability, lethargy, hyperactivity subscales | Enhancing frontal inhibitory circuits by reduction of cortical excitability | ||
Ni et al. 2017 | bilateral pSTS, bilateral DLPFC | stereotactic neuronavigation, group-derived functional targets, transformed into single-subject anatomical MRI | 50 (iTBS) | 80% aMT, sham: 60% aMT | 600 * 3 | CCPT, WCST, Y-BOCS, SRS | DLPFC: increased sustained attention (CCPT) and social communication subscale of SRS; pSTS: decreased compulsive behaviors (Y-BOCS) | supporting frontal systems for cortical inhibition | |
van Steenburg et al. 2017 | left anodal/right cathodal DLPFC, right anodal/left cathodal DLPFC | landmark-oriented (10–20 electrode system) | 3 Sessions | 1.5 | n-back, BTA | Improved working memory | enhancing function of hypoactive prefrontal systems underlying working memory |
Publication . | NIBS placement . | Spatial precision . | Hz . | MT . | Pulses* Session . | mA . | Outcome assessment . | NIBS effects . | Discussed rationale . |
---|---|---|---|---|---|---|---|---|---|
Sokhadze et al. 2009 | left DLPFC | landmark-oriented, 5 cm anterior to motor hotspot | 0.5 | 90% rMT | 150 * 6 | oddball task, ABC, SRS, CGI, RBS-R | Improved repetitive behaviors (RSB-R) | supporting frontal systems for cortical inhibition | |
Fecteau et al. 2011 | pars opercularis (left, right), pars triangularis (left, right) | stereotactic neuronavigation, structural MRI Targets | 1 | 70% rMT | 1800 * 5 | Boston Naming Test | pars opercularis left: faster naming; pars triangularis left: slower naming | inhibiting frontal abnormally connected regions may aid or disrupt function | |
Enticott et al. 2014 | bilateral DMPFC | landmark-oriented, 7 cm anterior to M1 | 5 | 100% rMT | 1500 * 10 | Reading the mind in the eyes test, animations mentalizing test, RAADS, AQ, IRI | improvement in social relatedness subscore of RAADS and personal distress subscale of IRI | enhancing reduced activity in frontal aspects of the mentalizing network | |
D Urso et al. 2015 | left cathodal DLPFC/right arm | landmark-oriented (10–20 electrode system) | 10 Sessions | 1.5 | ABC | Improved autistic symptoms including irritability, lethargy, hyperactivity subscales | Enhancing frontal inhibitory circuits by reduction of cortical excitability | ||
Ni et al. 2017 | bilateral pSTS, bilateral DLPFC | stereotactic neuronavigation, group-derived functional targets, transformed into single-subject anatomical MRI | 50 (iTBS) | 80% aMT, sham: 60% aMT | 600 * 3 | CCPT, WCST, Y-BOCS, SRS | DLPFC: increased sustained attention (CCPT) and social communication subscale of SRS; pSTS: decreased compulsive behaviors (Y-BOCS) | supporting frontal systems for cortical inhibition | |
van Steenburg et al. 2017 | left anodal/right cathodal DLPFC, right anodal/left cathodal DLPFC | landmark-oriented (10–20 electrode system) | 3 Sessions | 1.5 | n-back, BTA | Improved working memory | enhancing function of hypoactive prefrontal systems underlying working memory |
Neuromodulation techniques and rationale of selected studies. Stimulation parameters: aMT, active motor threshold; mA, milliampere; rMT, resting motor threshold. Outcome assessment: ABC, Aberrant Behavior Checklist; AQ, Autism-Spectrum Quotient; BTA, Behavior Test A; CCPT, Continuous Performance Test; CGI, Clinical Global Impression; IRI, Interpersonal Reactivity Index; RAADS, Ritvo Autism Asperger Diagnostic Scale-Revised; RSB-R, Repetitive Behaviors Scale-Revised; SRS, Social Responsiveness Scale; WCST, Wisconsin Card Sorting Test; Y-BOCS, Yale-Brown Obsessive-Compulsive Scale.
Regarding psychopharmacological treatment, four studies reported that medication was present in up to 39% of participants (Enticott et al. 2014) and continued during NIBS treatment. Two studies did not report if medication was being continued during the intervention (Sokhadze et al. 2009; Steenburgh et al. 2017).
Adverse events were reported in three of the six reviewed studies, none of them fulfilled the criteria for classification as serious adverse events. In one of the studies, no information was found regarding adverse events (Sokhadze et al. 2009). Applying rTMS over the inferior frontal gyrus (Fecteau et al. 2011) induced adverse effects in 6 out of 10 participants, comprising sleepiness (n = 3), stiff neck (n = 2), trouble concentrating (n = 2), subtle disorientation (n = 1), dizziness (n = 1), headache (n = 1), increased emotionality (n = 1), and discomfort at the stimulation site (n = 1). When applying rTMS over the DMPFC (Enticott et al. 2014), 3 of 15 patients reported adverse effects, lightheadedness (n = 1), and minor facial discomfort (n = 2). Applying iTBS over DLPFC was reported to induce discomfort due to twitches around the eyes in 3 of 19 patients (Ni et al. 2017). The two studies applying tDCS reported the absence of adverse effects (D’Urso et al. 2015; Steenburgh et al. 2017).
Discussion
The review of six available studies investigating NIBS effects on autism-related functional domains in adolescent and adult patients with ASD illustrates different strategies to modulate neural systems contributing to social cognitive abilities. The small number of studies, which varied in study protocols, control conditions, and target regions, leads to the conclusion that currently applied NIBS protocols do not allow testing for systematic effects of NIBS on social cognition in ASD. Rather, the studies reviewed have demonstrated feasibility and safety for different stimulation protocols and treatment targets, suggesting that some symptoms of autism, such as stereotypical behaviors, may be improved. Based on the reviewed literature, we review the rationales and limitations of previous studies when targeting specific neural systems. In this context, we first address the potential placebo effects of NIBS, which must be considered to enhance the robustness of experiment designs. Further, we discuss strategies and methodological advances that may reduce interindividual variability in future studies, aiding in personalize NIBS in ASD.
Rationale of NIBS in ASD
In all of the studies reviewed here, NIBS is applied under the assumption that a modulation of specific brain regions or neuronal populations can lead to altered processing, and thus alter behavior, ideally ameliorating ASD symptoms. Both NIBS techniques, rTMS (Pascual-Leone et al. 1996; Chen et al. 1997) and tDCS (Boggio et al. 2007) can be used to induce lasting changes in cortical excitability. At the cellular level, these effects are explained by neuronal long-term potentiation and depression (Ilić and Ziemann 2005; Esser et al. 2006). Notably, tDCS and rTMS pose fundamentally different methods in how to interfere with neural tissue. During tDCS, cortical excitation is modulated by a weak current between an anodal and cathodal electrode (Woods et al. 2016), with reduced excitation at the cathodal site and increased excitation at the anodal site. In contrast, rTMS is delivered via trains of magnetic pulses, inducing currents in the neural tissue below the TMS coil (Lefaucheur et al. 2020). Therefore, the focal magnetic field of rTMS allows more selective targeting of brain regions compared to the diffuse currents of tDCS but may be experienced as more aversive due to the co-stimulation of muscle and cutaneous nerves (Groppa et al. 2012; Rossini et al. 2015). The frequency of magnetic pulses is decisive for effect on neural populations, with low-frequency rTMS (i.e. <5 Hz) tending to suppress neural excitability, whereas high-frequency protocols (i.e. >5 Hz) increase excitability (Hoogendam et al. 2010). While traditional rTMS protocols require patients with ASD to sit still for 15–30 minutes (Fecteau et al. 2011; Enticott et al. 2014), intermittent Theta burst stimulation (iTBS) has been shown to increase cortical excitability after less than 4 minutes and at relatively low stimulation intensities (Huang et al. 2005), applying a total of 600 pulses in short bursts of three pulses at 50 Hz at a theta-frequency (5 Hz) for 2 seconds, which is repeated every 10 seconds. In ASD, this protocol has been tested targeting DLPFC and pSTS in both hemispheres by Ni et al. (2017), who report beneficial effects on attention (DLPFC) and compulsive behavior (pSTS) after iTBS. While iTBS leads to increased excitability in most individuals, a continuous Theta burst stimulation (cTBS) has been shown to decrease cortical excitability (Huang et al. 2005; Lazzaro et al. 2005). NIBS is safe and well tolerated by most recipients (Rossi et al. 2009), gaining relevance in scientific investigations and clinical practice. At the clinical end, NIBS has been investigated as a treatment option for multiple diseases affecting the central nervous system. To list some examples, positive effects have been reported in therapy-refractory depression (Sonmez et al. 2019; Qiu et al. 2023), bipolar disorder (Yatham et al. 2021), schizophrenia (Wang et al. 2017), obsessive-compulsive disorder (Zhou et al. 2017; Carmi et al. 2018), Alzheimer’s disease (Hsu et al. 2015), and stroke (Hsu et al. 2012). Importantly, many of the aforementioned studies observed a high interindividual variability of effects, which remains a major challenge when aiming to define generally effective treatment protocols. Furthermore, the chosen regions across these studies depended highly on the studies’ objectives. The DLPFC, for example, is frequently targeted to ameliorate depressive (Sonmez et al. 2019) and obsessive-compulsive symptoms (Zhou et al. 2017), inferior frontal and temporoparietal regions were targeted when aiming to improve language and memory in Alzheimer’s disease (Hsu et al. 2015), and M1 was modulated to improve motor deficits after stroke (Hsu et al. 2012). Regarding ASD, most studies have chosen to modulate the left DLPFC to improve executive function and stereotypical behaviors (Sokhadze et al. 2009; D’Urso et al. 2015; Ni et al. 2017; Steenburgh et al. 2017), while less is known about modulating social cognitive processes, such as mentalizing by targeting DMPFC (Enticott et al. 2014).
Controlling for placebo effects
A major challenge in measuring the impact of NIBS on behavior is minimizing potential placebo effects, which requires rigorous implementation of control conditions in study designs (Oberman, Enticott, et al. 2016a; Cole et al. 2019; García-González et al. 2021). Out of the six studies included in this review, three were, in fact, not blinded (Ni et al. 2017), and two of which did not contain an NIBS intervention as a control condition (Sokhadze et al. 2009; D’Urso et al. 2015) (Table 1). Consequently, the observed changes in patients’ behavior in these studies may be confounded by placebo effects, which can have critical effects on the study outcome. For instance, in patients with medication refractory depression, substantial improvement of symptoms was observed not only after real rTMS but likewise in patients receiving control (sham) rTMS (Dunlop et al. 2020). Such findings underscore the importance of recognizing that NIBS procedures are inherently prone to inducing expectations that may bias outcomes in neuropsychological interventions (Rabipour et al. 2019). Future study designs could minimize such biases by implementing realistic sham conditions that rely on sham coils or coil placements and interventions, which induce sensations but do not reach neural tissue (Duecker and Sack 2015). Since this review focuses on innovations potentially optimizing NIBS in ASD, we will discuss solutions to control for potential placebo effects when investigating such advances in future trials.
Personalized NIBS target selection
The choice of stimulation site is considered relevant for the successful induction of effects in NIBS studies (Polanía et al. 2018; Cash et al. 2020; Penton et al. 2020; Lynch et al. 2022). Previous and current research suggests DLPFC, pSTS, IFG, TPJ, and DMPFC to be hypothesis-driven targets based on the neural systems involved in ASD. These brain regions are also known to be involved in social cognition and therefore targeted in other NIBS studies aiming to interfere with the ability to mentalize across healthy and patient populations (Schuwerk et al. 2014), yet it remains unclear which region should be selected when aiming to modulate specific aspects of behavior. Evidence from systems neuroscience suggests that global interactions between distant brain regions are relevant for social and cognitive functions in ASD (May and Kana 2020; Sha et al. 2022). Appreciating that NIBS alters neural activation not only regionally, but also in distant regions, may explain why tDCS induced effects on working memory even after switching electrodes between bilateral DLPFC (Steenburgh et al. 2017). Neuromodulatory effects distant from the stimulation site have also been demonstrated for rTMS, showing that the modulation of a focal brain area leads to altered activation of distant regions (Beynel et al. 2020). Taken together, the rationale of previous studies and recent findings from systems neuroscience suggest that proximal as well as distant effects should be considered when selecting a target region for NIBS. Notably, many of the previously selected regions, such as TPJ, DLPFC, and DMPFC are functionally subdivided, with each subregion interacting with different functional networks (Shih et al. 2011; Cieslik et al. 2012; Bzdok et al. 2013; Eickhoff et al. 2016; Cheng et al. 2018; Jung et al. 2022) (Fig. 3A). For example, based on functional connectivity and task activation, the anterior portion of the right TPJ has been associated with language and phonology-related tasks and shows extensive functional connectivity with the auditory cortex, whereas the posterior right TPJ was linked to social cognitive tasks and functional connectivity with the dmPFC (Mars et al. 2012; Bzdok et al. 2013). To illustrate another example, a coactivation-based parcellation of the DLPFC suggested a functional division into an anterior-ventral cluster, associated with action inhibition processes and a posterior-dorsal cluster, linked to action execution and working memory. Such functional discrepancies between neighboring subregions highlight the need for precision methods when targeting neural systems, such as networks underlying social cognition or executive function in ASD. In addition, due to interindividual neuroanatomical differences, there is a high risk of missing the intended electric field when using traditional methods of rTMS coil placement and orientation (Balslev et al. 2007; Caulfield et al. 2022). To overcome this problem, stereotactic neuronavigation systems have been demonstrated to increase spatial precision and, thereby, facilitate a more efficient modulation of the target region (Ahdab et al. 2010; Peleman et al. 2010; Schönfeldt-Lecuona et al. 2010). Given the expected high interindividual variability of functional activation patterns during social cognitive tasks (Ojemann et al. 1989; Frost and Goebel 2012; Wang et al. 2015), it has been suggested as a strategy for future studies to assess brain function in potential target regions on the single-subject level before NIBS using neuroimaging methods, such as MEG or fMRI (Polanía et al. 2018). Indeed, the use of stereotactic neuronavigation systems to target single-subject fMRI-derived maxima has been shown to significantly outperform cranial landmark-oriented approaches (Sparing et al. 2008). FMRI cannot only be used to localize targets with the highest activation peaks at the single subject level (Sparing et al. 2008; Hensel et al. 2021, 2022) but also to identify targets based on their connectivity to specific brain networks (Cash et al. 2019, 2020; Lynch et al. 2021). Importantly, such connectivity-derived targets could be reproduced within individuals across different scanning sessions, even after 1 year (Cash et al. 2021). Targeting circuits rather than single brain regions is of particular interest when aiming to expand stimulation effects to deeper brain regions, such as the subgenual cingulum when for instance treating major depressive disorder. Targeting neural networks for social cognition in ASD, the TPJ might analogously allow to expand NIBS effects to deeper medial prefrontal regions (Fig. 3A). Recent endeavors of personalizing NIBS protocols further suggest accounting for anatomical variability, performing electric field simulations to estimate the optimal coil position (Balderston et al. 2020; Lynch et al. 2022). Lynch et al. (2022) introduced an automated pipeline that uses resting-state fMRI to localize functional networks, which are subsequently masked by reconstructed sulcal maps based on anatomical MRI scans, yielding personalized targets with the highest possible electric fields in functionally defined regions of interest. By reducing the variability in the effectively targeted functional networks, this approach has been suggested as a promising strategy for personalizing the application of rTMS in depression (Cash et al. 2021; Lynch et al. 2021). Surprisingly, only 2 out of the 6 studies reviewed here have integrated a stereotactic neuronavigation system in their experimental setup (Fecteau et al. 2011; Ni et al. 2017). Fecteau et al. (2011) thereby ensured that rTMS was correctly applied on specific macroanatomical structures (i.e. pars operculares and triangulares), as located by single-subject structural MRI. In contrast, Ni et al. (2017) targeted meta-analytically defined peaks, based on task fMRI studies on mentalizing in neurotypical cohorts (Overwalle and Baetens 2009). Yet, we could not find any study applying techniques, such as fMRI, EEG, connectivity measures, or electric field simulations to optimize targets individually in ASD. These methods could provide sufficient temporal and spatial resolution to localize personalized targets and examine whether these targets remain stable over time. To test whether electric field modeling can identify effective treatment targets for NIBS in ASD, future studies might implement two arms comparing effects from one arm defining stimulation sites by anatomical landmarks and a second arm defining targets by electric field models. Likewise, the benefit of personalized targets (e.g. defined by fMRI) may be tested by comparing NIBS based on individual fMRI targets in one arm, and group-activation-based targets in another. In conclusion, the subregional organization and potential interindividual structural and functional differences offer an opportunity to reduce the variability of NIBS effects (Fig. 3B), calling for randomized controlled trials that specifically probe the effectiveness of personalized targets.
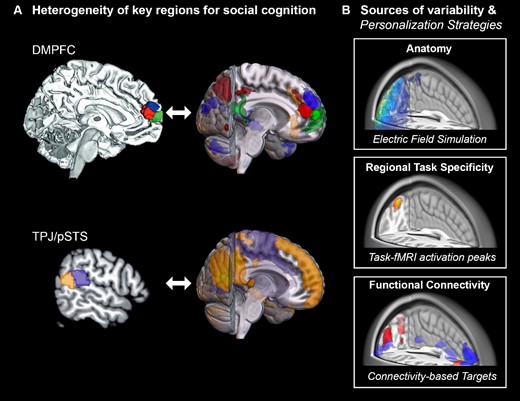
Heterogeneity of regions and personalization strategies. A) Parcellations of two key regions involved in social cognition, DMPFC and TPJ, based on dissociable fMRI co-activation patterns. Subregion-specific functional connectivity patterns are shown to the left of each parcellation. The data for the illustrations were shared with friendly permission (Bzdok et al. 2013; Eickhoff et al. 2016). B) Schematic example of strategies accounting for intersubject variability for NIBS on the single-subject level. Top: Optimal extracranial device positions and required stimulation intensities can be estimated based on individual anatomy. Middle: Intracranial target may be defined by task-specific activation or, bottom: by connectivity to a predefined network of interest.
Behavioral effects through modulation of neural systems
Patients seeking therapy to ameliorate symptoms of ASD are often treated with psychotherapeutic approaches, which access disease mechanisms from the behavioral level. Given the increasing interest in the neurobiology underlying ASD, there is a growing potential in using NIBS to modulate neural systems which are linked to specific behaviors. It has been suggested that neuroplastic changes induced by NIBS may lead to larger effects if combined with subsequent behavioral training, for example, by psychotherapy. From a multi-level perspective, ranging from genetics to brain networks, and behavior (Piggot et al. 2009; Geschwind and Flint 2015; Lahnakoski et al. 2022), NIBS may allow modulating neural processes where psychological approaches may be limited or where modulation of neural systems may be a prerequisite for psychotherapeutic success. For example, on the behavioral level, Sokhadze et al. reported that patients with ASD were prone to errors in the Kanizsa Illusory Figure Test, a task that requires both perceptual and attentional resources. At the neurophysiological level, the authors elegantly align the behavioral level to the neurophysiological data using event-related potentials in EEG recordings, demonstrating that rTMS over the DLPFC alters neural processing. Targeting the DLPFC in ASD patients has been motivated by the abnormally low activation in this region in patients with ASD (Chantiluke et al. 2015; Carlisi et al. 2017; Lukito et al. 2020). The preference for targeting the DLPFC as well as the tendency of reporting beneficial effects is in line with a recent review on NIBS in ASD, which additionally included single-case studies and populations in younger patients (Khaleghi et al. 2020). The DLPFC is well described as a core region within the central executive network (Seeley et al. 2007; Sridharan et al. 2008), in line with the beneficial effects of NIBS in this region on working memory (Steenburgh et al. 2017), sustained attention (Ni et al. 2017), and behavioral inhibition (Sokhadze et al. 2009; D’Urso et al. 2015) of ASD patients. Electrophysiologically, NIBS is thought to modulate the imbalance of GABAergic inhibitory and glutamatergic excitatory transmitter systems in ASD (Casanova et al. 2006; Coghlan et al. 2012; Hull et al. 2017). It should be noted, however, that testing this hypothesis in vivo by combining TMS and magnetic resonance spectroscopy (MRS) has led to inconsistent results so far (Masuda et al. 2019; Bernardino et al. 2022), indicating that current models of transmitter imbalance in ASD could be oversimplified and may have varying implications for different brain circuits, i.e. involving processing of reward and social information. For example, fMRI studies in ASD have suggested altered processing of social cues in patients, involving the amygdala, the fusiform gyrus, the inferior frontal gyrus, and the pSTS (Whalen et al. 1998; Baron-Cohen et al. 1999; Critchley et al. 2000; Wang et al. 2004; Pinkham et al. 2007). Moreover, ASD patients show reduced activation in TPJ and DMPFC during social cognitive (e.g. mentalizing) tasks (Castelli et al. 2002; Lombardo et al. 2011; Philip et al. 2012; Kana et al. 2015). While the amygdala and the fusiform are located too deep below the skull for NIBS, the remaining cortical targets have been investigated by a few and more recent studies. For example, applying the excitatory iTBS on bilateral pSTS resulted in less compulsive behaviors and a trend for improved social communication (Ni et al. 2017). Accumulating evidence for the central role of the more dorsally located TPJ in both social cognition and ASD (Donaldson et al. 2015) has motivated a recently initiated trial in an envisaged cohort of 150 patients, applying iTBS over the right TPJ (Enticott et al. 2021). Another study aimed to modulate social cognitive processing via the frontal lobe, applying an excitatory 5 Hz rTMS protocol over the DMPFC (Enticott et al. 2014), which led to an improvement in social relatedness, involving social communication, social interaction, and social awareness. Unexpectedly, however, no significant improvements were found in the ability to mentalize. The authors discussed this null effect with the possibility that other mechanisms than mentalizing could lead to better social relating after NIBS or, offering an alternative explanation, that the experimental assessment of mentalizing may not have been sensitive enough to detect alterations after rTMS. This illustrates a challenge in the current state of research applying NIBS in ASD. While different neural systems are targeted with distinct hypotheses, outcome parameters are not yet harmonized. Table 2 illustrates that none of the behavioral outcome measures were consistently used across the six reviewed studies, ranging from broad clinical scores, such as the Autism-Spectrum Quotient, to more specific tests of functional domains, such as the Wisconsin Card Sorting Test probing executive functions not necessarily related to autism. The heterogeneous choice of outcome measures may contribute to the variability of findings, rendering a systematic comparison between studies impossible. While a consensus on using a common set of autism-related clinical scores may improve comparability, these tools may not optimally capture behavioral effects and thus may not allow early differentiation of responders versus non-responders to NIBS, when aiming to find an optimal stimulation strategy individually. One solution of this problem could be the implementation of automatized and time-efficient assessment tools, such as motion tracking, providing objective measures to quantify social behavior (Budman et al. 2019; Trujillo et al. 2021; Lahnakoski et al. 2022) as well as tasks based on computational models (Henco et al. 2020). To date, the longest monitored outcomes were reported by d’Urso et al. (2015), who observed improvements in the ABC checklist 1 week after stimulation, and by Enticott et al. (2014), reporting that improvements in the social relatedness subscore of the RAADS sustained until 1 month post-intervention. In summary, three anatomically dissociable neural systems associated with (i) central executive function, (ii) language, and (iii) social cognition have been of interest for NIBS studies in ASD. Assuming that these systems specifically map to symptoms in ASD, future trials may combine NIBS with behavioral training to enhance therapeutic effects and monitor outcomes longitudinally. Yet, the current data reviewed here do not sufficiently support the assumption that symptoms in ASD can be mapped to dissociable neural systems. Consequently, future trials are needed to systematically test this notion by including two different systems (e.g. DLPFC and DMPFC or anterior and posterior TPJ), while investigating how NIBS alters two different symptoms (e.g. related to executive function and social cognition). Such findings would be of clinical interest, suggesting that targets for NIBS may be defined based on the individual burden of symptoms.
Conclusion
Reviewing previous clinical studies using NIBS in adolescent and adult patients with ASD generally suggests that changes in social behavior might be induced by both rTMS and tDCS. The reviewed studies indicate that functional domains could be modulated in ASD. For example, executive function and stereotypical behaviors could be improved by modulating DLPFC excitability, whereas social cognitive function was improved by modulation of DMPFC. Furthermore, examinations are underway to examine neuromodulatory effects in the TPJ, which plays a critical role in both language and social cognition, two highly relevant functions for persons with ASD. To date, the relatively small sample sizes, inconsistent experimental control conditions, and outcome parameters of the reviewed studies do not allow any conclusive verdict about the consistency of effects. The fact that only six studies with relatively small cohorts have been published also raises the question of potential obstacles hindering neuromodulation from being translated into clinical practice. As such, we discussed the variability of neurophysiological effects due to individual anatomy and the uncertainty of target regions in relation to behavioral outcome measures. The use of functional assessment of brain activity and electrical field simulations based on structural MRI may reduce the variability of effects and thereby increase the efficacy of NIBS in ASD, calling for further investigations in randomized controlled trials.
Author contributions
Lukas Hensel (Conceptualization, Visualization, Writing—original draft, Writing—review & editing), Jana Lüdtke (Conceptualization, Data curation, Methodology, Writing—original draft, Writing—review & editing), Katia O. Brouzou (Validation, Writing—review & editing), Simon B. Eickhoff (Conceptualization, Funding acquisition, Writing—review & editing), Daniel Kamp (Conceptualization, Data curation, Supervision, Writing—review & editing), and Leonhard Schilbach (Conceptualization, Funding acquisition, Writing—review & editing).
Funding
Funding was provided via a grant by the Forschungskommission of the Medical Faculty at the Heinrich-Heine-University to LS and SBE.
Conflict of interest statement: None declared.
References
Author notes
Lukas Hensel and Jana Lüdtke contributed equally to this work.