-
PDF
- Split View
-
Views
-
Cite
Cite
Cristian Morales, Juan Facundo Morici, Nelson Espinosa, Agostina Sacson, Ariel Lara-Vasquez, M A García-Pérez, Pedro Bekinschtein, Noelia V Weisstaub, Pablo Fuentealba, Dentate Gyrus Somatostatin Cells are Required for Contextual Discrimination During Episodic Memory Encoding, Cerebral Cortex, Volume 31, Issue 2, February 2021, Pages 1046–1059, https://doi.org/10.1093/cercor/bhaa273
- Share Icon Share
Abstract
Memory systems ought to store and discriminate representations of similar experiences in order to efficiently guide future decisions. This problem is solved by pattern separation, implemented in the dentate gyrus (DG) by granule cells to support episodic memory formation. Pattern separation is enabled by tonic inhibitory bombardment generated by multiple GABAergic cell populations that strictly maintain low activity levels in granule cells. Somatostatin-expressing cells are one of those interneuron populations, selectively targeting the distal dendrites of granule cells, where cortical multimodal information reaches the DG. Nonetheless, somatostatin cells have very low connection probability and synaptic efficacy with both granule cells and other interneuron types. Hence, the role of somatostatin cells in DG circuitry, particularly in the context of pattern separation, remains uncertain. Here, by using optogenetic stimulation and behavioral tasks in mice, we demonstrate that somatostatin cells are required for the acquisition of both contextual and spatial overlapping memories.
Introduction
Episodic memory is made up of a collection of different events which may contain overlapping information. The capacity to discriminate similar memory episodes, which could otherwise be confused, is critical for correct encoding (Colgin et al. 2008), effective retrieval (Dickerson and Eichenbaum 2009; Hulbert and Norman 2015), and avoiding catastrophic interference (Mcclelland and Goddard 1997). Accordingly, disruptions of memory discrimination have been related to cognitive impairment in aging and neuropsychiatric disorders, such as depression or posttraumatic stress disorder (Holden and Gilbert 2012; Kheirbek et al. 2012; Leal and Yassa 2018). In addition, conditions such as aging and epilepsy exhibit episodic memory deficits as very common symptoms, also showing disrupted pattern separation (de Lanerolle et al. 1989; Holden et al. 2012; Reyes et al. 2018). Computational models suggest that pattern separation ought to be implemented for efficient discrimination of overlapping memories, by the transformation of similar patterns of inputs into segregated synaptic outputs (McClelland et al. 1995; O'Reilly and McClelland 1994; Treves and Rolls 1994). Abundant evidence from animal experiments support the hippocampus is one of the brain regions where pattern separation is implemented, in particular the dentate gyrus (DG), which allows the discrimination of overlapping spatial representations (Gilbert et al. 1998; Clelland et al. 2009; Bekinschtein et al. 2013) and similar contextual fear conditioning memories (McHugh et al. 2007).
It has been reported that the activity patterns of subsets of granule cells in the DG represent spatial and contextual aspects of a memory episode (Ramirez et al. 2013, 2014), with similar episodes of experience being stored into distinct nonoverlapping activation patterns in a process called orthogonalization (Leutgeb et al. 2007; Deng et al. 2013; Neunuebel and Knierim 2013), that is essential for pattern separation. Granule cells conform a large neuronal population that remains sparsely active due to strong inhibitory control provided by local GABAergic inputs (Jung and Mcnaughton 1993; Nitz and Mcnaughton 2004; Danielson et al. 2016). The tight regulation of granule cell excitability is critical (Rolls and Kesner 2006; Rolls 2013), as evidenced by deteriorated pattern separation when the spiking activity of granule cells is experimentally enhanced (Jinde et al. 2013) or when tonic inhibition is decreased. Moreover, tonic inhibition of DG granule cells is important for the control of memory interference (Engin et al. 2015). The most significant inhibitory inputs to granule cells arise locally from parvalbumin (PV)-expressing cells that provide massive synaptic inhibition to the perisomatic region (Kepecs and Fishell 2014) and tightly control the spike timing of granule cells (Hu et al. 2014). In addition, somatostatin-expressing neurons (SOM), mostly located in the hilus (Freund and Buzsáki 1996; Yuan et al. 2017), densely innervate the distal dendritic arbor of granule cells (Halasy and Somogyi 1993; Yuan et al. 2017). The dendritic arbor of granule cells is largely distributed across the molecular layer and is impinged by entorhinal inputs massively carrying contextual information (Yuan et al. 2017). Thus, SOM are good candidates to control the excitatory inputs reaching distal dendrites, yet they provide slow, weak, and unreliable inhibition upon postsynaptic targets, including granule cells (Espinoza et al. 2018) and other interneuron types (Savanthrapadian et al. 2014). Thus, the role of SOM in controlling the flow of information in the DG circuitry remains elusive. Recent experiments have started to change this view and established the relevance of SOM in the control of excitability of granule cells (Savanthrapadian et al. 2014; Hofmann et al. 2016) and the size of neuronal ensembles during memory encoding in vivo (Stefanelli et al. 2016). Taken together, these findings suggest that SOM are ideally positioned to participate in contextual discrimination, possibly through the regulation of pattern separation by controlling the dendritic excitability of granule cells (Goldberg and Coulter 2013). Hence, we tested the hypothesis that functional DG SOM are required for contextual discrimination through the control of granule cells excitability. We found that optogenetic suppression of SOM in the DG modulated the firing rate of putative excitatory cells and putative PV interneurons. Moreover, optogenetic stimulation impaired both contextual and spatial discrimination of overlapping recognition memories during task acquisition. Our results suggest that SOM are required for successful pattern separation during episodic memory encoding.
Materials and Methods
Mice were housed at 12 h light/dark cycle at 23°C with food and water ad libitum. Experiments took place during the light phase of the cycle (lights on at 8 AM) in a quiet room located inside the animal facility with dim light. The experimental protocol for this study was approved by the National Animal Care and Use Committee of the Catholic University of Chile and Favaloro University of Argentina. All experiments were performed on adult (8–30 weeks old) mice.
All experimental procedures were in accordance with institutional regulations of Institutional Animal Care and Use Committee of the Favaloro University, ASP # 49527/15, Argentinian government regulations (SENASAARS617.2002) and in accordance with Comite Etico Cientifico para el Cuidado de Animales y Ambiente (ID 151223006 and CEBA 13–014) of the Pontificia Universidad Catolica de Chile. All efforts were made to minimize the number of animals used and their suffering.
Experimental Animals
For the electrophysiological and optogenetics experiments, three strains of mice were used, C57Bl/6, Ai39 (RCL-eNpHR3.0/EYFP), and Sst-IRES-Cre. All transgenic lines were obtained from Jackson laboratories (www.jax.org). We used these strains as controls and refer to them as NpHR− animals throughout the text. Double transgenic animals were obtained from the breeding of SST-IRES-Cre+/+ and Ai39+/− mice, so that they expressed functional Natronomonas pharaonis halorhodopsin (NpHR) exclusively in somatostatin cells. We refer to such animals as NpHR+ throughout the text. For the pharmacological experiments, we use wild-type C57Bl/6 mice from the Pharmacy and Biochemistry School, University of Buenos Aires, Argentina. A total of 95 mice (70 male, 25 female) were used for experiments. We pooled data between female and male mice as discrimination was not significantly different (Unpaired Student’s t-test, t = 0.9290, P = 0.3712).
In Vivo Electrophysiological Recordings Under Anesthesia
Anesthesia was induced with isoflurane 4% followed by an intraperitoneal injection of urethane (0.8 g/kg). Animals were left to rest for 20 min and placed in a homeothermic blanket that maintained the body temperature at 37° throughout the experiment. After 20 min, an intraperitoneal injection of ketamine/xylazine (40/4 mg/kg) was applied. When mice exhibited no reflexes, they were put into a stereotaxic frame. The skin above the skull was surgically removed and a small craniotomy (1 mm) was drilled above the cortex (coordinates 1.5 mm lateromedial, 2.0 anteroposterior, Franklin and Paxinos 2007). In addition, a customized support bar was glued to the skull to release pressure from the ears and mouth, and hold the animal’s head in position for recordings. At this time, an intraperitoneal cannula was placed to deliver supplementary doses of urethane each 20 min (1/12 of the initial volume). After removing the dura over the cortex, the electrode array was placed on the cortex and carefully descended to the DG. In our experiments we used commercial optrodes, the A1x32-Poly2 and A1x32-Poly3 (NeuroNexus) probes. Both of them had one shank and an optical fiber glued 100 μm above the most superior recording site. The Poly2 shank had recording sites distributed in two columns covering a total length of 700 μm. The Poly3 shank had recording sites on three columns with a full length of 600 μm. In both optrodes, the distance between the nearest recording sites was 50 μm approximately.
Surgery for Chronic Implantation and Optogenetic Stimulation
Mice were anesthetized with isoflurane (4% induction, 1.5–2% maintenance) and when exhibited no reflexes were placed on a stereotaxic frame. Temperature was kept at 37° throughout the procedure (1–2 h) using a homeothermic blanket. The skin was incised to expose the skull. Three or two craniotomies were made with a dental drill for anchoring screws. Additionally, another craniotomy (~1 mm) was made above the DG bilaterally (anteroposterior −2 mm, mediolateral ±1.5 mm from Bregma). Two optic fibers (diameter 200 μm) glued to ceramic ferrules (diameter 230 μm) were descended through both craniotomies until reaching the DG and fixed in position using dental acrylic. After surgery, mice received a daily dose of enrofloxacin for 3 days and supplementary analgesia with ketoprofen for 3 days. After surgical implantation, we daily controlled the weight of each animal and allowed it to stabilize to at least 90% of the initial ad libitum weight. This procedure took 5–7 days after surgery in most cases. In addition, animals were closely supervised by a veterinary at the animal facility, who helped us to evaluate other physiological parameters such as state of the surgical wound, spontaneous and provoked behavior, general mobility, body posture, eye secretions, fur condition, etc.
Surgery and Drug Infusions for Pharmacological Experiments
For pharmacological experiments, mice were deeply anesthetized with ketamine/xylazine (150/6.6 mg/kg) and placed in a stereotaxic frame. The skin was incised to expose the skull. Small craneotomy holes were then drilled and a set of 23 G guide cannulae (0.5 cm long) were implanted bilaterally over the DG (anteroposterior −2 mm, mediolateral ±1.5 mm from bregma). Cannulae were fixed to the skull with dental acrylic. At the end of surgery, animals were injected with a single dose of meloxicam (0.33 mg/kg) as analgesic and gentamicin (5 mg/kg) as antibiotic. Behavioral procedures were started 5–7 days after surgery. Infusions were made using a 30 G injection cannula connected to a 10 uL Hamilton syringe. Infusions were made on the training day or test day. Cannulated mice received bilateral 0.3 uL infusions of DNQX or vehicle into the DG 15 min before each training or test session. DNQX was diluted in physiologic solution to a final concentration of 1.89 ug/uL.
Optogenetic Stimulation
For chronic implants, optogenetic stimulation of DG somatostatin interneurons was achieved with a 200 μm optic fiber (N.A. 0.37) coupled to a green laser (532 nm, Laserglow Techonologies) that provided a total light power of 0.1–60 mW at the fiber tip. Light stimuli consisted of 5 s light pulses each 20 s, and power at the tip of the fiber was set between 5–15 mW.
For acute recordings, optogenetic stimulation was achieved with an optrode, which consisted of an optic fiber (100 μm, N.A. 0.22) attached to an array of electrodes, so electrical recordings and optical stimulation could be performed simultaneously on the same site. The dentate spike was used as a physiological marker to locate the polymorphic layer, as it has been reported that its amplitude is highest in the hilus (Bargin et al. 1995; Penttonen et al. 1997; Senzai & Buzsáki, 2017). To confirm the correct positioning in the hilus, we placed the tip of the optical fiber approximately 400 μm over the site where we recorded the largest amplitude dentate spikes (Supplementary Figure S1). Moreover, light stimuli consisted of 5 s light pulses every 20 s and power at the tip of the fiber was set between in 5–12 mW. It has been reported that somatostatin cells increase their firing rate in novel environments and participate in processing contextual information (Stefanelli et al. 2016). Further, the DG is very active during spatial representation (Bekinschtein et al. 2013), opening the possibility that somatostatin cells may also participate in spatial memory formation. Since the precise dynamics and timing of contextual, spatial and novelty processing is not well understood, we decided to suppress somatostatin cells during the entire exploration epoch. Importantly, we did not want to maintain continuous laser stimulation as it 6 produces temperature changes in the neural tissue and potentially long-term circuit modifications (Arias-Gil et al. 2016; Picot et al. 2018; Peixoto et al. 2020). For this reason, we decided to use prolonged laser pulses, with long silent intervals to allow recovery of neural circuitry dynamics. Furthermore, we have previously used the same protocol to silence somatostatin neurons in subcortical regions and have been able to assess both neural activity and behavioral performance (Espinosa et al 2019a, 2019b).
Unit Crosscorrelation Analysis
Neural activity of DG was crosscorrelated with the light pulse. A time window of ±15 s was defined with point zero assigned to the light onset. The timestamps of the spikes within the time window were considered as a template and were represented by a vector of spikes relative to t = 0 s, with a time bin of 200 ms and normalized to the basal firing rate of hippocampus neurons. Thus, the central bin of the vector contained the ratio between the number of neural spikes elicited between ±100 ms and the total number of spikes within the template. Next, the window was shifted to successive light pulses throughout the recording session, and an array of templates was obtained. Then, we calculated the z-score of this crosscorrelogram using the mean and standard deviation obtained, bin-to-bin, from the distribution of 1000 shuffled crosscorrelograms. We classified as excited units, those that presents more than 4 bins with Z-score larger than 3 during laser presentation. Similarly, we classified as inhibited units, those that presents more than 4 bins with z-score more negative than −3 during laser presentation.
Identification of Putative Neuron Types
We defined laser-inhibited units as somatostatin cells. To identify different types of units within excited cells we adapted a previous analysis (Senzai and Buzsáki 2017). Specifically, the trough-to-peak latency and burst index allows to distinguish between glutamatergic cells and GABAergic interneurons, as glutamatergic neurons have longer trough-to-peak latency and higher burst index than GABAergic interneurons. We performed a similar analysis for units that were responsive to laser stimulation. To measure the trough-to-peak, we calculated, for the mean waveform of each detected unit, the temporal difference between the minimum voltage and the maximum voltage (between the minimum and the end of the waveform). To calculate the burst index, first, we calculated the autocorrelogram of the timestamps of each unit outside laser presentation (i.e., baseline activity); second, we computed the ratio between the peak of the autocorrelogram in the central bins (−1–6 ms) and the mean value of the autocorrelogram (200–300 ms); finally, we calculated the logarithm of such ratio. This allowed us to construct a bidimensional vector for excited units. Then, we used hierarchical cluster analysis to discriminate different type of units. The hierarchical cluster analysis showed three types of cluster, two of them were merged in a new cluster because both had similar properties when were compared with somatostatin interneurons. Finally, we compared the trough-to-peak latency and burst index among somatostatin and two clusters were obtained.
Detection of Dentate Spikes
Based on LFP activity, we identified the electrodes located in the hilus and filtered activity between 100 and 249 Hz. Then, we calculated the z-score of the signal using the mean and the standard deviation of the entire LFP recording. Finally, we selected high-frequency events based on amplitude, with 5–7 SD threshold.
Arenas and Objects Used in Behavioral Tests
Identical copies of objects made from plastic, glass, or aluminum were used. The height of objects ranged from 4 to 6 cm. All objects were affixed to the floor of the apparatus with an odorless reusable adhesive to prevent them for being displaced during exploration. Objects had no natural relevance for mice as they were not associated with reinforcement. The objects, floor, and walls were cleaned with ethanol 10% between sessions. Since no differences were observed in behavioral performance during the experiments between sexes, we pooled animals depending on the genotype or treatment received.
Object-in-Context Task
Four different contexts were used for these experiments. In the dissimilar condition, a rectangular and triangular arenas were used. Both had homogenous gray walls constructed from opaque foam board. The rectangular apparatus was 40 cm × 25 cm length × 30 cm high, while the triangular one was 40 cm × 25 cm length × 30 cm high. Both contexts had the same surface area to avoid differences due to the size of the arena. Contextual cues were geometric shapes of different colors. In the similar condition, we used two rectangular arenas made of white opaque foam board. The measures of these arenas were identical to those used for the rectangular arena of the dissimilar condition. Then, the cues presented in both contexts were different in shape but same sizes and colors.
Spontaneous Location Recognition Task
A circular maze of 40 cm diameter with walls 40 cm high was used. Both floor and wall were gray. Three spatial clues were glued at 15 cm over the floor. A video camera and laser cable were mounted above the maze.
Object-in-Context Task
This behavioral test is composed of three phases that allows the evaluation of the congruency between pairs of context and object (Wilson et al. 2013). During the training phase, animals were exposed to two different object-context associations. These two training sessions were 1 h apart. The test session was carried out 24 h after the training 2. During this phase, a new copy of each of the objects used during the training phase was presented in one of the arenas. The context to be used during the test phase was randomly selected, preserving similar total proportions. The selection of the context to be used during the test phase was pseudorandomly assigned. Thus, one of the objects was presented in contextual miss-match, the incongruent object, whereas the other object was not, the congruent object. In this task, novelty arises from the novel combination of object and context. Then, exploratory behavior should be driven by retrieval of a particular conjunctive representation of object and context (Eacott and Norman 2004; Morici, Ciccia, et al. 2015).
Habituation Sessions
These sessions were conducted to familiarize animals with the procedure of being exposed to an environment where they could encounter novel objects. On the first day, mice were handled and placed in a circular context and allowed to explore for 10 min. Thirty minutes later, they were reintroduced in the arena, yet in this case two different objects were placed in the arena. Mice were allowed to freely explore for 5 min.
Training Sessions
On the first training session, two identical objects (A1 and A2) were placed into one of the arenas (context 1). Animals were placed into the arena and allowed to freely explore the environment for 10 min. At the end of the session, animals were returned to their home cage. After 1 h, animals were exposed to a second object-context association, different from the first one. For that, mice were placed in a different context (context 2), in which a second pair of objects was present (B1 and B2). Arenas were pseudo-randomly assigned as context 1 or 2.
Test Session
During this session, animals were re-exposed to previously familiar context-object pairs for 5 min. Mice were reintroduced to context 1 or context 2 where they could explore one copy of object A and one copy of object B. Then depending on the context in which this phase takes place, one of the objects presented will be contextually congruent while the other will be contextually incongruent.
Spatial Location Recognition Task
This behavioral paradigm, as the object-in-context, was comprised by three phases that allowed for the evaluation of spatial location novelty detection (Ennaceur et al. 1997; Warburton et al. 2000). During training sessions, animals were exposed to three identical objects placed into a circular arena and were allowed to explore them. The separation angle between two of those objects was manipulated in order to generate conditions with variable levels of spatial-location similarity. During the test session, one of the objects was placed in a familiar location, while another copy of the same object was placed in a new location at the middle point between the two previous objects’ locations. The rationale behind the task was that if mice were able to discriminate the two similar spatial locations, their representations should be distinct and resilient to confusion. Thus, mice should show preference to explore the object presented in the novel position during the retrieval phase. However, if the representations of the two similar locations were not sufficiently segregated, then mice should behave as if the new location was familiar.
Habituation Sessions
These sessions were conducted to familiarize animals with the procedure of being exposed to an environment where they could encounter novel objects. During these sessions, animals had the opportunity to generate a spatial map of the environment. To that end, mice were repeatedly exposed to the environment for 10 min during four consecutive days.
Training Session
During this session, animals were placed in the circular arena where three identical objects had been previously positioned. The angle between two of the objects was changed depending on the variation in use (large, 180°; medium, 120°; or small, 50°). Mice were then allowed to explore the environment for 10 min.
Test session. In this session only 2 copies of the previously presented objects were placed in the arena. One of the objects was located in the same position as before, yet the other object was placed in the intermediate position occupied by the two objects in the previous session. This session lasted 5 min.
This task is a slightly modified version of a previously developed task that was validated in rats (Bekinschtein et al. 2013). It was specifically designed to parametrically control the load of pattern separation. In the original article, the first experiment controlled for the possibility that a change in the number of objects was not driving novelty-related exploration (Bekinschtein et al. 2013). With that, the task controls for the change in the number of objects between sessions.
Behavioral Analysis
For each behavioral session, we quantified the exploration time of each object. For the test phase, we analyzed the exploration time for every copy of the object using a Matlab plug-in (ID tracker). For the training phase, manual score was performed. For the object-in-context test session, we calculated the discrimination index (DI) as tincongruent−tcongruent/ttotal exploration of the session. For the spontaneous location recognition test, the DI was calculated as tnovel-position– tfamiliar-position/ttotal exploration. For all experiments, object exploration was defined as the mouse having its nose directed to the object and located at 2 cm or less. Climbing over or sitting on the objects was not considered as exploration. Two persons scored independently the videos; one of them was blind to all conditions.
Statistical Analysis
Statistical analyses were performed with GraphPad 6.01 and Matlab. We used parametric analysis depending when data distributed normally. Electrophysiological data were analyzed using Kruskal–Wallis followed by Tukey–Kramer multiple comparison test. Behavioral data were analyzed using two-tailed Student’s t-test or two-tailed Wilcoxon test. For comparisons between two repeated-measured groups two-tail paired Student’s t-test or two-tailed paired Wilcoxon test were used. For more than three groups, we performed One-way ANOVA followed by Tukey’s post-hoc test or Kruskal–Wallis test followed by Dunn’s post-hoc test. Two-way ANOVA followed by Tukey’s posttest was used when three or more groups were involved. In all cases, P-values were considered statistically significant when smaller than 0.05. All data are presented as the mean ± S.E.M.
Histology and Immunocytochemistry
At the end of electrophysiological recordings and behavioral testing, mice were euthanized with a high dose of anesthetic (ketamine 200 mg/kg, xylazine 20 mg/kg) and intracardially perfused with saline followed by 20-min fixation with 4% paraformaldehyde. Brains were extracted and postfixed in paraformaldehyde for a minimum of 24 h before being transferred to PBS azide and sectioned coronally (70–100 μm thickness). Sections were further Nissl-stained. Location of electrode shanks and optical fibers were determined in reference to standard brain atlas coordinates (Franklin and Paxinos 2007) under a light transmission microscope.
Spike Sorting
Semiautomatic clustering was performed by KlustaKwik (Harris et al. 2000). This method was applied over the 32 channels of the silicon probe, grouped in 8 pseudo-tetrodes of 4 nearby channels.
Results
Optogenetic Suppression of Putative Somatostatin Cells Disrupts DG Neuronal Firing Patterns and Trigger Dentate Spikes Under Anesthesia
In order to establish the effect of locally inhibiting SOM on the DG network, we stereotaxically implanted an optrode covering the entire dorsoventral extension of the DG (Supplementary Figure S2) in anesthetized double transgenic mice that selectively expressed the inhibitory halorhodopsin pump (NpHR+) in SOM (Espinosa et al. 2018, 2019). To ensure the simultaneous recording of all 3 DG laminae and optical suppression of hilar SOM (Freund and Buzsáki 1996), the tip of the optical fiber was positioned close to the hippocampal fissure (Fig. 1A). Next, we delivered prolonged laser pulses to achieve maximal optogenetic inhibition, reproducing previous experimental protocols (Espinosa et al. 2018, 2019). Control experiments showed that effects were selective for transgenic NpHR+ animals, with little effect on control (NpHR-) mice (Supplementary Figure S2).
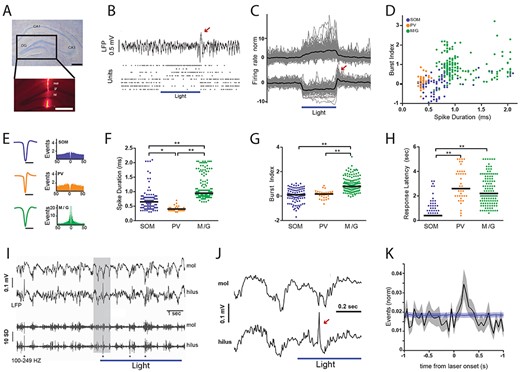
Optogenetic inhibition of putative hilar somatostatin cells disrupts local network activity patterns in the dentate gyrus under anesthesia. (A) Nissl-stained coronal brain section of dorsal hippocampus showing different regions, CA1, CA3, and DG. Scale bar, 500 μm. Inset, fluorescence micrography of the DG with optrode track stained with DiI. mol, molecular layer; gr., granular layer; hil., hilus. Scale bar, 500 μm. (B) Field potential and unit activity from the DG shown in (A). Horizontal bar depicts laser stimulation (5 s-pulse delivered every 15 s, 12 mW, fiber diameter 100 μm). Top, LFP (filtered 1 Hz–4 kHz); bottom, raster plots for eight simultaneously recorded cells. Note that during laser stimulation the four upper units increased their firing rate whereas the four lower units decreased their activity. Red arrow in (B) and (C) depicts rebound activity at the end of the laser pulse. (C) normalized discharge probability of responsive units during laser stimulation. Horizontal bar depicts laser stimulation (5–12 mW). Top, excited units (average increase = 12%, n = 203). Bottom, inhibited units (average decrease = 6.3%, n = 111). Black line, population average; gray lines, individual units. (D) Scatter plot showing the distribution of spike duration (trough-to-peak latency) and burst index for all laser-responsive units. (E) Average waveforms and autocorrelograms for neuronal populations shown in (D). (F) Comparison of the spike duration between unit clusters. Lines, population median; points, individual units. Kruskal–Wallis test, P = 4.95 × 10−37; Tukey–Kramer multiple comparison test, *P = 3.48 × 10−5; **P = 9.56 × 10−10. (G) Comparison of burst index between unit clusters. Kruskal–Wallis test, P = 2.82 × 10−32; Tukey–Kramer multiple comparison test, **P = 9.56 × 10−10. Lines, population median; points, individual units. (H) Comparison of response latency between unit clusters. Kruskal–Wallis test, P = 1.12 × 10−37; Tukey–Kramer multiple comparison test, **P = 9.56 × 10−10. Lines, population median; points, individual units. (I) top traces show examples of molecular and hilar LFP activity. Bottom traces show the same periods filtered for high-frequency activity (100–249 Hz). Asterisks depict dentate spikes. Shaded gray region is expanded in the next panel (J). (J) Arrow depicts an example dentate spike. (K) Crosscorrelogram between laser onset and dentate spikes. Black line, data average (n = 34 recording sites); gray area, data standard error; blue line, shuffling average (1000 iterations), blue area, shuffling standard error. Note significant increase in the relative density of dentate spikes during the first part of optical stimulation.
Overall, we found a small proportion of units changing their firing patterns upon laser stimulation in NpHR+ mice. A minor fraction of units (6.3%, n = 111) robustly decreased (median = 57%, IQR = 55%) their activity relatively fast (median = 400 ms, IQR = 200 ms). Hence, such neuronal population was defined as SOM (Fig. 1C). Another small group of cells (12%, n = 203) showed a significant increase in firing rate (median = 87%, IQR 127%), presumably by synaptic disinhibition, with significantly slower kinetics than SOM (median = 2.4 s, IQR = 2 s, P < 0.001, Fig. 1C,Supplementary Figure S2). DG units have been previously classified as glutamatergic or GABAergic based on their in vivo spike duration and bursting patterns (Senzai and Buzsáki 2017). Indeed, excitatory cells, such as mossy cells and granule cells exhibit longer trough-to-peak latency and higher burst index than GABAergic interneurons (Senzai and Buzsáki 2017). Thus, we carried out a similar analysis. We first plotted all laser-responsive units in a bidimensional space comprised by spike duration and burst index (Fig. 1D). By definition, SOM comprised one cluster given their common physiological characteristic of optogenetic inhibition (Fig. 1D and E). Within the optically excited units, we recognized two different populations. We considered one group of excited units as putative PV cells because of their short spikes, significantly more rapid than the other groups (median = 0.4 ms, IQR = 0.1 ms, P < 0.001; Fig. 1F). Such short spike duration has been well documented in GABAergic PV cells (Lübke et al. 1998; Hosp et al. 2014; Senzai and Buzsáki 2017) that are synaptically targeted by SOM (Savanthrapadian et al. 2014b). On the other hand, the other cluster of excited units was consistent with the presence of mossy cells and granule cells (M/G). Indeed, M/G units had slower spikes (median = 0.95 ms, IQR = 0.34 ms, P < 0.001; Fig. 1F) and higher burst index (median = 0.8, IQR = 0.6, P < 0.001; Fig. 1G) than either PV cells or SOM. Furthermore, there was no significant difference in response latency to optogenetic stimulation between PV cells and M/G units (Fig. 1H), but they were both significantly slower than SOM (median = 0.4 s, IRQ = 0.2 s, P < 0.001; Fig. 1H). These results suggest that a small proportion of both PV cells and M/G units was probably disinhibited upon optogenetic suppression of SOM.
Next, we aimed to evaluate the effect of the modulated synaptic output of SOM onto DG oscillatory activity. We noted that at the end of laser pulses a prominent deflection was apparent in the DG field potential (Fig. 1B) that was probably the result of rebound activity in SOM (Fig. 1C,Supplementary Figure S3). A similar effect has been previously described in the dorsal CA1 area (Royer et al. 2012). We then quantified the spectral distribution of DG activity. The Locald field potentials (LFP) frequency spectrum showed a prominent shoulder in the gamma range (30–80 Hz) which then decayed with the characteristic 1/f distribution at higher frequencies (Freeman et al. 2000, Supplementary Figure S4). Interestingly, optogenetic suppression of SOM selectively decreased power in the high-frequency range (100–250 Hz, Supplementary Figure S4). Dentate spikes are hallmark population patterns exclusive of the DG, which due to their large-amplitude, short-duration can be detected in the high-frequency activity range (Bragin et al. 1995). Indeed, high-pass filtering LFP recordings, particularly in the hilar region, evidenced the presence of prominent dentate spikes (Fig. 1I and 1J). Dentate spikes result from massive dendritic depolarization and perisomatic inhibition of granule cells (Bragin et al. 1995; Penttonen et al. 1997). Given that optogenetic suppression of SOM increased the activity of putative granule cells and PV interneurons, that inhibit the perisomatic region of granule cells (Freund and Buzsáki 1996), we reasoned that during optical stimulation the incidence of dentate spikes was likely to increase. Indeed, crosscorrelation analysis between laser pulses and dentate spikes showed a significant increase in their probability of occurrence (Fig. 1K). Similarly, their incidence increased when comparing the periods before and after the onset of laser stimulation (Supplementary Figure S4). Hence, the inhibition of SOM may disinhibit PV interneurons and granule cells, with the concomitant increase of network excitability, reflected in the elevated density of dentate spikes. Altogether, our results suggest that optogenetic inhibition of SOM disrupts local network activity patterns in the DG.
Encoding of Overlapping Contextual Memories Requires Functional Glutamatergic Transmission and Somatostatin Cells in the DG
Fully functional granule cells are necessary for the discrimination of similar contexts (McHugh et al. 2007; Danielson et al. 2016), as well as the acquisition of novel information. However, the role of other DG cell-types is not so well established, particularly interneuron populations that regulate the spike timing of granule cells. Hence, we investigated the contribution of hilar SOM to behavioral performance in context-dependent memory tasks. To test this idea, we developed a variation of the object-in-context task (Eacott and Norman 2004; Wilson et al. 2013; Morici et al. 2015; Morici et al. 2015b; Morici et al. 2018). We reasoned that discrimination of the novel object-context pairing should recruit the DG only when contextual configurations were overlapping. Accordingly, we manipulated contextual information by training animals in the object-in-context task using similar or dissimilar contexts (Fig. 2A,Supplementary Figure S5). First, we evaluated whether the DG was engaged in contextual memory encoding in this test. For this, we blocked glutamatergic transmission during memory encoding by infusing DNQX into the DG preceding every training session. Regardless of the object-context configuration, blockade of AMPA receptors did not affect exploratory behavior during training sessions (Supplementary Figure S6). Consistent with our hypothesis, blocking DG excitatory transmission obliterated discrimination during the test only when contexts were similar (mean = 0.013, SEM = 0.012, P < 0.001; Fig. 2C, E,Supplementary Figure S7). Importantly, the experimental manipulation did not affect total exploration time (Fig. 2D, F) suggesting that these results were not due to changes in motivation, awareness, or exploratory behavior in general. Together, these results suggest that glutamatergic transmission in the DG is necessary for the encoding of object-in-context memories only when the contextual information presented is similar, consistent with the observation that pattern separation is engaged only when overlapping spatial representations ought to be discriminated (Gilbert et al. 1998, 2001).
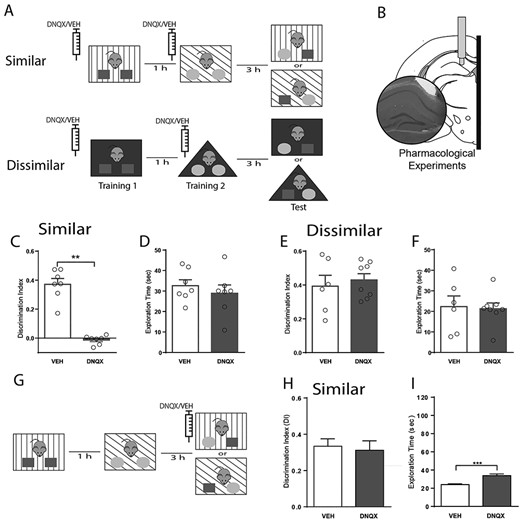
Encoding, but not retrieval, of overlapping contextual memories requires functional glutamatergic transmission the DG. (A) Schematic representation of the two versions of the object-in-context behavioral test used to assess context discrimination. Pharmacological experiments were performed by locally injecting either DNQX (1.89 μg/μL, 0.3 uL) or vehicle (veh., saline 0.3 μL) preceding every training session, when memory was encoded. (B) Anatomical representation of the hippocampus locations targeted during pharmacological experiments. Inset, histological verification was performed with cresyl violet staining. DI accumulated over the entire behavioral test (5 min) for animals during pharmacological probing for both versions of the behavioral test, similar (C) and dissimilar (E). Note a significant effect only in the similar condition. Total exploration time accumulated over the entire behavioral test (5 min) for animals during pharmacological probing was not affected during either the similar (D) or dissimilar (F) condition. Data are represented as population mean ± SEM and individual animals (circles). Total exploration time during the training phase. (G) Schematic representation of the object-in-context behavioral test used in the similar condition to test memory retrieval. (H) DI during the retrieval phase. (I) Total exploration time during the retrieval phase. Unpaired Student’s t-test; 2C, **P = 8.74 × 10−7; 2D, P = 0.56; 2E, P = 0.61; 2F, P = 0.43; 2H, P = 0.74; 2I, *P = 0.0008.
It has also been proposed that the DG is recruited during the retrieval of similar representations (Denny et al. 2014; Krasne et al. 2015; Bernier et al. 2017). Therefore, we manipulated glutamatergic transmission in the DG during memory recall. For that purpose, we performed the object-in-context task in the similar condition and infused DNQX into the DG 15 min before the test session, when memory is retrieved (Fig. 2G). In this condition, we did not detect any effect of the drug on the cumulative DI (Fig. 2H) or during the entire experimental session (Supplementary Figure S7). Moreover, total exploration time was not different between groups (Supplementary Figure S8). Interestingly, blocking AMPA receptors influenced behavior as exploration times were higher for drug-infused animals (mean = 33.83 s, SEM = 1.95 s, P < 0.001; Fig. 2I). We reasoned that the effect observed could result from a generalized increase in locomotor activity rather than a specific increase in object exploration. Indeed, we found that the total ambulatory distance was also augmented upon drug injection (meanVEH = 9.18 ± 1.56 mt, N = 6; meanDNQX = 15.89 ± 1.34 mt, N = 5; unpaired t-test. P = 0.0055, t = 3.631).
Importantly, both control (vehicle) and experimental (DNQX) groups explored significantly more the incongruent object than the congruent object (2-way ANOVA, Pinteraction = 0.8047, F (1,9) = 0.06483; Pdrug = 0.0947, F (1,9) = 3.488; Pobject < 0.0001, F(1.9) = 83.84) supporting the hypothesis that DNQX might increase general exploratory behavior without affecting the discrimination capacity when infused preceding the test. Hence, this result suggests that the DG is actively engaged during encoding of similar contextual representations, but not during their retrieval.
Recent evidence suggests that SOM are recruited during fear memory formation (Lovett-Barron et al. 2014; Stefanelli et al. 2016) and may participate in pattern separation by controlling the size of engrams encoding spatial representations (Freund and Buzsáki 1996; Hargreaves et al. 2005; Stefanelli et al. 2016). Consequently, we conducted the object-in-context task in our transgenic mice expressing functional halorhodopsin (NpHR+) in SOM (Espinosa et al. 2018). We bilaterally implanted optic fibers into the DG (Fig. 3B) in order to activate NpHR during the training sessions (Fig. 3A). Laser stimulation did not affect the distribution of object exploration times during training sessions for neither task version (Fig. 3D, F,Supplementary Figure S6). Nonetheless, optogenetic inhibition of SOM during the training session impaired discrimination during retrieval when contexts were similar (mean = 0.09, SEM = 0.098, P < 0.01; Fig. 3C,Supplementary Figure S7). This effect was specific, as it was not detected for the dissimilar context (Fig. 3E,Supplementary Figure S7), suggesting that DG SOM regulate the encoding of object-in-context memory only when contextual information is similar. Overall, this result suggests that SOM regulate the encoding of contextual recognition memory by controlling excitatory activity in the DG.
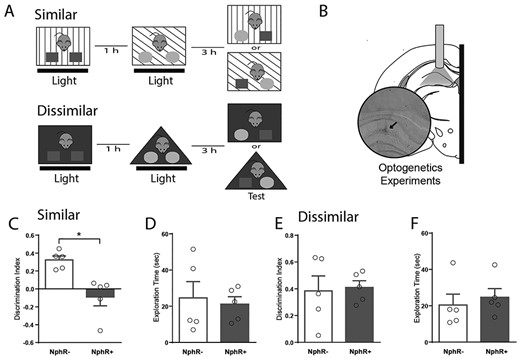
Encoding of overlapping contextual memories requires somatostatin cells in the DG. (A) Schematic representation of the two versions of the object-in-context behavioral test used to assess context discrimination. (B) Anatomical representation of the hippocampus locations targeted during optogenetic experiments. Black arrow indicates the location of fibber tip. Histological verification was performed with cresyl violet staining. Optogenetic experiments were performed in either transgenic mice expressing functional halorhodopsin (NpHR+) or control mice lacking functional halorhodopsin (NpHR-) optically stimulated (5 s-pulse every 15 s, 15 mW) during both training sessions. DI accumulated over the entire behavioral test (5 min) for animals during optogenetic probing for both versions of the behavioral test, similar (C) and dissimilar (E). Note a significant effect only in the similar condition. Total exploration time accumulated over the entire behavioral test (5 min) for both versions of the behavioral test was not affected (D, F). Data are represented as population mean ± SEM and individual animals (circles). Unpaired Student’s t-test; 2C, *P = 0.005; 2D, P = 0.75; 2E, P = 0.84; 2F, P = 0.63
Discrimination of Overlapping Spatial Configurations is Regulated by Somatostatin Neurons During Memory Encoding
We showed that SOM can control the encoding of contextual recognition memory. Previous studies have shown the essential role played by granule cells in encoding distinct neural representations of overlapping spatial configurations (Leutgeb et al. 2007; Deng et al. 2013b; Neunuebel and Knierim 2013). Furthermore, inhibition of the lateral entorhinal input disrupts pattern separation in spatial tasks (Vivar et al. 2012). Therefore, we reasoned that hilar SOM may also regulate the encoding of overlapping spatial configurations. To test this, we conducted the spontaneous location recognition task that has been shown to be sensitive to the functional integrity of the DG (Bekinschtein et al. 2013). In this task animals explore three identical objects placed in separate locations and are then tested with two objects, with one object placed in a familiar location and another object placed in between the previous two locations (Fig. 4A). As the two objects are placed closer together in the training session, it becomes more difficult for mice to discriminate the novel location in the test session (Bekinschtein et al. 2013; Miranda et al. 2017, 2018). Accordingly, we varied the angle between objects during the training sessions in small (50°), medium (120°), or large (180°) separations; and compared the exploration times between the training and test session. We found that mice were able to discriminate correctly the medium and large separations, yet failed to distinguish the small separation (mean = −0.062, SEM = 0.068, P < 0.05; Fig. 4B). This effect was robustly expressed during the entire test session (Supplementary Figure S8). These results suggest that the small separation is too ambiguous for mice to be able to discriminate it. Thus, we assessed whether spatial discrimination of the medium configuration relied on the activity of hilar SOM. For that, we chronically implanted bilateral optic fibers on the hippocampal fissure of transgenic NpHR+ mice (Fig. 4D) to optogenetically inhibit SOM during memory encoding. Laser stimulation had no effect on exploratory behavior during the training sessions (Supplementary Figure S9). However, optogenetic inhibition of SOM during acquisition selectively impaired spatial discrimination in NpHR+ mice without affecting performance in control NpHR- mice (mean = 0.014, SEM = 0.012, P < 0.05; Fig. 4E). Importantly, exploratory behavior of mice was not affected by laser stimulation (Fig. 4F). This result suggests that SOM regulate the encoding of spatial recognition memory by controlling neural activity in the DG. Another possible interpretation of our results could be related with changes in the number of objects used in the task. Nonetheless, the original study that designed and described the spatial task demonstrated that discrimination depended on the position of the objects, not on their number (Bekinschtein et al. 2013). Overall, our results suggest that SOM can regulate both the reactivity and excitability of granule cells in-vivo (Supplementary Figure S10).
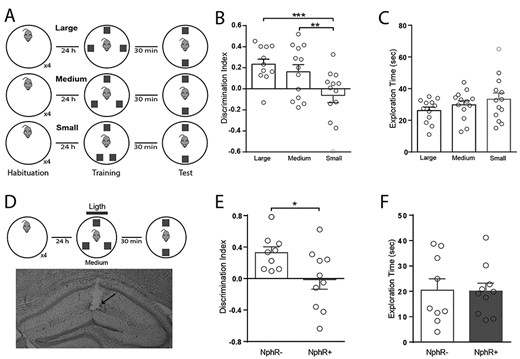
DG somatostatin cells regulate the encoding of spatial recognition memory. (A) Schematic representation of the spontaneous location recognition test used in three versions (large = 180°, medium = 120°, and small = 50° separation) to assess spatial memory. Note that angular distance between B and C objects change in training. (B) DI accumulated over the entire behavioral test (5 min) for animals performing the three different versions. Note that only the small separation is not discriminated. One-way ANOVA, P = 0.0057; Tukey post-hoc test; **P = 0.039; ***P = 0.006. (C) Total exploration time of test objects accumulated over the entire session for every version of the test, One-way ANOVA, P = 0.26. (D) Schematic representation of the medium version of the spontaneous location recognition test combined with optogenetic stimulations (blue line; 5 s-pulse every 15 s, 15 mW) delivered during training. Bottom, coronal Nissl-stained coronal hippocampus section showing the track of the chronically implanted optic fiber (arrow). (E) DI accumulated over the entire behavioral test for animals performing the intermediate version of the spontaneous location recognition test combined with optogenetic stimulation. Unpaired Student’s t-test; *P = 0.03. (F) Total exploration time of test objects accumulated over the complete session. Unpaired Student’s t-test; P = 0.94.
Discussion
Here, we studied the physiological and behavioral effects of selectively suppressing DG SOM during contextual memory acquisition. We found that inhibition of SOM increased the activity of two neuronal populations identified on the basis of their brief spike waveforms and firing patterns. Indeed, inhibitory interneurons, putative PV cells, were identified by their fast spiking pattern and short spikes (Lübke et al. 1998; Hosp et al. 2014; Senzai and Buzsáki 2017) whereas, excitatory neurons, putative granule cells, and mossy cells were recognized by their bursting pattern and slow spikes (Senzai and Buzsáki 2017). Both neuronal populations increased their activity during laser stimulation, probably resulting from synaptic disinhibition (Yuan et al. 2017), which was also consistent with the enhanced incidence of dentate spikes, a hallmark of DG synchronous activity (Bragin et al. 1995; Penttonen et al. 1997). Low excitability of granule cells has been proposed as a requirement for efficient orthogonalization of afferent spiking patterns arising in the entorhinal cortex (Rolls 2013). Thus, nonselective increments of activity in granule cells are predicted to alter efficient pattern separation. By performing two types of episodic-like memory tasks, one contextual and another spatial, we reveal that discrimination of similar memories is disrupted by optogenetic inhibition of DG SOM.
The hippocampus has been proposed as a key structure for the control of memory expression using contextual information (Preston and Eichenbaum 2013). For this reason, the ability to differentiate overlapping contextual information during encoding should be important to facilitate posterior memory recall (Greene et al. 2013). In this regard, previous studies have typically used the fear conditioning paradigm to characterize the role of the DG in encoding overlapping contextual information (McHugh et al. 2007; Sahay et al. 2011). Given the emotional valence of this kind of task, the amygdala is likely to be recruited (Zheng et al. 2019), and possibly activate the hippocampus. Indeed, the basolateral amygdala is densely interconnected with the ventral hippocampus (Felix-Ortiz and Tye 2014; Yang and Wang 2017; Ahlgrim and Manns 2019). Thus, engagement of the DG is expected when the amygdala is massively activated during aversive memory formation. The DG also participates in the differentiation of overlapping object-spatial representations in neutral conditions (Bekinschtein et al. 2013; Miranda et al. 2018). By experimentally manipulating object-context associations, we establish here that excitatory activity in the DG mediated by AMPA receptors is necessary to discriminate overlapping contextual associations. Our results suggest that the DG is involved in the general process of discriminating overlapping spatial-contextual information independently of its emotional valence. Specifically, we show that inhibition of hilar SOM impaired the acquisition of similar contextual and spatial representations, thus suggesting that somatostatin-dependent inhibition of the DG is at play in the discrimination of different types of similar episodic memories. Moreover, it is also remarkable the animal performance variability between spatial and contextual tests. This effect could be related with the differences in the size and shape of the arenas used for each protocol, as well as the optogenetic stimulation protocol. Indeed, since the arena used for the spatial task was larger than those used in the contextual task, the probability of inhibiting SOM precisely during object exploration may be much lower. Future experiments, using closed-loop optogenetic stimulation may well solved this issue.
During pattern separation, different neuronal engrams represent similar contexts (Deng et al. 2013b). It has been shown that some of the DG cells are highly sensitive to small changes in contextual cues (Leutgeb et al. 2007; Danielson et al. 2016b), suggesting that DG ensembles recognize differences in contextual information with high sensitivity. Importantly, SOM regulate the size of such memory ensembles (Stefanelli et al. 2016), and thus inhibiting SOM is expected to dysregulate both the size and specificity of memory engrams. This hypothesis is supported by the anatomical distribution of SOM that selectively target the most external region of the dendritic trees of granule cells in the outer molecular layer (Freund and Buzsáki 1996), where contextual information is conveyed by entorhinal inputs (Hargreaves et al. 2005), and damage to those afferents impairs spatial pattern separation (Vivar et al. 2012). Thus, the behavioral impairment resulting from the optogenetic inhibition of SOM could be attributed to deficits in the ability of the DG to differentially encode contextual information resulting from impaired pattern separation.
Pattern separation is believed to be critical during contextual memory acquisition, but its contribution to memory recall remains debated. It has been proposed that inhibition and excitation of DG circuits play different roles during encoding, consolidation, or recall of overlapping memories (Lee and Kesner 2004; Rolls 2018). This is supported by the blockade of DG AMPA and kainite receptors, which impairs the expression of fear memory (Pierson et al. 2015), while the blockade of GABAA receptors impairs consolidation, yet not the acquisition or retrieval of fear memories (Shahidi et al. 2008). Some theoretical models propose that sparse patterns of neuronal activation in the DG guide memory encoding in the CA3 region, whereas memory retrieval is mediated through direct entorhinal inputs to the CA3 region (Rolls and Kesner 2006). However, other studies suggest that the DG contributes to both memory encoding and retrieval (Denny et al. 2014; Krasne et al. 2015; Bernier et al. 2017). This is supported by recent studies showing that recall cues can trigger reactivation of neural ensembles active in the DG during memory encoding (Liu et al. 2012; Ramirez et al. 2013; Ryan et al. 2015). Interestingly, we found that blockade of DG AMPA receptors did not affect the recall of contextual memories. The differences observed could be, at least partly, due to methodological reasons. For example, we pharmacologically blocked AMPA receptors before the test session whereas the above-mentioned studies selectively controlled very specific subsets of DG neurons. Hence, our results support the idea that the role of the DG in the process of pattern separation is restricted to the encoding phase, at least, for emotionally-neutral memories.
Feedback inhibition in the DG is necessary for appropriate orthogonalization of neuronal ensembles representing similar memory episodes (Mcavoy et al. 2015). SOM receive direct inputs from both mature (Freund and Buzsáki 1996) and newborn (Groisman et al. 2020) granule cells. Both granule cell populations have been proposed as drivers of feedback inhibition in pattern separation (Drew et al. 2016; Stefanelli et al. 2016). Several studies proposed a leading role for newborn granule cells (Mcavoy et al. 2015; Drew et al. 2016). Indeed, the activation of newborn granule cells engages inhibitory feedback principally arising from PV interneurons that provide perisomatic inhibition, but not from SOM that provide dendritic inhibition (Temprana et al. 2015). Moreover, ablation of hippocampal neurogenesis induces high excitability in granule cells (Ikrar et al. 2013) and impairs pattern separation (Clelland et al. 2009), supporting the idea that feedback inhibition mediated by PV interneurons controls pattern separation. On the other hand, a recent study suggests that mature granule cells poorly engage PV interneurons and preferentially excite SOM in vivo (Stefanelli et al. 2016). Thus, it is plausible that in pattern separation two parallel circuits are engaged during feedback inhibition. One circuit in which newborn granule cells preferentially recruit PV cells and another circuit where mature granule cells preferentially activate SOM that would control the inhibition of granule cells directly. Since newborn cells are more excitable than granule cells (Marín-burgin et al. 2012), it is possible that feedback activity of SOM is more delayed than feedback inhibition of PV cells. Interestingly, computational models of pattern separation predict little contribution for the inhibitory feedback provided by SOM (Mcavoy et al. 2015). Our results suggest that feedback inhibition provided by SOM is necessary for pattern separation and it will be interesting that future computational studies consider this parameter when modeling hippocampal networks. Further, we found that prolonged optogenetic suppression of SOM triggered a brief rebound activation at the end of the pulse that was associated with brief increased excitability of the DG network. Since we did not test shorter pulses, we could not study the effect of different durations on the amplitude and dynamics of the rebound response. This is relevant to assess the occurrence of such rebounds under physiological conditions, which future experiments will have to address.
Furthermore, the regulation of orthogonalization of granule cells relies heavily on the perisomatic lateral inhibition provided by PV cells (Sambandan et al. 2010; Guzman et al. 2019). In turn, lateral inhibition might be controlled by SOM through direct dendritic inhibition of granule cells and perisomatic inhibition of PV cells (Yuan et al. 2017). When mice explore novel environments, thus forming new memories, or during intense presynaptic activity in the DG in vitro, dendritic inhibition is significantly larger than perisomatic inhibition (Moser 1996; Liu et al. 2014). A population of SOM locally innervates fast-spiking PV cells in the DG and distally several other cell-types in the septum (Yuan et al. 2017). Consistent with such anatomic connectivity, we found that inhibition of SOM increased the activity of putative PV neurons and granule cells. Importantly, SOM do not constitute a homogeneous population in the DG as not all cells project to the outer blade of the molecular layer, and some of them project long-range axons reaching the medial septum (Yuan et al. 2017). Our experimental design was not able to discriminate between these neuronal populations, thus it is possible that some of the effects here described were, at least partially, mediated by those long-range projections reaching the medial septum. Future experiments will have to address this possibility to establish the exact contribution of different subpopulations of SOM. Moreover, we observed increased incidence of dentate spikes, which results from simultaneous, brief dendritic depolarization and perisomatic inhibition of granule cells (Bragin et al. 1995; Penttonen et al. 1997). Furthermore, SOM synaptically target PV interneurons unidirectionally, with no feedback projection described to date (Acsády et al. 2000; Savanthrapadian et al. 2014b). This synaptic projection regulates both the discharge probability and spike timing of PV neurons (Savanthrapadian et al. 2014b; Yuan et al. 2017). These changes in the DG circuit are consistent with memory deficits and disrupted pattern separation taking place in cases where the population of hilar SOM is selectively decreased, such as epilepsy or aging (Holden and Gilbert 2012; Spiegel et al. 2013; Reyes et al. 2018). In summary, our results suggest that SOM regulate general excitability in the DG and are required for pattern separation during episodic memory encoding.
Notes
We thank Maria José Díaz and David Alberto Jaime for their technical assistance. This work was supported by the National Commission for Scientific and Technological Research (CONICYT) scholarship, Chile (to C.M. 21140967); The School of Medicine, Pontificia Universidad Catolica de Chile (to C.M. PMD-02/17), The Programa de Investigacion Asociativa (PIA) Anillos de Ciencia y Tecnologia (to P.F. ACT 172121); and Fondecyt Regular grant (to P.F. 1190375); The National Agency of Scientific and Technological Promotion of Argentina (ANPCyT, to N.V.W. PICT 2015-2344), and 2016 LARC-IBRO PROLAB program (to N.V.W.) for Latin American labs co-operation.
References
Arias-Gil G, Ohl FW, Takagaki K, Lippert MT.
Peixoto HM, Cruz RMS, Moulin TC, and Leão RN.
Picot A, Dominguez S, Liu C, Chen IW, Tanese D, Ronzitti E, et al.
Zheng J, Stevenson RF, Mander BA, Mnatsakanyan L, Hsu FPK, Vadera S, et al.
Author notes
Cristian Morales and Juan Facundo Morici contributed equally to this work.