-
PDF
- Split View
-
Views
-
Cite
Cite
Anniek Frederike Lubberding, Simon Veedfald, Jonathan Samuel Achter, Sarah Dalgas Nissen, Luca Soattin, Andrea Sorrentino, Estefania Torres Vega, Benedikt Linz, Caroline Harriet Eggert Eggertsen, John Mulvey, Signe Toräng, Sara Agnete Larsen, Anne Nissen, Lonnie Grove Petersen, Secil Erbil Bilir, Bo Hjorth Bentzen, Mette Marie Rosenkilde, Bolette Hartmann, Thomas Nikolaj Bang Lilleør, Saddiq Qazi, Christian Holdflod Møller, Jacob Tfelt-Hansen, Stefan Michael Sattler, Thomas Jespersen, Jens Juul Holst, Alicia Lundby, Glucagon-like peptide-1 increases heart rate by a direct action on the sinus node, Cardiovascular Research, Volume 120, Issue 12, August 2024, Pages 1427–1441, https://doi.org/10.1093/cvr/cvae120
- Share Icon Share
Abstract
Glucagon-like peptide-1 receptor agonists (GLP-1 RAs) are increasingly used to treat type 2 diabetes and obesity. Albeit cardiovascular outcomes generally improve, treatment with GLP-1 RAs is associated with increased heart rate, the mechanism of which is unclear.
We employed a large animal model, the female landrace pig, and used multiple in vivo and ex vivo approaches including pharmacological challenges, electrophysiology, and high-resolution mass spectrometry to explore how GLP-1 elicits an increase in heart rate. In anaesthetized pigs, neither cervical vagotomy, adrenergic blockers (alpha, beta, or combined alpha-beta blockade), ganglionic blockade (hexamethonium), nor inhibition of hyperpolarization-activated cyclic nucleotide–gated (HCN) channels (ivabradine) abolished the marked chronotropic effect of GLP-1. GLP-1 administration to isolated perfused pig hearts also increased heart rate, which was abolished by GLP-1 receptor blockade. Electrophysiological characterization of GLP-1 effects in vivo and in isolated perfused hearts localized electrical modulation to the atria and conduction system. In isolated sinus nodes, GLP-1 administration shortened the action potential cycle length of pacemaker cells and shifted the site of earliest activation. The effect was independent of HCN blockade. Collectively, these data support a direct effect of GLP-1 on GLP-1 receptors within the heart. Consistently, single nucleus RNA sequencing showed GLP-1 receptor expression in porcine pacemaker cells. Quantitative phosphoproteomics analyses of sinus node samples revealed that GLP-1 administration leads to phosphorylation changes of calcium cycling proteins of the sarcoplasmic reticulum, known to regulate heart rate.
GLP-1 has direct chronotropic effects on the heart mediated by GLP-1 receptors in pacemaker cells of the sinus node, inducing changes in action potential morphology and the leading pacemaker site through a calcium signalling response characterized by PKA-dependent phosphorylation of Ca2+ cycling proteins involved in pacemaking. Targeting the pacemaker calcium clock may be a strategy to lower heart rate in people treated with GLP-1 RAs.
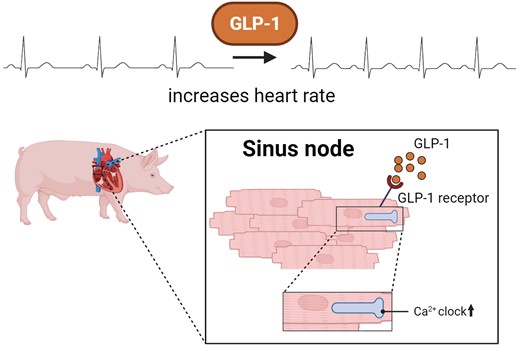
Time of primary review: 24 days
See the editorial comment for this article ‘New insights into the effects of glucagon-like peptide-1 on heart rate and sinoatrial node function’, by S. Kaur and R.A. Rose, https://doi.org/10.1093/cvr/cvae150.
1. Introduction
Glucagon-like peptide-1 receptor agonists (GLP-1 RAs) are attractive compounds for the treatment of type 2 diabetes (T2D) and chronic weight management.1 Patients with T2D are at increased risk of cardiovascular disease, a primary cause of morbidity and mortality in this population. Clinical data from randomized cardiovascular outcome trials have demonstrated that GLP-1 RAs significantly reduce major adverse cardiovascular events, as well as cardiovascular morbidity and mortality in T2D patients.2–9 A meta-analysis demonstrated 14% reduction in major cardiovascular events and 12% reduction in all-cause mortality.1 Similar findings have recently been reported for people with obesity without T2D, but with pre-existing cardiovascular disease.10 Despite the reported cardiovascular benefits, the prescription of GLP-1 RA by cardiologists has so far remained low.11 There are several possible reasons for this, which could span from clinical inertia, a sense that prescribing these drugs require endocrinologist expertise, or to the subcutaneous mode of administration of most drugs in this class.12 Another possibility may be uncertainties related to GLP-1 RA administration increasing heart rate and the latter having been associated with cardiovascular mortality.13 A resting heart rate increase of 5 b.p.m. has been associated with a 17% increase in cardiovascular mortality,14 with estimates exceeding 20% for heart rate increases of 10 b.p.m.13 GLP-1 RA cardiovascular outcome trials2–9 and head-to-head studies,15–29 with a follow-up range of 0.5–5.4 years, have documented that GLP-1 RAs increase heart rate by 0.1–5.7 b.p.m., with an average increase of 2.4 b.p.m. During continuous monitoring of GLP-1 RA effects on heart rate, increases as high as 6–10 b.p.m. have been reported.30 Despite the well-established clinical cardiovascular benefits of GLP-1 RAs, the chronotropic effect may limit the use of GLP-1RAs in some patients with underlying heart conditions. For instance in patients with heart failure with reduced ejection fraction, where heart rate is a risk factor,31 its reduction improves clinical outcome32,33 and guidelines accordingly recommend rate control with beta-blockers or ivabradine when necessary.34
The chronotropic mechanism of GLP-1 RAs remains unclear, although involvement of the autonomic nervous system has been proposed.35–37 Evidence from rodents suggested that sympathetic input is required for the response.35,38,39 However, there are important species differences.40 Plasma concentrations of adrenaline and noradrenaline are not increased in humans after i.v. infusion of GLP-1 in doses sufficient to elevate heart rate,41 and GLP-1 receptor expression was reported in the human sinus node.42–44 Recently, a single cell gene expression-based informatics approach identified pacemaker cells as cellular targets for GLP-1 RAs,44 suggesting that at least part of the positive chronotropic effect may be mediated through the sinus node. Yet, the relation between autonomic nervous system activation, GLP-1 receptor expression in the heart, and the chronotropic action of GLP-1 RAs is unclear. Here, we use a large mammalian animal model with cardiac electrophysiological properties close to that of humans45 to test the hypothesis that (i) the chronotropic effect of GLP-1 is independent of the autonomic nervous system, (ii) the chronotropic effect of GLP-1 is a direct effect on the heart through the GLP-1 receptor, (iii) GLP-1 affects electrophysiological properties of the sinus node, (iv) the chronotropic effect is mediated by changes of the phosphorylation state of key proteins that control Ca2+ homeostasis in myocytes, and (v) the signalling effect is elicited in pacemaker myocytes in the sinus node. Our results unveil a molecular mechanism for the chronotropic effect of GLP-1 and document that the response is independent of autonomic innervation and hyperpolarization-activated cyclic nucleotide–gated (HCN) currents, but depends on the GLP-1 receptor. The response is mediated by a shortened cycle length and steeper diastolic depolarization potential of pacemaker action potentials and a shift of the site of earliest activation within the sinus node. Our findings highlight calcium clock signalling of the cardiac pacemaker as a potential target to prevent the increase in heart rate in patients receiving GLP-1 RAs.
2. Methods
Experiments were performed in female Danish landrace pigs, acclimatized for 1 week in the animal house at the Department of Experimental Medicine, Faculty of Health and Medical Sciences, University of Copenhagen. Pigs were kept at a 12/12 h light–dark cycle separated by a 15–30 min dusk period. Procedures conformed with Danish legislation governing animal experimentation, with the principles stated in the ‘Guide for the Care and Use of Laboratory Animals’ published by the National Institute of Health guidelines (National Institutes of Health publication No. 85–23, Revised 1996), and with permission granted from the Animal Experiments Inspectorate, Ministry of Justice, Denmark (licence number 2018-15-0201-01397) and approval by the local institutional animal care and use committee. All pigs were pre-medicated with intramuscular injections of midazolam (Hameln, 1 mg/kg) and ketamine (ketaminol Vet, 10 mg/kg). For anaesthesia, a propofol infusion of 15–17 mg/kg/h was used during surgical preparation, which was reduced to 12.5 mg/kg/h during the equilibration period and experiments. A constant infusion of fentanyl was used to ensure analgesia (5 µg/kg/h). At the end of in vivo experiments, pigs were euthanized under anaesthesia by an intravenous bolus of 100 mg/kg pentobarbital (Euthanimal, Alfasan, Netherlands). For isolation of hearts and sinus nodes, pigs were euthanized under deep euthanasia by exsanguination upon excision of the heart. Synthetic GLP-1-(7–36) amide was purchased from Polypeptide Laboratories. GLP-1 infusions were administered intravenously using a precision pump at a rate of 10–15 pmol/kg/min for 45–60 min periods depending on the protocol. Details are given in the Supplementary material.
For in vivo experiments, the alpha-adrenergic blocker phentolamine, the beta-adrenergic blocker propranolol, the HCN channel blocker ivabradine, and the ganglion blocker hexamethonium were purchased from Sigma-Aldrich. The dipeptidyl peptidase 4 (DPP-4) inhibitor valine pyrrolidide (Val-Pyr) was a kind gift from Novo Nordisk A/S. The muscarinic blocker Atropine was purchased from the Capital Region Pharmacy (Region Hovedstadens Apotek, Herlev, Copenhagen). All compounds were dissolved in isotonic saline and administered via a central vein catheter. Details of preparation and administration methods are described in the Supplementary material.
In isolated heart perfusion experiments, pig hearts were placed in an Organ Care System (TransMedics, Massachusetts USA) and perfused through the aorta. Hearts stabilized for 45 min followed by aortic bolus injections of GLP-1 or exendin-9-39. Pseudo electrocardiogram (ECG), atrial effective refractory period, and conduction velocity were measured. Details are provided in the Supplementary material.
In isolated sinus node superfusion experiments, sinus nodes were isolated and superfused. Either a monophasic action potential electrode was placed in the sinus node region for sinus node action potentials, or a flexible 64 high-density unipolar multi-electrode array (Mapping Lab, UK) was placed over the sinus node area. Sinus nodes were allowed to stabilize for 30 min followed by continuous superfusion with GLP-1 (1 nM), ivabradine (1 µM), or GLP-1 + ivabradine. Cyclic adenosine monophosphate (cAMP) measurements were performed from supernatants. Details are provided in the Supplementary material.
Procedures for sinus node and myocardial tissue isolation for transcriptomic and proteomic analysis were performed on hearts excised, while the pericardium was opened during ongoing infusion of GLP-1 or saline. Sinus node tissue homogenization, peptide preparation, tandem mass tag (TMT) labelling, phosphopeptide enrichment, off-line peptide fractionation, and mass spectrometry measurements followed published procedures46 and are detailed in the Supplementary material. Sinus node preparations for single nucleus RNA sequencing (snRNAseq) are also detailed in the Supplementary material.
2.1 Statistical analysis
Data are presented as mean data with standard error of the mean (SEM). Information on statistical testing is provided in figure legends. Graphs were prepared using GraphPad Prism (GraphPad Software, La Jolla, California, USA).
3. Results
3.1 Administration of GLP-1 increases heart rate
The effect of GLP-1 on heart rate was evaluated in vivo in anaesthetized pigs by two sequential 45 min infusions of GLP-1 followed by 45 min washout periods. Heart rate increased consistently during both GLP-1 infusions with an average increase of 25 ± 4 and 21 ± 3 b.p.m. (Figure 1A–C). We assessed whether a similar effect could be elicited by the primary metabolite of GLP-1 after DPP-4 cleavage, GLP-1 9-36 amide, as reported in mice.47 In addition, the effect was evaluated in the absence or presence of the DDP-4 inhibitor Val-Pyr. Infusions of the intact hormone, but not the metabolite, increased heart rate, regardless of the order of administration of GLP-1 and its metabolite (Figure 1D–F). Concomitantly to increasing heart rate, GLP-1 administration increased cardiac output and diastolic blood pressure but decreased stroke volume and systemic peripheral resistance (see Supplementary material online, Figure S1). There was no evidence that the increase in heart rate was a baroreceptor-mediated, secondary response to haemodynamic changes since blood pressure did not drop (see Supplementary material online, Figure S1); rather, the change in heart rate appears as a primary effect.
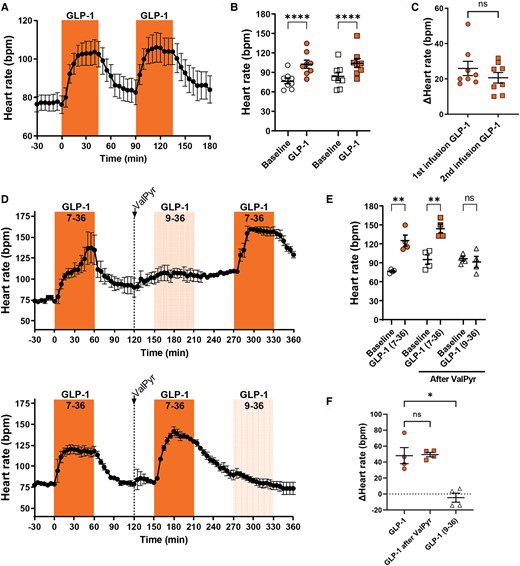
GLP-1 increases heart rate in the anaesthetized pig, but its metabolite GLP-1 (9-36) does not. (A–C) GLP-1 was infused at 10 pmol/kg/min for two consecutive 45 min periods with two 45 min washout periods (n = 8). Heart rate increased similarly in both infusion periods and was followed by a return to near-baseline during washout. (D–F) GLP-1 was infused at 15 pmol/kg/min for 1 h as a control, followed by a bolus injection of the DPP-4 inhibitor Val-Pyr (300 µmol/kg). Subsequently, an hour infusion of GLP-1 at 15 pmol/kg/min either preceded or followed by an hour infusion of the metabolite GLP-1 (9-36) at 10 pmol/kg/min was performed (n = 4). GLP-1 increased heart rate consistently, whereas its metabolite GLP-1 (9-36) did not. Tested with two-way repeated measures ANOVA with Bonferroni multiple comparisons testing (B and E), paired t-test (C), and repeated measures one-way ANOVA (F); *P < 0.05, **P < 0.01, ****P < 0.0001.
3.2 The chronotropic effect of GLP-1 is independent of beta- and/or alpha-adrenergic blockades
To test if the sympathetic nervous system is involved in the GLP-1-induced heart rate increase, we repeated the GLP-1 infusion experiments in the presence of relevant doses of pharmacological blockers of the beta- or alpha-adrenergic receptors after a first (control) GLP-1 dose (Figure 2). Atropine was administered at the end of the experiments as a positive control. Heart rate invariably increased during the first (control) GLP-1 infusion. Neither administration of saline, beta-adrenergic blockade, alpha-adrenergic blockade, nor combined beta- and alpha-adrenergic blockade affected heart rate excursions at the second GLP-1 infusion (Figure 2A–G and Supplementary material online, Figure S3A), giving comparable increases in heart rate to the first (control) GLP-1 infusion (Figure 2B, D, F, and G). Blood pressures were unaffected by receptor blockades (see Supplementary material online, Figure S2), and the final atropine infusion always increased heart rate. Hence, we found no evidence for sympathetic nervous system involvement in the chronotropic effect of GLP-1.
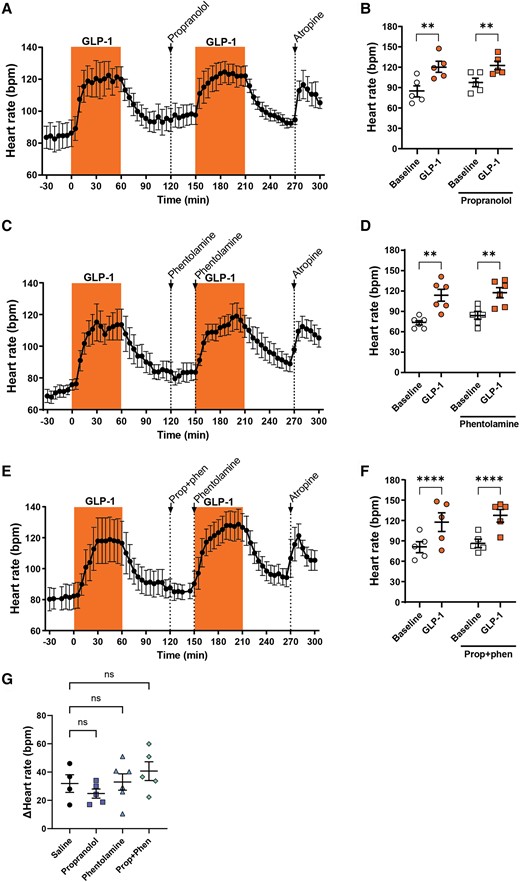
The increase in heart rate induced by GLP-1 is independent of alpha- and beta-adrenergic stimulation. GLP-1 was infused at 15 pmol/kg/min for two 60 min periods. Before the second infusion period, bolus injections of either propranolol (1 mg/kg; A and B), phentolamine (1 + 0.5 mg/kg; C and D), or a combination of propranolol and phentolamine (E and F) were given to block the beta-adrenergic receptors (n = 5), alpha-adrenergic receptors (n = 6), or both (n = 5), respectively. Heart rate increased with subsequent GLP-1 infusion regardless of the pre-treatment with any of the adrenergic blockers. The GLP-1-induced increase in heart rate after the interventions was comparable between interventions (G). Tested with two-way repeated measures ANOVA with Bonferroni multiple comparisons testing (B, D, and F) and one-way ANOVA with Dunnett’s multiple comparisons testing (G); **P < 0.01, ****P < 0.0001.
3.3 GLP-1 increases heart rate despite cervical vagotomy, autonomic ganglion blockade, and HCN channel blockade
Next, we evaluated if the parasympathetic nervous system, or the combined autonomic nervous system, is involved in the GLP-1-induced increase in heart rate. In the same set of experiments, we also evaluated the involvement of the sinus nodal HCN channel. Accordingly, anaesthetized pigs were exposed to acute cervical vagotomy with subsequent blocking of HCN channels with ivabradine or autonomic ganglion block (Figure 3; Supplementary material online, Figure S3). All interventions were preceded by an initial control infusion of GLP-1, which increased heart rate in all experiments (Figure 3). Cervical vagotomy resulted in an immediate increase in heart rate (Figure 3A) and blood pressure (see Supplementary material online, Figure S3A). A new baseline was reached prior to the second GLP-1 infusion. GLP-1 infusion after vagotomy increased the heart rate in all pigs by 18 ± 4 b.p.m., comparable with the heart rate increase observed during the initial control infusion with GLP-1 of 20 ± 1 b.p.m. (Figure 3A–C). Subsequent bolus injection of ivabradine rapidly reduced heart rate to a level similar to that observed before vagotomy. However, GLP-1 still elicited an increase in heart rate comparable with the previous infusions (16 ± 3 b.p.m.; Figure 3A–C). In another set of experiments, we evaluated the effect of ganglion blockade. As in previous experiments, the initial control infusion of GLP-1 robustly increased heart rate (Figure 3D). Subsequent hexamethonium administration immediately led to a robust and stable increase in heart rate (Figure 3D) and a modest but brief increase in blood pressure (see Supplementary material online, Figure S3B). Administration of a second bolus of hexamethonium did not lead to further changes in either heart rate or blood pressure. Indeed, within minutes after the first bolus of hexamethonium, a plateau in heart rate had been reached. Subsequent infusion of GLP-1 further increased heart rate by 30 ± 7 b.p.m., which was comparable with the first control GLP-1 infusion of 33 ± 4 b.p.m. (Figure 3D–F). After the final infusion of GLP-1, atropine was administered, which affected neither heart rate nor blood pressure, confirming the lack of parasympathetic nervous system signalling (Figure 3D; Supplementary material online, Figure S3B). Thus, neither the parasympathetic nervous system, the ganglia, nor the HCN channels are involved in the chronotropic effect of GLP-1.

The increase in heart rate induced by GLP-1 is independent of the autonomic nervous system or the HCN channel. (A–C) GLP-1 was infused at 10 pmol/kg/min for 45 min as a control. Subsequently cervical vagotomy was performed, followed by a second infusion of GLP-1. This was followed by a bolus injection of the HCN channel blocker ivabradine (0.35 mg/kg) and a third infusion of GLP-1 (n = 6). All infusions of GLP-1 increased heart rate. (D–F) GLP-1 was infused at 12.5 pmol/kg/min for 45 min as a control, followed by two bolus injections of the ganglionic blocker hexamethonium (8 mg/kg, administered twice), and another 45 min infusion of GLP-1 (n = 5). Even after ganglionic block, heart rate increased during the GLP-1 infusion. Tested with two-way repeated measures ANOVA with Bonferroni multiple comparisons testing (B and E), repeated measures one-way ANOVA with Dunnett’s multiple comparisons testing (C), and paired t-test (F); *P < 0.05, ****P < 0.0001.
3.4 The chronotropic effect of GLP-1 is present in isolated hearts and is mediated by the GLP-1 receptor
The in vivo pharmacological experiments cannot exclude an influence of circulating factors. Therefore, we used an isolated perfused heart set-up to evaluate the effect of GLP-1 on the porcine heart in an isolated system. In the isolated perfused heart preparation, bolus injections of GLP-1 increased heart rate, with an average increase of 12 ± 2 b.p.m. (Figure 4A and B). After GLP-1 injection, heart rate returned to near-baseline levels within ∼30 min (Figure 4A). After two boluses of GLP-1, a bolus of the selective GLP-1 receptor blocker exendin-9-39 was given. This decreased heart rate to a new baseline. A subsequent injection of GLP-1 no longer affected heart rate (Figure 4A and B). Two hearts that were in a sustained monomorphic accelerated ideoventricular rhythm were also exposed to the two doses of GLP-1 and revealed no effect (see Supplementary material online, Figure S4). Collectively the ex vivo experiments support a direct effect of GLP-1 on the heart’s sinus node, which is mediated through the GLP-1 receptor.
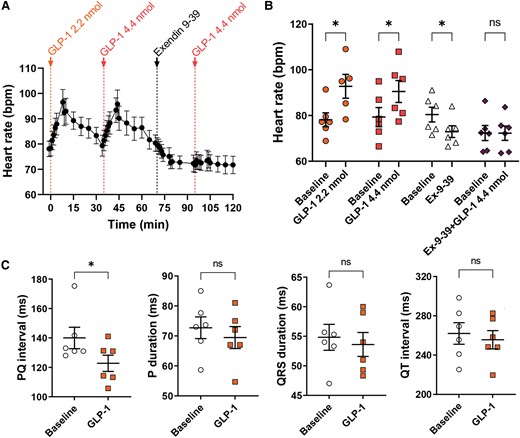
GLP-1 increases heart rate in the isolated perfused pig heart, which is dependent on the GLP-1 receptor, and increases AV nodal conduction. (A and B) Isolated perfused hearts were exposed to consecutive boluses of GLP-1 (2.2 nmol), GLP-1 (4.4 nmol), the GLP-1 receptor blocker exendin 9-39 (100 nmol), and GLP-1 (4.4 nmol) (n = 6). Heart rate increased with both doses of GLP-1, but GLP-1 no longer affected heart rate after the bolus of the GLP-1 receptor blocker. (C) To evaluate other electrophysiological effects of GLP-1, ECG was measured in vivo in the anaesthetized pig during atrial pacing (400 ms basic cycle length) before and after 30 min infusion of GLP-1 (10 pmol/kg/min) (n = 6). PQ interval shortened, but the other parameters were not affected, indicating increased AV nodal conduction. Tested with two-way repeated measures ANOVA with Bonferroni multiple comparisons testing (B) and with paired t-test (C); *P < 0.05.
3.5 GLP-1 modulates conduction from the atria to the ventricles
Given the direct cardiac effect of GLP-1, it became pertinent to evaluate cardiac electrophysiological effects. Accordingly, electrophysiological studies were performed in vivo in the anaesthetized pig and ex vivo in the isolated perfused pig heart. ECG analysis in vivo during atrial pacing (cycle length 400 ms) revealed a shortening of the PQ interval but no effect on other parameters (Figure 4C). The shortened PQ interval reflects faster atrioventricular conduction. Atrial conduction velocity, measured by catheters placed either in the high right atrium or coronary sinus, was unaffected by GLP-1 administration (see Supplementary material online, Figure S5A). In vivo, atrial effective refractory period (ERP) was reduced by GLP-1 (see Supplementary material online, Figure S5B), which was confirmed in the isolated perfused pig heart, and found to be dependent on the GLP-1 receptor (see Supplementary material online, Figure S5C). Ventricular ERP and atrioventricular (AV) nodal refractoriness were unaffected (see Supplementary material online, Figure S5D). These data suggest that cardiac GLP-1 exposure not only increases heart rate but also decreases conduction time from the atria to the ventricles.
3.6 In isolated sinus nodes, GLP-1 shortens action potential cycle length and shifts the site of earliest activation independently of HCN channel conduction
To further investigate the electrophysiological effect of GLP-1 on the sinus node, isolated porcine sinus nodes (see Supplementary material online, Figure S6A) were exposed to continuous superfusion with GLP-1, ivabradine, or ivabradine + GLP-1. GLP-1 shortened the cycle length of pacemaker action potentials (Figure 5A–C). The effect of GLP-1 corresponds to an average increase in rate of 33 ± 6%. Monophasic action potential recordings of the sinus node also demonstrated a significantly steeper diastolic depolarization potential upon GLP-1 administration (Figure 5C and D and Supplementary material online, Figure S6B) and shorter action potential duration independent of rate (see Supplementary material online, Figure S6C and D). Ivabradine did not abolish GLP-1’s effect on cycle length (Figure 5E). High-density unipolar multi-electrode mapping of the sinus node area using a grid of 64 electrodes demonstrated that GLP-1 shifts the site of earliest activation towards the crista terminalis and inferior vena cava (Figure 5F and G; Supplementary material online, Figure S6E). This shift upon GLP-1 exposure also occurred in the presence of ivabradine (Figure 5H). There was no effect of GLP-1 on conduction velocity or average local activation time (see Supplementary material online, Figure S6F and G). These data support a direct electrophysiological effect of GLP-1 on pacemaker cells which is independent of HCN channels.
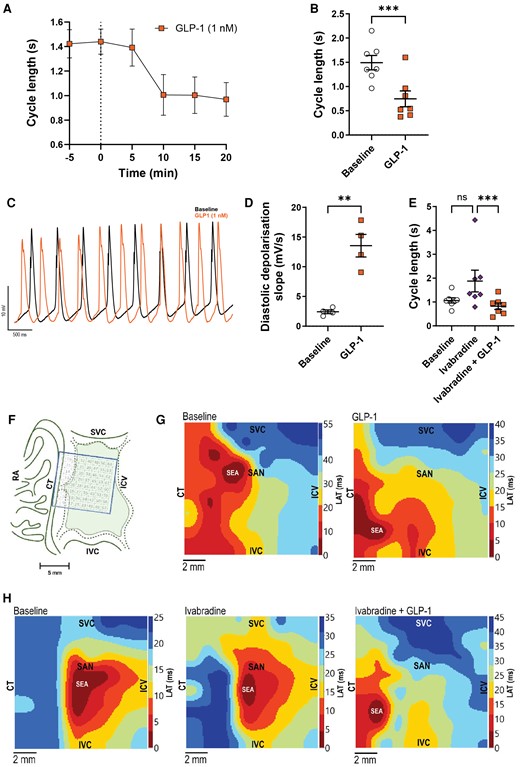
GLP-1 decreases cycle length and shifts the site of earliest activation in the isolated superfused pig sinus node independent of HCN channel block. (A–H) Isolated superfused sinus node tissues were exposed to GLP-1 (1 nM), ivabradine (1 µM), or both compounds combined. (A) Effect of GLP-1 on action potential cycle length over time (n = 7). (B) Quantification of the effect of GLP-1 on sinus node action potential cycle length (n = 7). (C) Representative monophasic action potential traces recorded at baseline (black) or after GLP-1 superfusion (orange). Note differences in rate and diastolic depolarization. (D) Diastolic depolarization (dV/dt) at baseline and after GLP-1 superfusion (n = 4), identifying a steeper diastolic depolarization during GLP-1 exposure (for method of analysis, see Supplementary material online, Figure S7B). (E) GLP-1 shortened sinus node cycle length in the presence of HCN channel blocker ivabradine (n = 7). (F) Illustration showing mapping electrode array placement on the sinus node. (G) High-density maps of activation times were measured across the array (n = 4). Representative maps at baseline (left) and after GLP-1 superfusion (right) are shown. Note the shift in site of earliest activation towards the crista terminalis. (H) Representative high-resolution maps of activation times measured at baseline (left) and in the presence of ivabradine (middle) and ivabradine + GLP-1 (right). Ivabradine did not affect the shift in site of earliest activation by GLP-1. Statistical tests: paired t-test (B and D) and a Friedman test with Dunn’s multiple comparison testing (E); **P < 0.01 and ***P < 0.001. CT, crista terminalis; ICV, intercaval vein; IVC, inferior vena cava; LAT, local activation time; RA, right atrium; SAN, sinoatrial node; SEA, site of earliest activation; SVC, superior vena cava.
3.7 The GLP-1 receptor is expressed in sinus node pacemaker cells
Given the robust increase in heart rate in the isolated perfused pig heart, which was dependent on the GLP-1 receptor, we evaluated the expression of the GLP-1 receptor within the sinus node using snRNAseq on nuclei isolated from anatomical preparations of pig sinus node tissues. The anatomical preparation contains the sinus node, confirmed by positive hyperpolarization-activated cyclic nucleotide–gated channel 4 (HCN4) staining, as well as surrounding atrial tissue (see Supplementary material online, Figure S7). Nuclei isolation from the anatomical preparation was quality controlled by FACS sorting (see Supplementary material online, Figure S8) prior to measurements. All transcript data from the single nuclei isolated are provided in Supplementary material online, Table S1. A total of 7971 nuclei were profiled covering 13 different cell types (Figure 6A). GLP-1 receptor was expressed at low transcript abundance in 413 nuclei and exclusively expressed within a subset of cardiomyocytes (Figure 6B; Supplementary material online, Figure S8D). The expression of GLP-1 receptor overlapped with canonical pacemaker cell markers, such as HCN4 and calcium voltage-gated channel subunit alpha1 D (CACNA1D) (Figure 6C and D). Collectively, these data provide compelling evidence for the expression of GLP-1 receptor in pacemaker cells of the porcine sinus node.
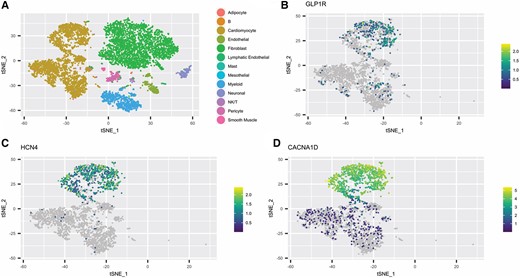
GLP-1 receptor localizes to pacemaker cells in the pig sinus node. (A–D) Neighbour embedding of snRNAseq of isolated nuclei from porcine sinoatrial tissue (n = 2), which predominantly contains cardiomyocytes and fibroblasts. Expression of GLP-1 receptor (B), HCN4 (C), and CACNA1D (D) in cardiomyocytes. snRNAseq, single nucleus RNA sequencing.
3.8 GLP-1 administration modulates the phosphorylation state of calcium cycling proteins in the sinus node
We speculated that the increased heart rate may result from phosphorylation changes elicited in the pacemaker cells expressing GLP-1R. We therefore performed an investigation of changes in the sinus node phosphoproteome induced by GLP-1. Four anaesthetized pigs received saline infusion, whereas another four pigs were infused with GLP-1 (Figure 7A). Heart rates of GLP-1-infused pigs were increased, as expected. After 1 h of GLP-1 infusion, anatomical preparations including the sinus node were collected (see Supplementary material online, Figure S7). The workflow of the sample processing is illustrated in Figure 7B. In brief, anatomical regions containing the sinus node were excised and proteins were extracted and enzymatically digested to peptides that were tagged with TMT prior to mixing, enrichment of phosphorylated peptides, fractionation, and measurement by high-resolution mass spectrometry. TMT labelling efficiency was >96%, and the peptide enrichment resulted in 86% of the 27 448 measured peptides being phosphorylated (see Supplementary material online, Figure S9A–D). For 14 395 phosphorylated peptides, localized to 3767 distinct proteins, we could quantify the abundance across all samples measured without any missing values. Notably, we could allocate the phosphorylation events to specific residues in 10 240 of these peptides, representing class I phosphorylation sites. Data for all quantified phosphorylation events are provided in Supplementary material online, Table S2. Pearson correlation coefficients for measured phosphopeptide intensities were above 0.92 for all samples (Figure 7C), underscoring high technical reproducibility. The signalling response evaluated is elicited in a cell population estimated from the snRNAseq data to account for ∼5% of the anatomical sinus node preparation and is as such expected to be small. Principal component analysis of the phosphoproteome data classified the samples according to the GLP-1 variable along the third component (Figure 7D), estimating the separation of Vehicle from GLP-1-treated animals to account for 8% of the variation in the phosphoproteome data set. Phosphorylation site-centric analyses for pig samples are challenged by limited site-specific information; therefore, we adopted a protein-centric approach to identify global pathways regulated by GLP-1 treatment. We analysed the global phosphoproteome data using gene set enrichment analysis to identify Kyoto Encyclopedia of Genes and Genomes (KEGG) pathways overrepresented in the GLP-1-infused hearts. This analysis highlighted an enrichment of pathways involved in calcium signalling in hearts stimulated with GLP-1 (Figure 7E; Supplementary material online, Table S2). Figure 7F depicts a gene-concept network plot displaying the proteins contributing to the overrepresentation of the four pathways: calcium signalling, cAMP signalling, insulin signalling, and adrenergic signalling in cardiomyocytes. The colour of the protein node indicates the fold change of the most regulated phosphorylation site on the protein. Information on all phosphorylation sites is also provided in Supplementary material online, Table S2. A small network of Ca2+ signalling proteins shared across pathways are highlighted in the network. Dynamic regulation of Ca2+ cycling proteins of the sarcoplasmic reticulum (SR) is a mechanism to regulate pacemaker rate48,49; we observed that GLP-1 induces phosphorylation of Ca2+ cycling proteins known to regulate heart rate. Specifically, the signature phosphorylation downstream of cAMP-induced protein kinase A (PKA) activation, phosphorylation of phospholamban on serine 16, is up-regulated (Figure 7F and G; Supplementary material online, Figure S9E). To evaluate the observed response, we measured cAMP from sinus node superfusates collected right before and 15 min after GLP-1 exposure, as well as in supernatants from sinus nodes statically incubated with or without GLP-1 for 30 min. The cAMP abundance was generally low in the samples, but showed a trend towards increased cAMP following GLP-1 exposure (see Supplementary material online, Figure S10). From the phophoproteome data, we also note regulated phosphorylation events on the ryanodine receptor; on the cardiac ion channels KCNQ1/Kv7.1, Nav1.5, and Cav1.2; on the sodium-calcium exchanger 1; on phosphodiesterase 3a and 4a; as well as on the calcium/calmodulin-dependent protein kinase IIs. That the overall phosphorylation signal is likely mediated by cAMP is further supported by the phosphorylation and activation of mitogen-activated proteins kinase ERK 1 and 2.
![Phosphoproteomics analysis suggests modulation of cAMP/Ca2+ signalling pathways in pig sinus node upon GLP-1 infusion. (A) Protocol of infusion of GLP-1 or vehicle prior to tissue collection (n = 4). (B) Workflow for phosphoproteomic experiments of pig sinus node tissues. Proteins were extracted from anatomical sections of sinus node tissue, tryptic digests were multiplexed with TMTpro reagents, and phosphopeptides were enriched using TiO2 beads. The enriched peptides were fractionated at high pH and analysed by LC-MS/MS. (C) Heatmap of Pearson correlation coefficients for all measured phosphorylated peptide intensities across all samples. (D) Principal component analysis (PCA) of phosphorylated peptide intensities displaying separation of the samples according to treatment along the third component. (E) Gene set enrichment analysis (GSEA) using phosphorylation fold changes as input identified enriched pathways consequent to GLP-1 infusion. For multi-phosphorylated proteins, the greatest phosphorylation fold change was used. Enriched pathways are indicated in the plot; for all pathways, the adjusted P-value was below 1e−5. The gene set size refers to the number of genes covered in our data set of the respective pathways, and the colour indicates the normalized enrichment score (NES). All proteins measured in our dataset for the pathways highlighted in bold are visualized in the network in panel F. (F) Gene–KEGG pathway network (cnetplot) displaying the proteins contributing to the overrepresentation of the four pathways shown. Nodes are coloured by the greatest phosphorylation event fold change measured for the protein. Proteins known to be involved in heart rate regulation of the sinus node are encircled by a dashed line. (G) Western blot analysis of sinus node samples from pigs infused with GLP1 (n = 4) or vehicle (n = 4). Representative data of phospholamban phosphorylated at amino acid serine 16 [p-PLN(S16)] and loading control (GAPDH). Upper panel: Comparison of ratios of p-PLN(S16)/total PLN intensities. Lower panel: one-way Mann–Whitney U test. AU, arbitrary units.](https://oup.silverchair-cdn.com/oup/backfile/Content_public/Journal/cardiovascres/120/12/10.1093_cvr_cvae120/1/m_cvae120f7.jpeg?Expires=1747849375&Signature=Gw2ykZ9un6uo11N0cJkjdeafRbpwo0v-6hn3aYv58C2Wme13w8wXlB5rF8R1n~VbXfPdhPGErx1dszqHP5-~EjVrJppkcqlA0wUjcgSzCqiPlNh6KDDPQoW9~vYw-l0ExmIWk-HDm8Y2MLGj75Gp9Z0AlQqqbtoINhCXWx~kFE9bIU7VtueHlFWIIP-QSq7k8ichoq4r~pIgZ~XExXbdLpWq7M9PJz3mVTORTxwWP~86LzlPB9yhZlSY5Zi2RSt8x~asLnzTS8ezwD9BsSjV~eDBKUnC0Ke1t7uFO0Bw4x9GfA3WWx8YN~vpYBr~cOasDWcCzrCSt6hOewHIyFlf0g__&Key-Pair-Id=APKAIE5G5CRDK6RD3PGA)
Phosphoproteomics analysis suggests modulation of cAMP/Ca2+ signalling pathways in pig sinus node upon GLP-1 infusion. (A) Protocol of infusion of GLP-1 or vehicle prior to tissue collection (n = 4). (B) Workflow for phosphoproteomic experiments of pig sinus node tissues. Proteins were extracted from anatomical sections of sinus node tissue, tryptic digests were multiplexed with TMTpro reagents, and phosphopeptides were enriched using TiO2 beads. The enriched peptides were fractionated at high pH and analysed by LC-MS/MS. (C) Heatmap of Pearson correlation coefficients for all measured phosphorylated peptide intensities across all samples. (D) Principal component analysis (PCA) of phosphorylated peptide intensities displaying separation of the samples according to treatment along the third component. (E) Gene set enrichment analysis (GSEA) using phosphorylation fold changes as input identified enriched pathways consequent to GLP-1 infusion. For multi-phosphorylated proteins, the greatest phosphorylation fold change was used. Enriched pathways are indicated in the plot; for all pathways, the adjusted P-value was below 1e−5. The gene set size refers to the number of genes covered in our data set of the respective pathways, and the colour indicates the normalized enrichment score (NES). All proteins measured in our dataset for the pathways highlighted in bold are visualized in the network in panel F. (F) Gene–KEGG pathway network (cnetplot) displaying the proteins contributing to the overrepresentation of the four pathways shown. Nodes are coloured by the greatest phosphorylation event fold change measured for the protein. Proteins known to be involved in heart rate regulation of the sinus node are encircled by a dashed line. (G) Western blot analysis of sinus node samples from pigs infused with GLP1 (n = 4) or vehicle (n = 4). Representative data of phospholamban phosphorylated at amino acid serine 16 [p-PLN(S16)] and loading control (GAPDH). Upper panel: Comparison of ratios of p-PLN(S16)/total PLN intensities. Lower panel: one-way Mann–Whitney U test. AU, arbitrary units.
4. Discussion
Multiple large randomized cardiovascular outcome trials on GLP-1 RAs have demonstrated reductions of major adverse cardiovascular events in T2D patients. The positive outcomes of the clinical trials have—understandably—led to a lot of excitement, and current guidelines in cardiology50 and diabetes51 now recommend the use of GLP-1 RAs to reduce cardiovascular risk in high-risk patients with T2D. The detailed molecular mechanisms behind the clinical cardiovascular benefits of the GLP-1 RAs are still being debated, but the clinical picture is consistent with an anti-atherogenic effect likely involving reduction of inflammation.52,53 Additionally, GLP-1 treatment clearly modifies several cardiovascular risk factors, including body weight, blood pressure, plasma glucose concentrations, plasma LDL cholesterol, and triglycerides.53 A perplexing conundrum is the positive chronotropic effect of GLP-1 RAs. Clinical trials in T2D patients have reported overall cardioprotective effects, but the increased heart rate effect could represent a concern in patients where increased heart rate is undesirable, for instance, in those at elevated risk of arrhythmic events, such as patients with chronic heart failure. Interestingly, however, genetic evidence supports repurposing of GLP-1 RAs for prevention of heart failure54 and a recent meta-analysis of the GLP-1 RA cardiovascular outcome trials identified an 11% reduction in hospital admission for heart failure with GLP-1 RA treatment.1 Recent dedicated studies in patients with heart failure with preserved ejection fraction and obesity found that treatment with semaglutide, a GLP-1 RA with marked weight loss effects, reduced body weight55 and heart failure-related symptoms10 and improved exercise function55 in these patients, in addition to reducing major cardiovascular events.55 These beneficial effects were observed despite a heart rate increase of 3.8 b.p.m.55 However, in dedicated studies of patients with heart failure with reduced ejection fraction, there was no beneficial cardiovascular effect of liraglutide.56–58 It was speculated that this might be related to arrhythmias related to the treatment.56 In line with the question of arrhythmia risk, a recent meta-analysis reviewed the association of GLP-1 RAs and incident arrhythmias. There was no significant increase in atrial fibrillation (in line with a previous meta-analysis59), atrial flutter, ventricular arrhythmia, or sudden cardiac death, although it was noted that higher doses of GLP-1 RAs may increase the risk of ventricular arrhythmia.60 Ultimately, understanding the mechanisms of the chronotropic effect could be important to identify strategies to prevent heart rate increases in GLP1 RA recipients where this might be considered beneficial.
Here, we conducted a set of in vivo and ex vivo physiological measurements to evaluate the effect of GLP-1 exposure on the electrical and functional activity of the heart. We used a robust large animal experimental model, the female landrace pig, with cardiac electrophysiological properties akin to humans45 and where repeatable responses to GLP-1 infusions allowed for evaluation of interventions in a paired design. We were unable to block the GLP-1-elicited increase in heart rate using sympathetic blockers, an HCN channel blocker, cervical vagotomy, and ganglionic blockade. Even in the isolated perfused pig heart and in isolated superfused pig sinus nodes, GLP-1 induced a sustained rate increase, which could be blocked by pre-administration of the GLP-1 receptor–specific blocker exendin 9-39, but not by administration of the HCN channel blocker ivabradine.
There are several reports that GLP-1 RAs may influence autonomic nervous function, though with important species-specific differences. GLP-1 may activate autonomic control sites in the rat brain, including medullary catecholamine neurons providing input to sympathetic preganglionic neurons,38 and central GLP-1 receptor stimulation was shown to increase blood pressure and heart rate in rodents.37 Accordingly, in both mice and rats, the increase in heart rate associated with GLP-1RAs was abolished by beta-adrenergic blockade.35,39 Further supporting the involvement of the autonomic nervous system in the positive chronotropic effect of GLP-1 in rodents is the lack of heart rate increases in response to GLP-1 in isolated perfused rat and mouse hearts.35,39,61 Contrary to this, in rabbit hearts, Jøns et al.40 reported that the chronotropic effect of the GLP-1 RA liraglutide was mediated by a direct effect on the heart, which was found to be independent of beta-adrenergic signalling, and also present in murine cardiomyocytes isolated from the sinus node. In the present study, we did not observe any effect of adrenergic blockers on the GLP-1-elicited increase in heart rate in pigs. This is in line with clinical observations, as the chronotropic effect of chronic administration with liraglutide in the LIVE study was observed despite concomitant treatment with maximum tolerable beta-blocker doses.56
There is also strong evidence that GLP-1 may influence vagal function, which has been implied to be involved in the GLP-1-associated increase in heart rate in mice.36 Not only does GLP-1 activate vagal afferents,62 but in pigs, it can be demonstrated to inhibit efferent vagal outflow with important consequences for gastric secretion and motility, as well as pancreatic exocrine secretion.63 Also in humans, GLP-1 seems to inhibit vagal regulation of gastro-pancreatic secretion and motility.64 A mechanism involving the vagal regulation of heart rate would therefore seem probable but was clearly not responsible for the heart rate responses observed here, since vagotomy had no effect.
In the isolated perfused heart experiments, we observed that GLP-1 did not affect heart rate in hearts that were not in sinus rhythm. This observation is consistent with clinical data from the LIVE study, where it was reported that the GLP-1 RA liraglutide did not increase heart rate in patients not in sinus rhythm,65 although these patients had atrial fibrillation, flutter, or pacing, compared with accelerated ventricular rhythm in our isolated perfused hearts. Additionally, we observed that the GLP-1 receptor blocker exendin-9-39 caused a decrease in heart rate. This could be caused by residual GLP-1 in the OCS system. This is supported by observations that baseline heart rate tends to decrease over time in this set-up (∼9 b.p.m. after 1–1½ h, unpublished data). Alternatively, exendin-9-39 could function as an inverse agonist, as previously described for the murine GLP-1 receptor,66 although it is not known whether this is the case in pigs or in the heart.
Monophasic action potential recordings and high-density electrode array mapping of the sinus node area identified electrophysiological characteristics of GLP-1’s effect on the sinus node. The increase in rate coincides with a steeper diastolic depolarization potential, shortening of the action potential duration, and a shift in the leading pacemaker site, all changes previously associated with a variety of neurohumoral factors67–69 but here identified to be independent of these. Our results in the porcine heart thus point to a direct action on the heart, involving the sinus node and GLP-1 receptor, whereas effects via autonomic nervous regulation could not be demonstrated. The in vivo and ex vivo experiments herein support that the mechanism underlying the chronotropic effect of GLP-1 is intrinsic to the heart.
The question of the presence and localization of GLP-1 receptors in the heart, as well as species-specific expression patterns herein, has been ongoing since the realization that the GLP-1 RAs invariably increase heart rate in humans.70 What animal species is best suited for studies of human heart incretin physiology and pharmacology remains to be determined. The GLP-1 receptor was localized to the atria in rodents,71 but in humans and monkeys, it was found by immunohistochemistry and ligand binding to be localized exclusively to the sinus node.42 However, Wallner et al.72 detected GLP-1 receptor mRNA transcripts in RNA isolated from human atria as well as the right and left ventricles, including in RNA from isolated cardiomyocytes. More recently, Baggio et al. studied the localization of GLP-1 receptor expression in the human heart using RT-PCR, qPCR, in situ hybridization, and immunocytochemistry. GLP-1 receptor expression was detected within all four chambers of the human heart at levels similar to those of the human pancreas, and GLP-1 receptor could be localized to cells within the sinus node, but no definitive localization of GLP-1 receptor expression was detected within cells of the left ventricle.43 Recently, an informatics approach combined with detailed human heart single cell resolution data highlighted pacemaker cells as cellular targets for GLP-1 analogues.44 Consistently with the human data, we here report the presence of GLP-1 receptor transcripts specifically in the pacemaker cells of the porcine sinus node. The observed shift in the site of earliest activation, or leading pacemaker site, in our studies, may also indicate that GLP-1 receptor expression is not uniform across the population of sinus node cells. Adrenergic and muscarinic receptors have been reported to be differentially expressed in different sites of the sinus node, consistent with the shifts in sites of earliest activation upon autonomic modulation.68 Similarly, expression of the GLP-1 receptor may differ across the sinus node, leading to different localization of the site of earliest activation upon GLP-1 exposure.
The question then remains, what signal is elicited in the pacemaker cells upon GLP-1 receptor activation, and how does this translate to increased heart rate? The sinus node autonomously generates action potentials, regulating the automated activity of the heart. Two processes contribute towards this: the membrane clock, linked to the activity of sarcolemmal ion channels and dominated by the HCN4 channel, and the calcium clock, driven by intracellular calcium signalling. We observed a sustained effect on pacemaker rate despite ivabradine blockade, both in vivo and in the isolated superfused sinus node, suggesting that GLP-1’s mode of action is not mediated via HCN channels. Further molecular experiments were consistent with the in vivo experiments. The quantitative phosphoproteomics analyses performed on sinus node samples isolated from porcine hearts excised after infusions of GLP-1 or saline in vivo identified phosphorylation-mediated signalling changes of calcium cycling proteins of the SR. Consistently, previous studies in HL-1 cells have reported calcium handling changes in response to GLP-1 exposure,73 and others have reported cAMP-dependent responses in murine pacemaker cells,40 though the latter study reported a GLP-1 RA response which, contrary to our findings, was sensitive to ivabradine blockade of the HCN channels. We previously evaluated expression profiles of murine sinus nodes and did not find particular pacemaker cell expression for GLP-1 receptor in murine sinus node,74 which may in part explain some of the species differences found between the present study and studies in mice.40 The phosphorylation-mediated signalling response we observe in the sinus node resembles the signalling response elicited by increased levels of cAMP and the canonical signalling mechanism associated with activation of the GLP-1 receptor, e.g. in pancreatic beta cells. In line with this, cAMP tended to be increased in supernatants of sinus nodes exposed to GLP-1, although we did not find a statistical difference. cAMP mediates PKA-dependent phosphorylation of Ca2+ cycling proteins of the SR in pacemaker cells, which influences pacemaker rate through regulation of SR Ca2+ loading and release characteristics; regulation of the Ca2+ cycling proteins of the SR is a common mechanism for pacemaker rate regulation.75 We also identified phosphorylation of several other targets, including ion channels and exchangers, that could be involved in the GLP-1-induced response. Additionally, cAMP can activate HCN channels independent of protein phosphorylation.76 However, our functional findings in vivo and ex vivo suggest that HCN channels are not required for the GLP-1-induced increase in heart rate. Based on the phosphoproteomics data, we suggest that GLP-1 engages a cAMP-mediated signalling mechanism that involves Ca2+ cycling proteins and possible other downstream targets, which converges with beta-adrenergic signalling downstream of cAMP. It is tempting to speculate that treatment of GLP-1 RAs might be combined with drugs targeting calcium clock dynamics or intracellular calcium levels, such as calcium channel blockers, or other downstream effectors, to prevent heart rate increases in patients receiving GLP-1 RAs where this may be considered beneficial.
In conclusion, we have demonstrated by in vivo and ex vivo experiments carried out in pigs that GLP-1 increases heart rate via signalling mechanisms intrinsic to the heart. Using snRNAseq, we localized the expression of the GLP-1 receptor to the porcine sinus node and phosphoproteomics analyses suggest that GLP-1 receptor activation regulates key proteins involved in calcium signalling pathways in the sinus node. Future studies should investigate whether the chronotropic effect of GLP-1 can be blocked by manipulating the calcium clock in the sinus node.
Despite improved cardiovascular outcome associated with glucagon-like peptide 1 receptor agonists (GLP-1 RAs), they increase resting heart rate, the mechanism of which remains unclear. Our results unveil a molecular mechanism of the chronotropic effect of GLP-1 in pigs that is independent of autonomic innervation; is mediated through the GLP-1 receptor, which is expressed in sinus node cells; and involves electrophysiological changes in sinus node firing mediated by changes in calcium clock signalling. The calcium clock of the cardiac pacemaker could thus be a target to prevent or reduce the increase in heart rate in patients receiving GLP-1 RAs.
Supplementary material
Supplementary material is available at Cardiovascular Research online.
Authors’ contributions
Animal experiments shown in Figures 1A and B and 4 and Supplementary material online, Figures S1, S4, and S5, were performed by A.F.L. with support from S.V., S.M.S., B.L., and C.H.E.E.; analysed by A.F.L., S.M.S., B.L., and C.H.E.E.; and supervised by T.J., J.T.-H., and J.J.H. Animal experiments shown in Figures 1C, 2, and 3 and Supplementary material online, Figures S2 and S3, were performed by S.V. with support from S.T., S.A.L., A.N., and L.G.P., with sample analysis by B.H., data analysis by S.V. and A.F.L., and supervision by J.J.H. Transplantation and maintenance of hearts in the OCS set-up was performed by S.Q. and T.N.B.L. and supervised by C.H.M. Superfusion experiments of sinus node tissue were performed by L.S., A.F.L., and S.D.N., analysed by L.S., and supervised by B.H.B., with cAMP measurements supervised by M.M.R. Single-cell RNA sequencing was performed by S.E.B., analysed by J.M. on samples prepared by S.V. and S.D.N. and supervised by A.L. Phosphoproteomics experiments were performed by A.S., J.S.A., and E.T.V., analysed by J.S.A., and supervised by A.L. Western blot was performed by E.T.V., A.F.L., S.V., A.L., and J.J.H. conceived the project, designed the experiments, critically evaluated results, and wrote the manuscript. All authors provided input for the manuscript.
Acknowledgements
The authors would like to thank Charlotte Kyhn Fink, Jannie Brøndkær Holm Jørgensen, Karsten Pharao Hammelev, and Embla Arnorsdottir from the Department of Experimental Medicine, University of Copenhagen, and Søren Petersen from the Department of Biomedical Sciences, University of Copenhagen, for expert technical assistance.
Funding
This work was supported by a grant from the Novo Nordisk Foundation (NNF 18CC0034900) to J.J.H., grants from the Novo Nordisk Foundation (NNF20OC0059767) and from the Carlsberg Foundation (CF17-0209) to A.L., as well as postdoctoral fellowship from the Lundbeck Foundation to A.S. (R322-2019-2698). Mass spectrometry analyses were performed at the Proteomics Research Infrastructure at the University of Copenhagen supported by the Novo Nordisk Foundation (NNF19SA0059305).
Data availability
Mass spectrometry proteomics and phosphoproteomics data have been deposited in the ProteomeXchange Consortium through the PRIDE partner with the dataset identifier PXD042583. Additional information can be made available from the corresponding authors on reasonable request.
References
Author notes
Anniek Frederike Lubberding and Simon Veedfald equally contributed first authorship.
Jonathan Samuel Achter, Sarah Dalgas Nissen and Luca Soattin equally contributed second authorship.
Jens Juul Holst and Alicia Lundby equally contributed last authorship.
Conflict of interest: J.J.H. has been on advisory boards for Novo Nordisk. The other authors report no conflict of interest.