-
PDF
- Split View
-
Views
-
Cite
Cite
Christian Bär, Shambhabi Chatterjee, Inês Falcão Pires, Patrícia Rodrigues, Joost P G Sluijter, Reinier A Boon, Rosa M Nevado, Vicente Andrés, Marida Sansonetti, Leon de Windt, Michele Ciccarelli, Nazha Hamdani, Stephane Heymans, Raquel Figuinha Videira, Carlo G Tocchetti, Mauro Giacca, Serena Zacchigna, Stefan Engelhardt, Stefanie Dimmeler, Rosalinda Madonna, Thomas Thum, Non-coding RNAs: update on mechanisms and therapeutic targets from the ESC Working Groups of Myocardial Function and Cellular Biology of the Heart, Cardiovascular Research, Volume 116, Issue 11, 1 September 2020, Pages 1805–1819, https://doi.org/10.1093/cvr/cvaa195
- Share Icon Share
Abstract
Vast parts of mammalian genomes are actively transcribed, predominantly giving rise to non-coding RNA (ncRNA) transcripts including microRNAs, long ncRNAs, and circular RNAs among others. Contrary to previous opinions that most of these RNAs are non-functional molecules, they are now recognized as critical regulators of many physiological and pathological processes including those of the cardiovascular system. The discovery of functional ncRNAs has opened up new research avenues aiming at understanding ncRNA-related disease mechanisms as well as exploiting them as novel therapeutics in cardiovascular therapy. In this review, we give an update on the current progress in ncRNA research, particularly focusing on cardiovascular physiological and disease processes, which are under current investigation at the ESC Working Groups of Myocardial Function and Cellular Biology of the Heart. This includes a range of topics such as extracellular vesicle-mediated communication, neurohormonal regulation, inflammation, cardiac remodelling, cardio-oncology as well as cardiac development and regeneration, collectively highlighting the wide-spread involvement and importance of ncRNAs in the cardiovascular system.
1. Introduction
The completion of the human genome project in 2003 had spurred great hope to identify underlying mechanisms and to find cures for pandemic diseases such as cancer and cardiovascular disease (CVD). Unfortunately, even 20 years later, CVDs are still on the rise accompanied by lack of effective therapeutic options. This is partially because most of the drug development has been focused on protein-coding genes. Considering that the human phenotype is not only dictated by protein-coding genes but also by genes which give rise to non-coding RNA (ncRNA) transcripts,1 recently more studies have been directed towards detailed investigation of such ncRNA molecules for CVD diagnostic and treatment options.2,3 The class of ncRNA molecules which make up to 98% of the human transcriptome comprises a wide variety of transcripts with numerous functions. MicroRNAs (miRs), long non-coding RNAs (lncRNAs), and circular RNAs (circRNAs) represent the major classes of ncRNAs involved in cardiovascular development and pathology (Table 1). The short miRs (∼20nt) mainly interact with the 3′-untranslated region of the target mRNA, thereby suppressing protein translation through RNA-interference mechanisms.4 The lncRNAs comprise a large group of extremely diverse molecules which are defined as ncRNA >200nt in length and are known to regulate gene expression both at the nuclear and cytoplasmic levels.5 They can influence gene expression and cellular function of all cardiac cell types playing pivotal roles in CVDs.6 The more recently discovered circRNAs are covalently closed RNA rings formed through alternative back-splicing of protein-coding exons. Their function may range from host gene regulation to scaffold and molecular sponge function.7 CircRNAs have also been implicated in the regulation of several cellular and pathological functions in the heart.8 While the biogenesis and the principle functional mechanisms of the different types of ncRNA have been reviewed in detail recently,7,9,10 in here, we will mainly highlight crucial roles of ncRNAs in cardiac development and disease progression. The importance of ncRNA in this field is reflected by the significant and increasing research efforts by members of the Working groups on Myocardial Function and Cellular Biology of the Heart of the European Society of Cardiology. As a follow up of the 2019 meeting in Naples, we will here provide an overview of the state-of-the-art of cardiovascular ncRNA research of both ESC Working groups focusing on different aspects of heart development, cardiac disease as well as regeneration (Figure 1). Importantly, since ncRNAs have made the first steps from basic science into clinical application, we will provide an outlook on the therapeutic perspective of ncRNAs.
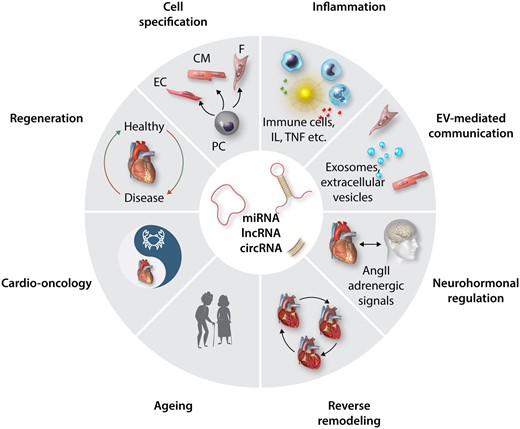
Overview of diverse cardiac processes which underlie ncRNA control. Recent research identified a number of ncRNAs including miRs, lncRNAs, and circRNAs in the depicted physiological and pathological processes which are subject of ongoing research in the ESC Working Groups of Myocardial Function and Cellular Biology of the Heart and which will be highlighted in the subsequent chapters. PC, progenitor cells; EC, endothelial cells; CM, cardiomyocytes; F, Fibroblasts; IL, interleukins; TNF, tumour necrosis factors; EV, extra-cellular vesicles; AngII, AngiotensinII.
ncRNA type . | Mechanisms of action . | Ref . |
---|---|---|
miRNA |
| 11,12 |
lncRNA |
| 6,13 |
circRNA |
| 7,8 |
ncRNA type . | Mechanisms of action . | Ref . |
---|---|---|
miRNA |
| 11,12 |
lncRNA |
| 6,13 |
circRNA |
| 7,8 |
ncRNA type . | Mechanisms of action . | Ref . |
---|---|---|
miRNA |
| 11,12 |
lncRNA |
| 6,13 |
circRNA |
| 7,8 |
ncRNA type . | Mechanisms of action . | Ref . |
---|---|---|
miRNA |
| 11,12 |
lncRNA |
| 6,13 |
circRNA |
| 7,8 |
2. Circular RNA: new kid on the block with specific challenges
CircRNAs comprise more recently described ncRNAs which differ from linear RNAs as they are covalently closed, do not possess strand polarities, and are generated in a process termed as back-splicing in which a downstream sequence is spliced to an upstream one.14 Although we are just beginning to unravel the molecular mechanisms of circRNAs, several studies have addressed the expression in the cardiovascular system and have assigned functions to some circRNAs. In the vascular system, cZNF292 was first identified among the >7.000 circRNAs in endothelial cells (ECs) to control EC and angiogenic sprouting in vitro.15 Various subsequent studies documented that several circRNAs affect vascular cell functions in vitro (for a comprehensive overview, see Refs8,16). A prominent example is the circular form of the ncRNA ANRIL, circANRIL, which is induced by coronary artery disease and regulates smooth muscle cells (SMCs), specifically cell death and proliferation, by interfering with ribosomal RNA maturation.17 This effect is mediated by binding to the pre-ribosomal assembly factor PES1. More recently, a circular transcript lipoprotein receptor 6, circLrp6, was identified as a crucial regulator of vascular SMCs by sponging of and counterbalancing miR-145.18
Further circRNAs were identified to control cardiac functions (for a comprehensive review, see Ref.14). Interesting examples include circFoxo3a, which aggravated doxorubicin-induced cardiomyopathy. CircFoxo3a primarily affected senescence, possibly by interacting with the senescence inhibitory protein and the transcription factors E2F1 and HIF1α.19 The highly abundant circSlc8a1 contributes to pressure overload-induced hypertrophy by sponging miR-133.20 A recent study further elegantly demonstrates that circFndc3c modulates cardiac repair after myocardial infarction (MI). Interestingly, the authors report a circRNA-protein interaction with the RNA-binding protein ‘fused in sarcoma’ (FUS), which affects vascular endothelial growth factor-A (VEGF-A) expression and subsequent cardiomyocyte (CM)-EC cross-talks.21
As mentioned above, circRNA research is still in its infancy and comes with specific challenges for this somewhat peculiar type of RNA. While overexpression and RNA silencing mediated deletion of circRNAs demonstrated functional roles in the cardiovascular system, the field still suffers from the lack of definitive genetic evidence confirming the proposed functions in vivo.8,16 This limitation is predominantly due to the fact that it is challenging to specifically interfere with the circularization without affecting the expression or the splicing of the host gene, which in most cases has also important functions as coding RNA. The diagnostic use and therapeutic targeting of circRNA is also more challenging as compared to miRs. The diagnostic potential is limited due to the fact that circRNAs are expressed at low levels and often come in various spliced isoforms, which makes their specific detection in the blood very challenging. The therapeutic application of circRNAs requires the overexpression of transcripts, which can be done using viral vectors containing flanking regions facilitating circularization.8 While this strategy was successfully used by many investigators, it is often neglected that the circularization is quite ineffective, leading to the co-expression of linear parts of the gene, which may have effects as well. Alternatively, recombinant circles can be generated, but their up-take is limited without delivery vehicles such as liposomes or nanoparticles. It is unclear if the major achievements in siRNA delivery strategies, for instance linking them to molecules that target the siRNA to specific cell types (e.g. GalNac for liver cells; Ref.22) can be used as well for circRNAs. Inhibition of circRNA expression is also hampered by the fact that silencing strategies to specifically target the circRNA are limited to the backsplice site, thereby reducing the flexibility of RNA sequences that can be used. Finally, the molecular mechanism of action is not always very compelling. Many studies reported that circRNAs act via sponging of miRs. However, given that miRs are expressed in much higher copy numbers, this mechanism of action depends on relatively high expression of the circRNA and/or the existence of many miR binding sites in the circRNA. Since a cross-talk between lowly expressed circRNAs with highly expressed miRs has been experimentally validated in various cases, one may need to find an alternative explanation how circRNA may interfere with the processing or localization of miRNAs or consider alternative interactions in RNA networks.
3. Non-coding RNAs in extracellular vesicle-mediated communication in the heart
In the past decade, extracellular vesicles (EVs) have come to light as novel elements of cell-to-cell communication in the cardiovascular system, not only constitutively released from many cardiac cell types, including CMs, fibroblasts (FBs), ECs, inflammatory cells, and resident stem cells but also detected in most body fluids.23 This EV-mediated way of intercellular communication is critical in physiological and pathological cardiovascular circumstances by allowing the exchange of biological information and therefore, the coordination of cell/organ and maintenance of homoeostasis.24–26
Incorporation of miRNAs in vesicles is not random as they are selectively exported to EVs at constant ratios, varying under specific pathophysiological conditions.27 Following delivery, miRNA-enriched EVs can exert functional roles in recipient cells, orchestrating their entire gene programs and affecting their phenotype. Recent developments in the field of intercellular cross-talk, demonstrate that EVs enriched in specific miRNAs could be key players during different cardiac disorders.26,28
The release of EVs, including exosomes, is a common way of communication between different cardiac cells such as CMs and ECs.29 Halkein et al.29 demonstrated that during peripartum cardiomyopathy, miR-146a-enriched EVs released by ECs are taken up by CMs and, by interfering with their physiological metabolism, affect contractility and lead to CM hypertrophy. Reciprocally, increased levels of miR-143 and miR-222 in EVs released by ischaemic CMs exert a pro-angiogenic effect on recipient ECs.30 Moreover, CMs from diabetic rat hearts were shown to release EVs enriched in miR-320 which, once delivered to cardiac ECs, compromised their proliferation, migration, and tube formation capacity, leading to impaired angiogenesis.31 In a recent study, CM-derived EVs were reported to increase cardiac angiogenesis and CM survival as a result of EV-mediated miR‐21-5p transfer,32 and CM autophagy through the uptake of miR-30a.33
Hergenreider et al.34 demonstrated that ECs can also use EVs to transfer miR-143/145 to SMC cells and reduce atherosclerotic lesion formation. Under atherosclerotic conditions, enrichment of miR-155 in endothelial EVs were responsible for modulating the phenotype of recipient monocytes and/or macrophages in vivo and in vitro.35
Extremely relevant is the cross-talk among ECs; indeed these cells seem to be particularly enriched of miR-214 that once released, it targets other recipient ECs, thereby stimulating angiogenesis.36 In accordance with this, Balkom et al.37 proved miR‐214 to be released from ECs contributes to EV-mediated angiogenesis and migration in neighbouring recipient cells. Among the different cardiac cells, also cardiac FB-derived EVs have been shown to play a relevant role in many CVDs. In mice subjected to cardiac pressure overload, miR-21-3p (miR-21*) is up-regulated and transferred from cardiac FBs to CMs through EVs, inducing hypertrophy in the recipient cells.38EV-mediated cross-talk was also reported between the major cardiac cell types and cardiosphere-derived cells,39 mesenchymal stem cells,40,41 and pluripotent stem cells (induced and embryonic).42,43
Although less intensively studied to date, lncRNAs and circRNAs were also identified in cardiac EV-mediated cell-to-cell communication.44 For example, RNA-enriched EVs secreted by hypoxic CMs were demonstrated to drive cardiac fibrosis.45 Nevertheless, further investigations are necessary to understand the molecular mechanisms which elicit and are driven by EV-mediated intercellular communication.
4. Reciprocal regulation of ncRNAs and the neurohormonal system
The neurohormonal system is a pivotal contributor to organ homoeostasis, as well as responsible for the adaptive mechanisms observed in chronic conditions such as HF.46 In turn, ncRNAs modulate cellular phenotypes through the regulation of gene expression at the transcriptional and translational level.6 Therefore, it may be plausible that these systems are tightly interconnected with reciprocal influence in terms of receptors expression and signal transduction. These connections are particularly evident in the pathophysiological conditions such as HF and hypertension, where the dysregulation of the neurohormonal system is involved in the development and progression of the disease.
In HF, the beta-adrenergic receptor stimulation can regulate miRNA expression in the animal model, and thus, mediate the effects on cardiac remodelling. For example, miRNA-214 is up-regulated following chronic isoproterenol stimulation in rat and promotes cardiac FB proliferation as well collagen production and fibrosis by regulating the target gene Mfn2 and its downstream ERK1/2 signalling pathway.47 Moreover, miRNAs can directly interfere with the adrenergic signalling by modulating receptor expression and as well as components of its intracellular pathway.48 For example, miR-133 can directly target the 3′-UTR of the β1-adrenergic receptor (β1AR) and its downstream effectors, thereby limiting the cAMP production and its deleterious effects in the presence of adrenergic overdrive.49
This picture, however, is more complicated when considering that multiple miRs can impinge the expression of molecules belonging to the neurohormonal signalling. miR-155 was found to target the 3′-UTRs of the angiotensin II type I receptor (AGTR1).50miR-125a/b down-regulates the expression of endothelin 1 in vascular ECs.51miR-766 down-regulates the expression of the aldosterone synthase gene, CYP11B2.52 Beneficial seems to be miR-425 which reduces the expression of atrial natriuretic peptide (NPPA),53 while miR-100 negatively regulates expression of the natriuretic peptide receptor 3 (NPR3), the clearance receptor for natriuretic peptides, in cardiac derived cells.54
In summary, miRs interfere with the expression of both hormones and their cognate receptors and, vice versa, hormones seem to control the expression of miRs, highlighting that the regulation of blood pressure level depends upon the dynamic interactions between those factors. It is likely that lncRNAs and circRNAs play important roles in neurohormonal regulation, however, this topic requires further research.
5. Non-coding RNAs in inflammatory responses
Metabolic syndrome represents a cluster of cardiovascular risk factors, including hypertension, insulin resistance, hyperlipidaemia, and obesity that are associated with increased risk of heart failure (HF). These comorbidities are characterized by chronic inflammation.55 Inflammation is not only critical for the development and progression of HF but the inflammatory response is also important for adverse remodelling processes following myocardial infarction.
Inflammation and oxidative stress are major sources of both endogenous, for example, sterile inflammation56 and exogenous challenges that promote HF phenotypes. There is a physiological interaction that links inflammatory and oxidative stress processes, to the activation of downstream networks that promote the physiological characteristics of various human pathologies, including aging, carcinogenesis, neurodegenerative disorders, and HF associated with various causes and phenotypes.57–59
In view of the manifold ncRNA mechanisms in the regulation of cardiovascular inflammation (recently reviewed by others; Ref.60), we will focus here on the implication of ncRNAs as immune regulators of the susceptibility to myocarditis upon cardiac viral infection. Human and experimental miR expression studies reveal a strong association between miR dysregulation and human myocarditis and suggest novel miRNA therapeutic targets.61 Indeed, inhibition of miR-155,62–64 -21, and -146 b65 by systemically delivered anti-miRs reduces cardiac inflammation and damage in CVB3- or auto-immune myocarditis in mice. Cardiac overexpression of miR-590-3p also prevents cardiac injury and dysfunction by inhibiting p50 expression, suppressing NF-κB activity and blocking IL-6/TNF-α expression.66 miRs may also modulate the virulence of cardiotrophic viruses.67 The miR-221/222 cluster in CMs regulates both virulence and inflammatory pathways in the heart.68 Systemic inhibition of miR-221/-222 in mice increases cardiac viral load, prolongs the viremic state, and aggravates cardiac inflammation and injury. Mechanistically, miR-221/-222 targets the expression of proteins that orchestrate viral replication and inflammation, including ETS1/2, IRF2, BCL2L11, TOX, BMF, and CXCL12. Similarly, miRNA-155 inhibits PU.1 and SOCS1 in the heart and as such de-represses the production of pro-inflammatory cytokines, enhancing T-cell and monocyte activation.68,69 Together, these results support the concept that a single miR or miR clusters may orchestrate immune activation and modulate myocarditis.
Very limited knowledge exists on the contribution of lncRNAs to viral myocarditis. A very recent report revealed that the loss of lncRNA AK085865 in macrophages promotes the polarization to M1 phenotype at the detriment of M2 macrophage levels, which increases the susceptibility of mice to coxsackievirus B3-induced viral myocarditis.70 In addition to viral myocarditis, a number of lncRNAs emerged as mediators of cardiac inflammation of other origin. The three best-studied lncRNAs are metastasis-associated lung adenocarcinoma transcript (MALAT1), antisense RNA in the INK4 locus (ANRIL), and HOX transcript antisense RNA (HOTAIR). MALAT1 regulates T-cell and macrophage activation.71,72 Knockdown of MALAT1 in a rat model of systemic inflammation protected against cardiac dysfunction in part by decreasing cardiac NF-κB protein levels, and also by decreased circulating TNF-α and IL-6.73 In diabetic mice, the absence of MALAT-1 decreased inflammatory cytokines in the heart.74 However, MALAT-1 does not affect cardiac inflammation in pressure-overloaded hearts, suggesting that the pro-inflammatory property of MALAT1 is dependent on the stimulus.75 LncRNA ANRIL is expressed from the ANRIL locus, a major hotspot for disease-associated mutations (including coronary artery disease).76,In vitro, ANRIL, as for MALAT-1 and ageing-related lncRNA HOTAIR,77 is a pro-inflammatory lncRNA. It is induced by TNF-α via the NF-κB pathway.78 ANRIL itself increases IL-6, the cell adhesion molecules intercellular adhesion molecule-1 (ICAM-1) and vascular cell adhesion molecule-1 (VCAM-1).79 ANRIL, in particular circular ANRIL, induces apoptosis of human ECs,79,80 altogether suggesting a pro-inflammatory role for ANRIL mainly in the vasculature.
6. Non-coding RNAs in the course of left ventricular remodelling and reverse remodelling
Cardiac remodelling is a complex process that introduced molecular, cellular, and interstitial changes leading to changes in size, mass, geometry, and function of the heart in response to pathological stimuli (e.g. high blood pressure, aortic stenosis, MI).81 The literature focusing on the role of ncRNAs in pathological left ventricular (LV) remodelling as well as their therapeutic use capable of preventing involved adverse processes (hypertrophy and fibrosis) is vast and has been adequately reviewed previously.2,6,82–84 However, limited research has been published describing which ncRNAs correlate to the extent of reverse remodelling (RR).
RR refers to any alteration in cardiac disease or HF that can be chronically reversed by a given therapeutic approach (pharmacological or surgical).85 Importantly, it represents a surrogate parameter for patient prognosis.86,87 For instance, severe aortic valve stenosis triggers cardiac remodelling characterized by LV concentric hypertrophy associated with diastolic dysfunction, while aortic valve replacement elicits RR by reducing hypertrophy and improving function. Other examples of RR include the expected recovery of cardiac function and structure after acute MI or in HF patients after cardiac re-synchronization therapy (CRT) or left ventricle assist devices (LVAD) implantation. Incomplete RR is associated with poor prognosis, thus, identification of biomarkers of RR progression or altered signalling pathways to reverse deleterious remodelling stands as a promising target. A recent study by Shah identified a cluster of miRs that provided higher discrimination than a clinical model to predict RR.88
It is tempting to assume that ncRNAs dysregulated in cardiac remodelling are most likely the ones that will normalize during RR. In this context, a study carried out in a rat model of heterotopic transplantation following abdominal aortic constriction, developed to mimic cardiac remodelling and RR, respectively, showed that the expression levels of seven miRs (miR-347, -483, -326, -212, -130 b, -29a, and -23a) were significantly altered in hypertrophic samples but normalized in unloaded heart samples.89 However, and most importantly, these authors indicated a subset of miRs whose expression only changed in unloaded hearts, for example, miR-125a, miR-143, miR-382, and let7 family, suggesting its exclusive role during RR89 (Figure 2). In LVAD patients, Akat et al.90 reported that relative miRNA abundance changes in myocardial tissue could not be detected within the pool of circulating miRNAs but that the former could still serve as excellent biomarkers of heart muscle injury. Nevertheless, miR-208a, miR-208b, and miR-499 in the circulation closely follow its myocardial expression, which is consistent with its cardiac production.90 Interestingly, these same miRs were later shown to serve as novel biomarkers for monitoring and forecasting postoperative myocardial injury and recovery after cardiac surgery in children.91miR-132 is up-regulated during adverse cardiac remodelling and directly contributes to the pathological processes. Importantly, pharmacological blockade of miR-132 to normalize its levels promotes functional recovery and RR in small and large HF animal models.92,93
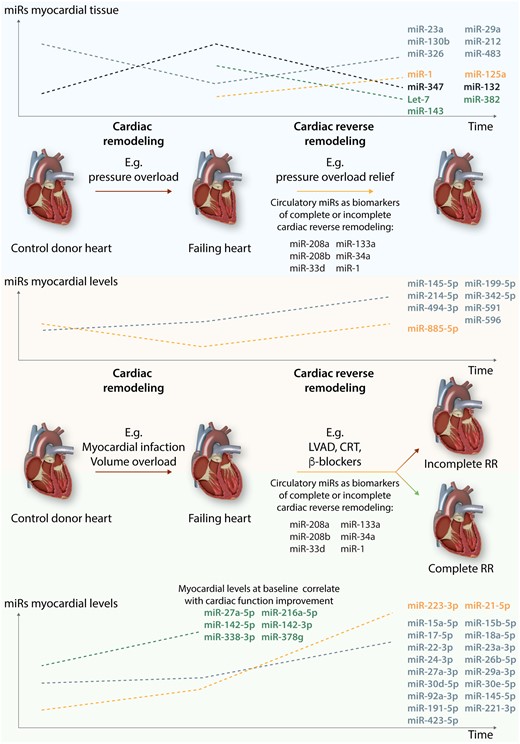
miRs in cardiac (reverse) remodelling. The left side of the figure represents the changes in miRs levels during ventricular remodelling induced by several pathologies, such as aortic valve stenosis, myocardial infarction, and volume overload. The right side depicts changes in miRs levels during reverse remodelling triggered by interventions such as aortic valve replacement, left ventricle assist devices (LVAD) implantation, cardiac re-synchronization therapy (CRT), or treatment with β-blocker. These changes depend on the extent of myocardial reverse remodelling. Myocardial recovery is considered when RR leads to a total normalization of cardiac function and structure.
In CRT patients, the beneficial effects of CRT on RR of HF patients were associated with modulation of circulating miR patterns implicated in cardiac hypertrophy, fibrosis, and apoptosis94 and vary significantly in responders vs. non-responders (patients that do not undergo RR) as depicted in Table 2. In addition, baseline plasma miR-30d levels were shown to be associated with a beneficial RR and to induced CM (iCM) growth and protection against apoptosis in vitro.95
Changes in miRNAs during several types of cardiac reverse remodelling and its relationship with the extent of myocardial recovery
Type of reverse remodelling . | Changes in plasma or myocardial miRNAs levels . | Implications for the extent of myocardial recovery and related signalling pathways . | Ref . |
---|---|---|---|
Left ventricular assist device (LVAD) | ↓ plasma levels of miRs-23a and miR-195 after LVAD | Associated with smaller CM size and a favourable ventricular RR | 100 |
↑ myocardial levels of miR-338-3p, miR-142-5p and -3p, miR-216a-5p, miR-223-3p, miR-27a-5p, and miR-378g | Correlation with off-pump cardiac index values. Predicted targets of these miRs were involved in focal adhesion/integrin pathway and in actin cytoskeleton regulation | 101 | |
↓ miR-29b-3p and miR-374b-5p | Correlation with pulmonary vascular resistance values | 102 | |
↑ myocardial levels of miR-137 | Correlates with down-regulation of α-1-antichymotrypsin mRNA tissue levels in RR | 103 | |
↑ plasma levels of miR-155 | Up-regulated with long-term LVAD support | 104 | |
↑ plasma levels of miR-483-3p and ↓ miR-1202 | Potential capacity to monitor and predict response to LVAD therapy. Low levels of miR-1202 are associated with better response to LVAD | 105 | |
↑ plasma levels of miR-210 at baseline |
| 106 | |
Cardiac reshyncronization therapy (CRT) | ↑ plasma levels of miR-30d at baseline | Associated with a beneficial RR and to induced CM growth and protection against apoptosis in vitro | 94 |
↑ plasma levels of miR-26b-5p, miR-145-5p, miR-92a-3p, miR-30e-5p, and miR-29a-3p | List of miRs that distinguish responders vs. non-responders patients after 1 year of CRT implantation | 107 | |
Aortic valve replacement (AVR) | ↑ levels of miR-21 both in plasma and myocardial tissue | Correlation with mean transvalvular gradient and LV fibrosis | 108 |
↓ plasma levels of miR-1 | Associated with LV hypertrophy (LVH) and correlated with levels of soluble heart-type fatty acid-binding protein-3 (FABP3) | 109 | |
↑ myocardial levels of miR-29, miR-21 | Independent predictors of reverse remodelling and systolic function recovery | 110 | |
↓ plasma levels of miR-206 | Correlated negatively with the left ventricular ejection fraction | 111 | |
↑ plasma levels of miR-133a | Plasma levels reflect its myocardial expression; Positive predictor of the hypertrophy reversibility after surgery | 112 | |
↓ plasma levels of miR-378 at baseline | Predicts LVH independent of the pressure gradient | 113 | |
Drug therapy | ↓ myocardial levels of miR-208a-3p, miR-208b-3p, miR-21-5p, miR-591, and miR-199a-5p and ↑ miR-1-3p 5 months after B-blocker treatment | Differentially expressed accordingly to the degree of RR and predicted the time-dependent RR in response to β-blocker treatment | 97 |
Myocardial infarction remodelling | ↑ plasma levels of miR-1254 | Predicted changes in LV volumes and LVEF at 6 months after STEMI | 114 |
↑ plasma levels of miR-208b and miR-34a | The increase levels were strongly associated with increased risk of mortality or HF within 6 months after acute myocardial infarction | 115 | |
↑ plasma levels of miR-1 and miR-29b | Increase levels correlate with infarct volume accessed by MRI and miR-29b was also associated with left ventricular end-diastolic volumes over time | 116 | |
↑ plasma levels of miR-30a-5p was elevated on admission day | Increased levels at the time of admission are associated development of LV dysfunction and HF symptoms 6 months after acute myocardial infarction | 117 |
Type of reverse remodelling . | Changes in plasma or myocardial miRNAs levels . | Implications for the extent of myocardial recovery and related signalling pathways . | Ref . |
---|---|---|---|
Left ventricular assist device (LVAD) | ↓ plasma levels of miRs-23a and miR-195 after LVAD | Associated with smaller CM size and a favourable ventricular RR | 100 |
↑ myocardial levels of miR-338-3p, miR-142-5p and -3p, miR-216a-5p, miR-223-3p, miR-27a-5p, and miR-378g | Correlation with off-pump cardiac index values. Predicted targets of these miRs were involved in focal adhesion/integrin pathway and in actin cytoskeleton regulation | 101 | |
↓ miR-29b-3p and miR-374b-5p | Correlation with pulmonary vascular resistance values | 102 | |
↑ myocardial levels of miR-137 | Correlates with down-regulation of α-1-antichymotrypsin mRNA tissue levels in RR | 103 | |
↑ plasma levels of miR-155 | Up-regulated with long-term LVAD support | 104 | |
↑ plasma levels of miR-483-3p and ↓ miR-1202 | Potential capacity to monitor and predict response to LVAD therapy. Low levels of miR-1202 are associated with better response to LVAD | 105 | |
↑ plasma levels of miR-210 at baseline |
| 106 | |
Cardiac reshyncronization therapy (CRT) | ↑ plasma levels of miR-30d at baseline | Associated with a beneficial RR and to induced CM growth and protection against apoptosis in vitro | 94 |
↑ plasma levels of miR-26b-5p, miR-145-5p, miR-92a-3p, miR-30e-5p, and miR-29a-3p | List of miRs that distinguish responders vs. non-responders patients after 1 year of CRT implantation | 107 | |
Aortic valve replacement (AVR) | ↑ levels of miR-21 both in plasma and myocardial tissue | Correlation with mean transvalvular gradient and LV fibrosis | 108 |
↓ plasma levels of miR-1 | Associated with LV hypertrophy (LVH) and correlated with levels of soluble heart-type fatty acid-binding protein-3 (FABP3) | 109 | |
↑ myocardial levels of miR-29, miR-21 | Independent predictors of reverse remodelling and systolic function recovery | 110 | |
↓ plasma levels of miR-206 | Correlated negatively with the left ventricular ejection fraction | 111 | |
↑ plasma levels of miR-133a | Plasma levels reflect its myocardial expression; Positive predictor of the hypertrophy reversibility after surgery | 112 | |
↓ plasma levels of miR-378 at baseline | Predicts LVH independent of the pressure gradient | 113 | |
Drug therapy | ↓ myocardial levels of miR-208a-3p, miR-208b-3p, miR-21-5p, miR-591, and miR-199a-5p and ↑ miR-1-3p 5 months after B-blocker treatment | Differentially expressed accordingly to the degree of RR and predicted the time-dependent RR in response to β-blocker treatment | 97 |
Myocardial infarction remodelling | ↑ plasma levels of miR-1254 | Predicted changes in LV volumes and LVEF at 6 months after STEMI | 114 |
↑ plasma levels of miR-208b and miR-34a | The increase levels were strongly associated with increased risk of mortality or HF within 6 months after acute myocardial infarction | 115 | |
↑ plasma levels of miR-1 and miR-29b | Increase levels correlate with infarct volume accessed by MRI and miR-29b was also associated with left ventricular end-diastolic volumes over time | 116 | |
↑ plasma levels of miR-30a-5p was elevated on admission day | Increased levels at the time of admission are associated development of LV dysfunction and HF symptoms 6 months after acute myocardial infarction | 117 |
Changes in miRNAs during several types of cardiac reverse remodelling and its relationship with the extent of myocardial recovery
Type of reverse remodelling . | Changes in plasma or myocardial miRNAs levels . | Implications for the extent of myocardial recovery and related signalling pathways . | Ref . |
---|---|---|---|
Left ventricular assist device (LVAD) | ↓ plasma levels of miRs-23a and miR-195 after LVAD | Associated with smaller CM size and a favourable ventricular RR | 100 |
↑ myocardial levels of miR-338-3p, miR-142-5p and -3p, miR-216a-5p, miR-223-3p, miR-27a-5p, and miR-378g | Correlation with off-pump cardiac index values. Predicted targets of these miRs were involved in focal adhesion/integrin pathway and in actin cytoskeleton regulation | 101 | |
↓ miR-29b-3p and miR-374b-5p | Correlation with pulmonary vascular resistance values | 102 | |
↑ myocardial levels of miR-137 | Correlates with down-regulation of α-1-antichymotrypsin mRNA tissue levels in RR | 103 | |
↑ plasma levels of miR-155 | Up-regulated with long-term LVAD support | 104 | |
↑ plasma levels of miR-483-3p and ↓ miR-1202 | Potential capacity to monitor and predict response to LVAD therapy. Low levels of miR-1202 are associated with better response to LVAD | 105 | |
↑ plasma levels of miR-210 at baseline |
| 106 | |
Cardiac reshyncronization therapy (CRT) | ↑ plasma levels of miR-30d at baseline | Associated with a beneficial RR and to induced CM growth and protection against apoptosis in vitro | 94 |
↑ plasma levels of miR-26b-5p, miR-145-5p, miR-92a-3p, miR-30e-5p, and miR-29a-3p | List of miRs that distinguish responders vs. non-responders patients after 1 year of CRT implantation | 107 | |
Aortic valve replacement (AVR) | ↑ levels of miR-21 both in plasma and myocardial tissue | Correlation with mean transvalvular gradient and LV fibrosis | 108 |
↓ plasma levels of miR-1 | Associated with LV hypertrophy (LVH) and correlated with levels of soluble heart-type fatty acid-binding protein-3 (FABP3) | 109 | |
↑ myocardial levels of miR-29, miR-21 | Independent predictors of reverse remodelling and systolic function recovery | 110 | |
↓ plasma levels of miR-206 | Correlated negatively with the left ventricular ejection fraction | 111 | |
↑ plasma levels of miR-133a | Plasma levels reflect its myocardial expression; Positive predictor of the hypertrophy reversibility after surgery | 112 | |
↓ plasma levels of miR-378 at baseline | Predicts LVH independent of the pressure gradient | 113 | |
Drug therapy | ↓ myocardial levels of miR-208a-3p, miR-208b-3p, miR-21-5p, miR-591, and miR-199a-5p and ↑ miR-1-3p 5 months after B-blocker treatment | Differentially expressed accordingly to the degree of RR and predicted the time-dependent RR in response to β-blocker treatment | 97 |
Myocardial infarction remodelling | ↑ plasma levels of miR-1254 | Predicted changes in LV volumes and LVEF at 6 months after STEMI | 114 |
↑ plasma levels of miR-208b and miR-34a | The increase levels were strongly associated with increased risk of mortality or HF within 6 months after acute myocardial infarction | 115 | |
↑ plasma levels of miR-1 and miR-29b | Increase levels correlate with infarct volume accessed by MRI and miR-29b was also associated with left ventricular end-diastolic volumes over time | 116 | |
↑ plasma levels of miR-30a-5p was elevated on admission day | Increased levels at the time of admission are associated development of LV dysfunction and HF symptoms 6 months after acute myocardial infarction | 117 |
Type of reverse remodelling . | Changes in plasma or myocardial miRNAs levels . | Implications for the extent of myocardial recovery and related signalling pathways . | Ref . |
---|---|---|---|
Left ventricular assist device (LVAD) | ↓ plasma levels of miRs-23a and miR-195 after LVAD | Associated with smaller CM size and a favourable ventricular RR | 100 |
↑ myocardial levels of miR-338-3p, miR-142-5p and -3p, miR-216a-5p, miR-223-3p, miR-27a-5p, and miR-378g | Correlation with off-pump cardiac index values. Predicted targets of these miRs were involved in focal adhesion/integrin pathway and in actin cytoskeleton regulation | 101 | |
↓ miR-29b-3p and miR-374b-5p | Correlation with pulmonary vascular resistance values | 102 | |
↑ myocardial levels of miR-137 | Correlates with down-regulation of α-1-antichymotrypsin mRNA tissue levels in RR | 103 | |
↑ plasma levels of miR-155 | Up-regulated with long-term LVAD support | 104 | |
↑ plasma levels of miR-483-3p and ↓ miR-1202 | Potential capacity to monitor and predict response to LVAD therapy. Low levels of miR-1202 are associated with better response to LVAD | 105 | |
↑ plasma levels of miR-210 at baseline |
| 106 | |
Cardiac reshyncronization therapy (CRT) | ↑ plasma levels of miR-30d at baseline | Associated with a beneficial RR and to induced CM growth and protection against apoptosis in vitro | 94 |
↑ plasma levels of miR-26b-5p, miR-145-5p, miR-92a-3p, miR-30e-5p, and miR-29a-3p | List of miRs that distinguish responders vs. non-responders patients after 1 year of CRT implantation | 107 | |
Aortic valve replacement (AVR) | ↑ levels of miR-21 both in plasma and myocardial tissue | Correlation with mean transvalvular gradient and LV fibrosis | 108 |
↓ plasma levels of miR-1 | Associated with LV hypertrophy (LVH) and correlated with levels of soluble heart-type fatty acid-binding protein-3 (FABP3) | 109 | |
↑ myocardial levels of miR-29, miR-21 | Independent predictors of reverse remodelling and systolic function recovery | 110 | |
↓ plasma levels of miR-206 | Correlated negatively with the left ventricular ejection fraction | 111 | |
↑ plasma levels of miR-133a | Plasma levels reflect its myocardial expression; Positive predictor of the hypertrophy reversibility after surgery | 112 | |
↓ plasma levels of miR-378 at baseline | Predicts LVH independent of the pressure gradient | 113 | |
Drug therapy | ↓ myocardial levels of miR-208a-3p, miR-208b-3p, miR-21-5p, miR-591, and miR-199a-5p and ↑ miR-1-3p 5 months after B-blocker treatment | Differentially expressed accordingly to the degree of RR and predicted the time-dependent RR in response to β-blocker treatment | 97 |
Myocardial infarction remodelling | ↑ plasma levels of miR-1254 | Predicted changes in LV volumes and LVEF at 6 months after STEMI | 114 |
↑ plasma levels of miR-208b and miR-34a | The increase levels were strongly associated with increased risk of mortality or HF within 6 months after acute myocardial infarction | 115 | |
↑ plasma levels of miR-1 and miR-29b | Increase levels correlate with infarct volume accessed by MRI and miR-29b was also associated with left ventricular end-diastolic volumes over time | 116 | |
↑ plasma levels of miR-30a-5p was elevated on admission day | Increased levels at the time of admission are associated development of LV dysfunction and HF symptoms 6 months after acute myocardial infarction | 117 |
In RR induced by aortic valve replacement, regression of hypertrophy 1 year after the intervention correlated to the profile of myocardial gene expression at the time of surgery (e.g. anti-hypertrophic miR-133a, β-myosin heavy chain, myosin light chain-2, and other genes) in conjunction with patients’ clinical background (age, BMI, diabetes mellitus, and male gender). These constitute crucial determinants of RR, which were stronger predictors of cardiac mass reduction than postoperative improvement of valve haemodynamics.96
In idiopathic dilated cardiomyopathy patients, anti-hypertrophic miR-1, miR-199, the profibrotic miR-21-5p, the cardioprotective miR-494-3p, the anti-proliferative miR-591 and miR-208a miRs were differentially expressed according to the degree of RR and predicted the time-dependent RR in response to β-blocker treatment.97
Matkovich has shown that cardiac miR signature is an exquisitely discriminating biomarker of the severely failing heart and of the extent of RR after cardiac unloading, greatly enhancing the predictive ability of miRNA profiles to categorize the clinical status of heart failure.98 Nevertheless, a subsequent comprehensive deep-sequencing analysis highlighted that lncRNAs had the most dynamic expression changes in response to haemodynamic unloading assessed in myocardial biopsies from ischaemic and non-ischaemic patients before and after LVAD implantation. This study revealed that the expression profiles of lncRNAs, but not mRNAs or miRNAs, can discriminate failing hearts of different aetiologies and are markedly altered in response to LVAD support.99 A particularly striking finding here is the high abundance of mRNAs and lncRNAs of mitochondrial origin: 13 mitochondrial mRNAs and 9 mitochondrial lncRNAs alone account for 37% and 71% of the total cardiac mRNA and lncRNA read counts, respectively.99
7. Non-coding RNAs in right ventricular remodelling
Maintenance of normal heart haemodynamics is dependent on right ventricle (RV) function, which is compromised in common diseases such as pulmonary hypertension (PH), congenital diseases (e.g. Tetralogy of Fallot-TOF), and cardiomyopathies (e.g. arrhythmogenic RV cardiomyopathy—ARVC). Despite its importance, the molecular mechanisms underlying RV remodelling in response to stress still remain understudied.
Historically associated with low-pressure pulmonary circulation, RV holds unique features compared to the left ventricle (LV), including thin walls, a crescentic shape, greater compliance to volume overload, and less ability to adapt to higher pressures.118 Disturbances in RV homoeostasis, such as increased afterload, can lead to RV maladaptive remodelling characterized by increased hypertrophy, fibrosis, increased oxygen consumption, a metabolic switch, and alteration of the RV molecular signature including ncRNAs, ultimately leading to RV failure (RVF).118
A variety of miRs have been associated with PH-induced RVF.119miR-223 was found down-regulated in the RV of two rat models of PH, where it targets insulin-like growth factor (IGF) receptor 1 (IGF-IR)/IGF downstream signalling. De-repression of IGF-IR following ablation of miR-223 prevented maladaptive remodelling and improved RV function.120 Another study reported miR-126 as an angiogenic miRNA dysregulated during PH-induced RVF, as its levels drastically decrease in end-stage RVF possibly due to alterations in the angiogenic VEGF/VEGF receptor-2 (VEGFR2)/mitogen-activated protein kinase (MAPK) pathway.121,In vivo administration of miR-126 mimics to a RVF rat model increased expression levels of the target gene sprouty-related EVH1 domain containing 1 (SPRED1), a known negative regulator of the VEGF pathway, and consequently improved RV function.121 miR expression is also dysregulated in TOF and AVRC pathologies, both intrinsically involved in RVF.122,123
More recently, lncRNAs have been linked to RV remodelling and dysfunction. The lncRNA H19 is down-regulated in a rat model of PH-induced RV hypertrophy. The observed protective effect of melatonin treatment in this model, leading to a decrease in RV hypertrophy, was mediated through restored H19 levels and direct suppression of miR-200a by H19.124 A profiling study to identify the lncRNA signature in human RVF samples reported 78 lncRNAs to be differentially expressed when compared to healthy RVs.125 However, to date, no direct association has been reported regarding the functional role of other lncRNAs in RV function.
Thus, the knowledge of ncRNAs on RV remodelling and failure is still limited. Despite promising, studies directly focused on RV circRNAs and lncRNAs remain scarce and more data need to be generated in order to better understand RV remodelling and ultimately develop better therapies.
8. Non-coding RNAs in cardio-oncology
The role of ncRNAs in cardio-oncology is still debated.126 Nevertheless, miRNA-mediated modulation of anthracycline (e.g. doxorubicin)-induced cardiomyopathy has been hypothesized in recent papers.
It has been suggested that the cardiac-specific miR-208a is up-regulated in an experimental model of acute cardiomyopathy induced by anthracyclines.127 The inhibition of miR-208a was able to counteract the deleterious action of anthracyclines on cardiac function and apoptosis. Such inhibition can also de-repress miR-208a-target Gata4 with enhanced expression of the anti-apoptotic gene Bcl2.127miR-532-3p is another miRNA that was enhanced in CMs administered with anthracyclines,128 increasing CM susceptibility to doxorubicin by promoting mitochondrial fission.126 Interestingly, inhibiting miR-532-3p in tumour cells did not impact anthracyclines-mediated apoptosis. Hence, miR-532-3p blockade rescues CM death and mitochondrial fission caused by anthracyclines, with no changes in their anticancer activity.128
Importantly, beside CMs, FBs, SMCs, and cardiac progenitor cells, ECs may also play a major role in doxorubicin-induced cardiomyopathy.129 Acute administration of anthracyclines in murine models reduced microvessel density and VEGF-A expression, while it enhanced miR-320a.130 Inhibiting of miR320a ameliorated heart function, reduced apoptosis, and enhanced microvessel density in mice administered with anthracyclines, while overexpression of miR-320a produced opposite results. Conversely, overexpression of the miR-320a target VEGF-A prevented detrimental effects of miR-320a in cardiac dysfunction induced by anthracyclines, confirming that VEGF is a downstream target molecule.126,130 Moreover, miR-212/132 overexpression was shown to prevent cardiac atrophy in a chronic mouse model of doxorubicin-induced cardiotoxicity.131
Particularly, circulating miRs are intensively investigated as potential blood/plasma-based biomarkers in cardio-oncology.132 For example, circulating miR-1 levels could be enhanced upon anthracyclines administration in breast cancer patients who later developed cardiotoxicity.133 In a paediatric study, children with different tumours exhibited higher levels of circulating miR-29b and miR-499 post-chemotherapy.134 Furthermore, miR-29b and -499 levels were higher in subjects who showed higher hsTnT levels. Oatmen and colleagues performed miRNA profiling in childhood oncology patients and validated several miRNAs (miR-486-3p, -103-3p, -142-3p, and -92a-3p) as potential biomarkers for both acute and chronic anthracycline-induced LV dysfunctions. However, larger patient cohort studies are needed for further validations assessment of the prognostic potential of serum miRNAs.135
In addition, lncRNAs seem to play a role in anthracyclines cardiomyopathy. LncRNA myosin heavy chain-associated RNA transcripts (Mhrt) were found to be down-regulated in doxorubicin-treated hearts.136 Its overexpression in CMs is able to inhibit doxorubicin-induced apoptosis, while its inhibition exacerbates doxorubicin effects. The expression of lncRNA cardiac hypertrophy-related factor (Chrf) was higher in mice hearts after doxorubicin treatment. Chrf inhibition counters apoptosis and transforming growth factor-1 expression induced by anthracyclines. Chrf induction was not observed upon treatment with the angiotensin II receptor blocker valsartan, while adenovirus-mediated overexpression of Chrf reverts the positive effect of valsartan against cardiomyopathy from anthracyclines in rodents.137
Recently, also circRNAs are emerging as mediators of cardiotoxicity. The mitochondrial fission and apoptosis-related circRNA (Mfacr) is up-regulated in a model of anoxia/reoxygenation. Mfacr sponges miR-632-3p which leads to de-repression of Mtp18. Consequently, inhibition of Mfacr increases and decreases miR-632-3p and Mtp18, respectively, resulting in reduced mitochondrial fission and suppressed CM apoptosis.138 Interestingly, the RNA-binding protein Quaking was demonstrated to regulate a number of circRNAs derived from cardiac-specific loci (e.g. Ttn, Fhod3, and Strn3), thereby modulating the susceptibility of CMs to doxorubicin.139
9. Non-coding RNAs in cardiac development and cell specification
The essential role mature miRs play in cardiac development was first revealed by cardiac-specific removal of the miR processing ribonuclease Dicer, which prenatally resulted in defective heart morphogenesis and embryonic lethality.140
Since then, many miRs have been identified that contribute to morphogenesis, for example, miR-1/miR-133,141 to CM proliferation via the miR-15 family,142–144 and thereby mediating congenital heart disease or regeneration.145Muscle-specific myomiRs are involved in essential steps for cardiac development, and therefore, a fine-tuned regulation of these myomiRs, which includes miR-1, -133a, -208a, -208 b, and -499, is essential for the proper embryological development of the heart.146 Interestingly, recent cellular reprogramming and trans-differentiation insights demonstrated that a combined presence of transcription factors Gata4, Mef2c, and Tbx5 (GMT) were able to directly differentiate cardiac FBs into iCMs.147 Subsequently, the same was established by introducing several muscle-specific miRs at the same time, including miR-1, -133, -208, and -499,148 of which miR-133 is suggested to be responsible for further maturing the transdifferentiated iCMs.149 Interestingly, the efficiency of in vivo reprogramming with these approaches via, for example, viral constructs is even more successful than in vitro, leading to more mature CM.150
In addition to miRs, also many lncRNAs were reported to have a cardiac-specific origin during organ development but also in CVD151–154 but, due to their complex regulation, further studies are still needed. The first described key regulator lncRNA in cardiac development was Braveheart.155 Braveheart was detected in cardiac mesoderm and CMs, and involved to move cells from nascent to cardiac mesoderm and further towards full CM differentiation. Another lncRNA example was named TERMINATOR and is essential for maintaining of pluripotency in stem cells and the subsequent early mesodermal differentiation and survival. Interestingly, mesodermal specification followed by cardiac chamber formation is mediated via ALIEN, which is mainly expressed in cardiovascular progenitor cells.156 In addition, the CM regeneration-related lncRNA (CRRL) was identified to be involved in CM proliferation and regeneration, as indicated by enhanced positivity of Ki-67/pH3/EdU in CMs.157
More recently, transcriptional regulation of circRNAs was reported during rodent and human heart development and disease.158 The myocardium displays a general increased expression over-time of circRNAs, especially during the first weeks of the second trimester of human foetal development.159 Interestingly, very recently, circRNAs from the titin gene were dysregulated upon the removal of the splicing regulator RNA-binding motif protein 20 (RBM20).160 Nevertheless, the functional consequences of cardiac circRNA dysregulation still awaits further investigation.
10. Regulation of CM proliferation and cardiac regeneration by ncRNA
As any aspect of cardiac biology, CM proliferation is also under the control of the ncRNA network. Multiple miR and a few lncRNAs identified to date are known to either stimulate or inhibit CM proliferation.
Starting from a systematic screening of a library of ∼1000 human miRs,143 several miRs have been reported to stimulate CM proliferation in mice, rats, pigs, and human cells, as well as to induce cardiac regeneration after MI.100,143,161,162 Several of the pro-proliferative miRs belong to a few miR families, including members of the miR-302/367 cluster and the miR-290 family in mice. These miRNAs share a similar seed sequence and are highly expressed during the early stages of development. In particular, they are involved in the specification and maintenance of pluripotency of embryonic stem (ES) cells,163 in which the miR-290 cluster alone accounts for 70% of the entire miR content.164
Other miR families are known to be crucial in the regulation of cell proliferation in other cell types also induce CM replication. Expression of the miR-17-92 cluster165 is activated in several human tumours, hence the name OncomiR1.166,167 Transgenic overexpression of this miR cluster in CMs168 or cardiac delivery of two members of the family, miR-19a and miR-19b,169 induce CM proliferation in both pre- and postnatal hearts, and stimulate cardiac regeneration after MI.
Withdrawal of CMs from the cell cycle also depends on increased levels of the miR-15 family144 and of miR-29a.170 Inhibition of these miRs, which target various components of the cell cycle and DNA damage response machinery, leads to improved cardiac repair after MI.171
A common characteristic of most of the pro-proliferative miRs is the activation of the Yap transcriptional co-factor, originally discovered as the final positive effector of the otherwise inhibitory Hippo pathway in Drosophila.172,173 Indeed, a high-throughput screening performed on human-induced pluripotent stem cell (hiPSC)-derived CMs revealed that most of the miRs that increase CM proliferation converge on the Hippo pathway.174
Not surprisingly, CM proliferation is also under the control of various lncRNAs.175 In several instances, these inhibit CM proliferation by acting as sponges for pro-proliferative miRNAs. This is the case of CAREL (a sponge for miR-296; Ref.176), CRRL (for miR-199a-3p; Ref.157), and AZIN2-sc (for miR-214; Ref.50). In other cases, the sponge effect is for inhibitory miRNAs (such NR_045363 for miR-216a; Ref.177), with the lncRNA then exerting a positive effect on CM proliferation. Other lncRNAs that increase CM proliferation are ECRAR, which binds to and promotes phosphorylation of ERK1/2178 and Sirt1 antisense lncRNA, which stabilizes the Sirt1 mRNA.179 The CPR lncRNA instead blocks CM proliferation by recruiting DNMT3A to the promoter region of the MCM3 gene.180 While most of these lncRNAs or their inhibitors exert a positive effect after MI in rodents, the entity of this effect appears more modest compared to that exerted by miRs, likely due to pleiotropic functions of the latter molecules. It is possible that more effective lncRNAs regulating proliferation of CMs or cardiac progenitor cells will be identified in the class of enhancer-associated lncRNAs.175
11. Non-coding RNA in cardiovascular aging
Aging is the main risk factor for CVD. All cells and organs relevant for the cardiovascular system are affected by aging. Not surprisingly ncRNAs seem to also play important roles in cardiac aging, and this knowledge is derived mainly from mouse studies.
Analysis of young and old mouse hearts revealed differential expression of 65 miRNAs,181 including changes in the expression of three miR clusters—miR-17-92, -106a-363, and -106 b-25—that potentially target the Cdc42-SRF signalling pathway.182 A longitudinal study in mice from birth to 19 months of age found that miR-22 was prominently up-regulated during cardiac aging and appeared to contribute at least partly to accelerated cardiac FB senescence and migratory activity during aging.183 The most notable examples in ECs are miR-34 and miR-92. miR-34 was first identified as a P53-responsive SIRT1-targeting miR.184 SIRT1 is known to counteract aging and to induce EC function.185 Later it was shown that miR-34 contributes to endothelial aging by inhibiting SIRT1.186 Several other targets for miR-34 have been identified. PNUTS, the most notable aging-regulated miR-34 target, was shown to be even more important for miR-34-induced cardiac aging than SIRT1, although the role of PNUTS in endothelial aging is currently unknown.187 The involvement of miR-92 in vascular aging was demonstrated in 2017 by two groups.188,189 Mechanistically, endothelial miR-92 levels rise with aging, resulting in loss of the antioxidant transcriptional network orchestrated by NRF2. Moreover, expression and functional studies of the miR-17-92 cluster also support a role in CM aging through the up-regulation of connective tissue growth factor and thrombospondin-1.190miR-17, another miR-17-92 cluster member, is a senescence-related miRNA that inhibits mouse cardiac FB senescence by targeting Par4.191
In recent years, several lncRNAs have been linked to cardiovascular aging, the most prominent examples being Meg3, H19, MIAT, and HOTAIR. Meg3 was identified as an aging-induced lncRNA in ECs that inhibits angiogenesis.192 Inhibition of Meg3 in aged mice restored angiogenic function. Importantly, Meg3 is also highly expressed in cardiac FBs, and silencing Meg3 in CFs prevents the induction of MMP-2, thus leading to decreased cardiac fibrosis and improved diastolic performance in a mouse model of pressure overload-induced HF. LncRNA H19 that is repressed during aging, was found to regulate EC and SMC function. In ECs, the decline of H19 contributes to senescence and pro-inflammatory signalling,193 whereas in SMCs, H19 induction is causally related to aneurysm formation.194 MIAT is named myocardial infarction-associated transcript because it was first discovered to be genetically associated with (cardio)vascular disease. MIAT is repressed in senescent FBs195 and controls microvascular function.196 HOTAIR, on the other hand, is induced in senescent FBs. Several mechanisms for HOTAIR function have been proposed, depending on the cell type and disease context. For example, silencing HOTAIR inhibits senescence by regulating protein ubiquitination.77
Little is known about the involvement of circRNAs in cardiac aging. One example is the circRNA circ-Foxo3 which is up-regulated in heart samples of aged patients and mice. Mechanistic studies suggest circ-Foxo3-dependent induction of cell senescence, possibly via cytoplasmic retention of multiple anti-senescence and anti-stress factors (ID-1, E2F1, HIF1a, and FAK).19
11.1 Outlook and therapeutic perspective of ncRNA
As shown in the overview, there are many new aspects in the mechanistic view of how ncRNAs are able to control cellular functions of cardiovascular cells. We here focused on actual topics actively investigated within working groups of the ESC but also beyond ranging from cellular communication to regeneration to aging aspects. For instance, the discovery that CM proliferation is under the control of the miR network prompted the development of therapeutic strategies that take advantage of these molecules. Blocking the activity of endogenous, inhibitory miRNAs is now possible through the delivery of either antisense oligonucleotides containing locked nucleic acid (LNA)-modified nucleotides,197 often with a GapmeR design,198 or adeno-associated virus (AAV) vectors expressing antisense sequences. LNAs against miR-15171 or miR-34a199 and AAV vectors expressing anti-let-7 sequences200,201 have all been shown to induce cardiac regeneration after MI in rodents.
Another approach is to boost the minimal regenerative capacity of the heart by the delivery of miRNA mimics, independent from their endogenous, normal expression in CMs. Delivery of both miR-199a and miR-590 in mice resulted in remarkable formation of new cardiac mass after MI, with the consequent restoration of cardiac function.143 Analogous findings were reported for AAV vectors expressing miR-294162 and miR-19a/19b.169
The long-term expression of miRNAs inducing proliferation, as it occurs upon AAV-mediated gene transfer or in transgenic animals, however, can be detrimental, particularly because CM replication is accompanied by their de-differentiation. In addition, the pri-miRNA gene cloned inside AAV vectors results in the production of both miRNA strands, with possible unwanted side effects. Consistent with these concerns, infarcted pigs treated with an AAV-miR-199a vector showed remarkable regeneration at 1 month after treatment, however developed fatal arrhythmias at later times.202 Transgenic mice overexpressing the miR-302/367 cluster also developed cardiac dysfunction due to CM de-differentiation and hyperproliferation.161
These problems may be overcome by the transient delivery of synthetic miRNA mimics. The intracardiac injection of miR-199a-3p, miR-590-3p,203 or miR-19a/19b mimics,169 using different lipids, or of cholesterol-modified miR-302b/c mimics using a hydrogel,100 all led to the persistence of the miRNAs for several days after administration and stimulated cardiac repair. A regenerative effect after MI was also achieved by the daily intravenous administration of lipid formulations delivering miR302b/c,161 miR-19a/19b,169 and miR-708204 mimics.
While proved very effective in pre-clinical models already, several obstacles such as targeted delivery, off-target effects, and hepatic/renal toxicity remain to be overcome for broad clinical application of ncRNA therapeutics. For instance, the use of viral vectors based on naturally occurring serotypes of adeno-associated viruses is strongly limited by the presence of neutralizing antibodies in up to 70% of the population.205 Another example is the use of synthetic miR mimics or antisense-oligonucleotides. While systemic or local administration of such ncRNA therapeutic may be beneficial in the heart or a specific cardiac cell type, it may cause deterioration in other organs such as kidney or liver or in non-target cardiac cell types, respectively. Thus, further efforts are urgently needed allowing for precise spatiotemporal delivery of ncRNA therapeutics.
However, a promising translational example is the clinical development of anti-miR-132 molecules in patients with heart failure. Demonstrating strong efficacy of miR-132 inhibition to treat post-MI heart failure in pigs,93 a clinical study was launched to test the safety of CDR132L, a synthetic miR-132 blocker, directly in heart failure patients (www.clinicaltrial.gov; NCT04045405).
Collectively, basic, translational, and clinical ncRNA research conducted by members of the ESC Working Groups of Myocardial Function and Cellular Biology of the Heart helped to pave the way for future ncRNA-based therapies. Therapeutics targeting ncRNAs have now entered the clinical setting for the treatment of CVD. We strive to continue leading the ncRNA therapeutic field and we are following new developments with great passion and excitement.
Data availability
The data underlying this article are available in the article itself.
Conflict of interest: C.B. reports grants from the DFG and the BMBF, during the conduct of the study; in addition, C.B. has a patent Non-coding RNA-based Therapy in CVD pending. R.A.B. has several issued patents on ncRNAs. L.d.W. is founder and stockholder of Mirabilis Therapeutics BV, outside the submitted work. S.H. reports personal fees from CellProthera, personal fees from Galapagos, grants from Dutch Heart Foundation, grants from Horizon 2020, FP7 project EU, outside the submitted work; R.F.V. reports grants from Foundation for Science and Technology of Portugal (FCT), during the conduct of the study. C.G.T. reports grants from Federico II University, outside the submitted work. M.G. has several issued patents in miRNAs. S.Z. has a patent on microRNAs for cardiac regeneration issued to ICGEB, and a miRNA patent pending to ICGEB. S.E. has a licensed patent on a miRNA and received grants in the field of ncRNA fromthe DFG, the BMBF and the EU. S.D. reports grants from the DFG, the BMBF and the EU, licencing fees from miRagen, grants from Servier, during the conduct of the study; In addition, S.D. has a miRNA patent licensed to t2cure/Miragen, a has several further miRNA patents issued. S.D. is developing antimiR therapeutics and as such as a conflict of interest. T.T. reports grants from the DFG, the BMBF and the EU, during the conduct of the study; personal fees and other from Cardior Pharmaceuticals, outside the submitted work; In addition, T.T. has patents on noncoding RNAs with royalties (Regulus Therapeutics, Cardior) and is on an Advisory Board (Novo Nordisk) (outside the submitted work). All other authors have nothing to declare.
Funding
T.T. and C.B. were supported by Deutsche Forschungsgemeinschaft, DFG (TRR267) and the ERANet CVD (JTC2016 project EXPERT to T.T. and JTC2018 project INNOVATION to C.B.). C.G.T. was supported by a ‘Federico II University/Ricerca di Ateneo’ grant. I.F.P. is supported by Fundo Europeu de Desenvolvimento Regional (FEDER) through Compete 2020—Programa Operacional Competitividade E Internacionalização (POCI), the project NETDIAMOND (POCI-01-0145-FEDER-016385), supported by European Structural and Investment Funds, Lisbon’s regional operational programme 2020. P.R. is funded by FCT (SFRH/BD/96026/2013). R.M. was supported by a Cardio-Oncology grants from Incyte s.r.l. and funds from Ministero dell’Istruzione, Università e Ricerca Scientifica (549901_2020_Madonna: Ateneo). N.H. was supported by Deutsche Forschungsgemeinschaft, DFG (HA7512/2-1). J.P.G.S. was supported by the Project EVICARE (No. 725229) of the European Research Council (ERC). V.A.’s laboratory received support from the Instituto de Salud Carlos III (ISCIII) (grant AC16/00091, as member of the ERA-CVD JCT2016 EXPERT Network, European Union’s Horizon 2020 Framework Programme), with co-funding from the European Regional Development Fund (ERDF/FEDER, ‘Una manera de hacer Europa’). R.M.N. is supported by the Ministerio de Educación, Cultura y Deporte (predoctoral contract FPU16/05027). The CNIC is supported by the Spanish Ministerio de Ciencia e Innovación (MCI), the ISCIII, and the Pro CNIC Foundation, and is a Severo Ochoa Center of Excellence (SEV-2015-0505).