-
PDF
- Split View
-
Views
-
Cite
Cite
Daniel J Spade, Susan J Hall, Jeremy D Wortzel, Gerardo Reyes, Kim Boekelheide, All-trans Retinoic Acid Disrupts Development in Ex Vivo Cultured Fetal Rat Testes. II: Modulation of Mono-(2-ethylhexyl) Phthalate Toxicity, Toxicological Sciences, Volume 168, Issue 1, March 2019, Pages 149–159, https://doi.org/10.1093/toxsci/kfy283
- Share Icon Share
Abstract
Humans are universally exposed to low levels of phthalate esters (phthalates), which are used to plasticize polyvinyl chloride. Phthalates exert adverse effects on the development of seminiferous cords in the fetal testis through unknown toxicity pathways. To investigate the hypothesis that phthalates alter seminiferous cord development by disrupting retinoic acid (RA) signaling in the fetal testis, gestational day 15 fetal rat testes were exposed for 1–3 days to 10−6 M all-trans retinoic acid (ATRA) alone or in combination with 10−6–10−4 M mono-(2-ethylhexyl) phthalate (MEHP) in ex vivo culture. As previously reported, exogenous ATRA reduced seminiferous cord number. This effect was attenuated in a concentration-dependent fashion by MEHP co-exposure. ATRA and MEHP-exposed testes were depleted of DDX4-positive germ cells but not Sertoli cells. MEHP alone enhanced the expression of the RA receptor target Rbp1 and the ovary development-associated genes Wnt4 and Nr0b1, and suppressed expression of the Leydig cell marker, Star, and the germ cell markers, Ddx4 and Pou5f1. In co-exposures, MEHP predominantly enhanced the gene expression effects of ATRA, but the Wnt4 and Nr0b1 concentration-responses were nonlinear. Similarly, ATRA increased the number of cells expressing the granulosa cell marker FOXL2 in testis cultures, but this induction was attenuated by addition of MEHP. These results indicate that MEHP can both enhance and inhibit actions of ATRA during fetal testis development and provide evidence that RA signaling is a target for phthalate toxicity in the fetal testis.
Phthalate esters (phthalates), including di-(2-ethylhexyl) phthalate (DEHP), are male reproductive toxicants used in industrial processes and consumer goods, especially to plasticize polyvinyl chloride, but also in products such as solvents, adhesives, and personal care products. Human exposure to phthalates is ubiquitous (Kavlock et al., 2002, 2006), and phthalate exposure during gestation has been linked to male reproductive tract defects and decreased fertility in later life (Fisher, 2004; Fisher et al., 2003). Phthalates are well-characterized as anti-androgens that reduce fetal testicular testosterone in the rat (Furr et al., 2014). However, phthalates alter fetal seminiferous cord development even in the absence of detectable anti-androgenic effects (Gaido et al., 2007; Habert et al., 2014a,b; Heger et al., 2012; Johnson et al., 2012; Mitchell et al., 2012; Spade et al., 2014). It is therefore likely that phthalates have multiple targets in the fetal testis, including a molecular initiating event unrelated to androgen signaling that triggers the adverse effects of phthalates on Sertoli and/or germ cells.
A challenge for describing the mechanisms of phthalate toxicity has been identification of molecular initiating events (Howdeshell et al., 2015). The mechanism of the phthalate-induced anti-androgenic action in the rat fetal testis is reduction of testosterone, without antagonism of the androgen receptor (Foster et al., 2001; Parks et al., 2000). Phthalates interact with peroxisome proliferator-activated receptors (PPARs), but there is little evidence that activation of canonical PPAR targets is a major component of the fetal testis toxicity of phthalates (Hannas et al., 2012; Howdeshell et al., 2015; Rouiller-Fabre et al., 2015). Another possibility is that phthalate toxicity is mediated by disruption of signaling through other nuclear receptors, potentially mediated by crosstalk with PPARs. For example, in mouse Sertoli cells, exposure to mono-(2-ethylhexyl) phthalate (MEHP) or other peroxisome proliferators prevents translocation of retinoic acid receptors (RARs) into the nucleus, presumably because of competition between PPARs and RARs for access to retinoid X receptors (RXRs), with which both receptors form heterodimers (Bhattacharya et al., 2005; Dufour et al., 2003). Therefore, interaction between phthalates and the retinoic acid (RA) signaling pathway is a potential mechanism of phthalate toxicity in the fetal testis.
RA signaling is critical for gonad development. During fetal development, the testis is protected from high levels of RA by expression of the RA-metabolizing CYP26B1 enzyme, in contrast to the ovary, where RA is present at concentrations that promote ovary development and initiation of germ cell meiosis (Bowles et al., 2006; Li et al., 2009). Cyp26b1 levels in the testis are directly under the control of the pro-testis developmental signaling factors Sry and Sox9 (Kashimada et al., 2011; Li et al., 2014), indicating that metabolism of RA is required for testis development. Following RA exposure in vitro, or in systems in which CYP26B1 is knocked out or inhibited in vivo, fetal testis development is disrupted, resulting in decreased Leydig cell function, inappropriate meiotic entry in germ cells, and loss of seminiferous cord structure, with some variation by species (Cupp et al., 1999; Hogarth et al., 2015; Jorgensen et al., 2015; Lambrot et al., 2006; Livera et al., 2000, 2001, 2004; Trautmann et al., 2008). The disorganized testicular phenotype caused by all-trans retinoic acid (ATRA) exposure ex vivo is associated with enhanced expression of the ovarian development gene, Wnt4, and the anti-testis transcription factor, Nr0b1, as well as an increase in the number of FOXL2-positive Sertoli cells, indicating aberrant induction of signaling for ovary developmental processes in the testis (Spade et al., 2018). Because phthalates can interact with the RA signaling pathway, which influences germ cell and seminiferous cord development, and because phthalates have effects on germ cells and seminiferous cords that are not secondary to their anti-androgenic effects, we hypothesized that exposure to MEHP would alter RA signaling and expression of sex determination factors in the rat fetal testis. To test this hypothesis, rat fetal testes were cultured for 1–3 days with ATRA, MEHP, or co-exposure to both ATRA and MEHP, and cultured testes were analyzed for morphological changes and changes in expression of key genes and proteins involved in RA signaling and gonadal cell fate maintenance.
MATERIALS AND METHODS
Animals
All procedures involving animals were approved by the Brown University Institutional Animal Care and Use Committee, in accordance with the Guide for the Care and Use of Laboratory Animals. Timed pregnant SAS Sprague Dawley rats (strain code 400) were obtained from Charles River and euthanized on gestational day (GD) 15 by inhalation of isoflurane. Fetuses were euthanized by decapitation, and sex was determined by examination of gonads under a stereomicroscope.
Testis cultures
GD 15 fetal rat testes were cultured as reported in Spade et al. (2018). Briefly, testes were placed on Millicell-CM cell culture inserts in 24-well cell culture plates with 460 µl DMEM/F12 media supplemented with 20 µg/ml gentamicin, 50 units/ml penicillin, 50 µg/ml streptomycin, 50 µg/ml Albumax II, 200 µg/ml bovine serum albumin, 1× ITS liquid media supplement, and 50 µg/ml sodium l-ascorbate. Testes were exposed in culture media to vehicle control (1:4000 DMSO), MEHP (10−6, 10−5, or 10−4 M), 10−6 M ATRA alone, or 10−6 M ATRA with MEHP (10−6, 10−5, or 10−4 M; referred to hereafter as co-exposure). The testes of each male fetus were divided so that one was cultured in treatment media and the other in vehicle. Sample size for each analysis was 6–7 testis pairs per treatment group, except for FOXL2 IHC, where sample size was 3 testis pairs per treatment group. ATRA and MEHP stock solutions were prepared in DMSO, aliquoted in light-protected vials, and stored at −20°C for up to 14 days before use. Media was prepared daily and changed every 24 h. Stocks and media were protected from excessive exposure to fluorescent light by handling in light-protected vials under low light and/or a yellow lamp. Following 24 h of culture, samples intended for RNA isolation were collected by snap freezing in liquid nitrogen and were stored at −80°C. On day 3 of culture, the remaining samples were collected for histology by fixing in modified Davidson’s solution for 15 min, then storing in 70% ethanol at 4°C until processing. MEHP was chosen for this experiment as a model of the testicular toxicity of phthalates. MEHP and its diester parent compound, di-(2-ethylheyxl) phthalate (DEHP) are anti-androgenic in rats and induce multinucleated germ cells (MNGs) in rats, mice, and humans, according to a large body of literature (Andrade et al., 2006; Chauvigne et al., 2009,, 2011; Do et al., 2012; Dostal et al., 1988; Doyle et al., 2013; Gaido et al., 2007; Hannas et al., 2011; Howdeshell et al., 2007; Muczynski et al., 2012; Parks et al., 2000; Wang et al., 2016). MEHP falls within the 4–6 carbon parent side chain length range that has been determined to have the greatest effect on testosterone levels in rats (Furr et al., 2014). Phthalate monoesters are considered to be the dominant and toxicologically active phthalate metabolites in vivo (Albro, 1986; Calafat et al., 2006; Clewell et al., 2010; Jones et al., 1993; Saillenfait et al., 2001), making MEHP the appropriate compound for an in vitro experiment.
Histology and immunohistochemistry
For histology and immunohistochemistry analyses on 3-day testis culture samples, briefly, fixed testes were dehydrated in a series of graded ethanols, followed by xylenes, and embedded in paraffin. About 5 µm sections were cut from each paraffin-embedded sample at approximately 150, 300, 450, and 600 µm depths. Cut sections were deparaffinized in xylene and rehydrated through a series of graded ethanols. Sections were stained with hematoxylin and eosin (H&E) for analysis of general histology and counting of seminiferous cord number on an Olympus BH-2 light microscope. Seminiferous cord number per section was quantified for 6–7 testis pairs per treatment group. Sections were also examined for depletion of germ cells and induction of MNGs. Immunohistochemical staining was performed for actin, alpha 2, smooth muscle, aorta (ACTA2, also called smooth muscle α-actin) and the ovarian protein, forkhead box L2 (FOXL2), using staining methods and primary and secondary antibodies and concentrations reported in Spade et al. (2018). The IHC labeling protocol for the germ cell marker and VASA homolog, DEAD-box helicase 4 (DDX4) was modified as follows: citrate buffer antigen retrieval and permeabilization steps were omitted; blocking was performed for 60 min in PBS with 8% normal goat serum and 1% BSA; primary and secondary antibody concentrations were both 1:4000. All histological and immunohistochemical images were obtained from slides scanned at 40× magnification on an Aperio ScanScope CS (Aperio Digital Pathology/Leica Biosystems, Buffalo Grove, Illinois). FOXL2-positive cells were counted manually on digital slide images of 1–4 sections per block in Aperio ImageScope software by a scorer who was blind to treatment. The average value for each block was used for statistical analysis (n = 3 testis pairs per group). Cleaved caspase-3 IHC labeling is described in the Supplementary Methods and Supplementary Table 1.
Real-time RT-PCR
For RNA extraction, snap-frozen tissue samples were homogenized by shaking with approximately 100–200 mg stainless steel beads (Next Advance, Troy, New York) in a Disruptor Genie (Scientific Industries, Bohemia, New York) for 2 min at the highest setting. RNA was isolated and purified using the Qiagen DNA/RNA AllPrep kit, according to the manufacturer’s protocol, including the optional on-column DNase digestion. cDNA was prepared from 150 ng total RNA from each sample, using the Qiagen RT2 First Strand Kit (Qiagen, Valencia, California). cDNA produced from 1.35 ng input RNA was used in each real-time PCR reaction well. Primers for real-time PCR were RT2 QPCR Primer Assays, pre-validated primer sets obtained from SA Biosciences/Qiagen (Supplementary Table 2), and reactions were run with RT2 Real-Time SYBR Green/ROX PCR Master mix (Qiagen). PCR amplification and quantification was performed on a ViiA 7 Real-Time PCR System (Applied Biosystems, Foster City, California). Target genes selected for known responsiveness to treatment with ATRA or MEHP included the RAR target gene Rbp1, ovary development-related genes Wnt4 and Nr0b1, germ cell marker Ddx4, germ cell pluripotency marker Pou5f1, and the Leydig cell marker/steroidogenesis gene Star. Housekeeping genes were Ldha and B2m.
Each primer assay was first tested as described in Spade et al. (2018): the PCR reaction was run with a test pool of rat fetal testis cDNA, products were purified using the QIAquick PCR Purification Kit (Qiagen), then visualized on an agarose gel to confirm amplicon size and specificity. The concentration of purified PCR products was determined using a NanoDrop ND-1000 spectrophotometer, and purified products were serially diluted to create a 7-point standard curve with 108 through 102 copies of PCR product per reaction. RNA from randomly selected samples was pooled and used as template to create a no reverse transcriptase negative control. All real-time PCR samples and standards were run in triplicate. The real-time PCR reaction included an initial denaturation at 95°C for 15 min, 40 cycles of a 2-step PCR reaction (95°C for 15 sec, 60°C for 1 min), and a denaturation curve analysis. A standard curve was run on each plate, and each plate included no template (water) and no reverse transcriptase negative controls. The SA Biosciences rat genomic DNA negative control primer set was also run to confirm the absence of genomic DNA contamination in all samples. Real-time PCR analyses were based on 6–7 testis pairs per treatment group. Experimental sample concentration (copies/µl) was determined in QuantStudio Real-Time PCR Software v1.3 (Applied Biosystems) by comparison to the standard curve. Target gene concentration was normalized to the geometric mean of concentration for the housekeeping genes, and fold change was determined for each sample relative to the mean of the normalized vehicle control values.
Testosterone analysis
Media samples from the third day of culture with 10−6 M ATRA, 10−4 M MEHP, 10−6 M ATRA + 10−4 M MEHP, and paired vehicle samples were sent to the Ligand Assay and Analysis Core at the University of Virginia for testosterone analysis. Samples were diluted 1:10 or 1:20 to achieve a concentration within the reportable range of 10–1600 ng/dl and tested in duplicate using the mouse and rat testosterone ELISA from Immuno-Biological Laboratories. The intra-assay and inter-assay % CV reported by the Ligand Assay and Analysis Core for this assay in 2017 were 5.4 and 7.8, respectively.
Data analysis and statistics
Statistical analysis was performed using GraphPad Prism 7 (GraphPad Software, San Diego, California). All vehicle samples were treated as a single group, and differences between treatment groups were assessed using 1-way ANOVA, followed by Tukey’s test for multiple comparisons, for most analyses. Testosterone data were analyzed by 2-way ANOVA followed by Tukey’s test. Data are reported in figures as mean ± SEM.
RESULTS
MEHP Altered Seminiferous Cord Number and Testicular Gene Expression
Following 3-day ex vivo exposure to MEHP, the seminiferous cord structure of cultured fetal testes remained intact. There was no consistent effect of MEHP on seminiferous cord number; however, the mean cord number was slightly higher in all MEHP-treated groups than vehicle, and this was statistically significant at 10−5 M MEHP. ACTA2 was expressed around the basement membranes of seminiferous cords and in the periphery of the section, but a few abnormal patches of ACTA2 expression were present in the interstitium, and broken patterns of ACTA2 expression were present around poorly defined cords (Figure 1).
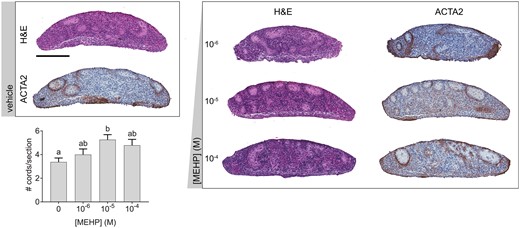
MEHP alone increased or had no effect on seminiferous cord number. Representative images of cultured fetal testes exposed to a concentration range of MEHP for 3 days, stained with hematoxylin and eosin (H&E) or immunohistochemically labeled for smooth muscle alpha actin (ACTA2). Scale bar = 200 µm. The impact of MEHP on the number of seminiferous cords per section was not concentration dependent, but 10−5 M MEHP significantly increased cord number versus vehicle. Many cords in MEHP-treated testes had irregular morphology. Values reported in chart are mean ± SEM. n = 17 testes in vehicle control group and 6–7 testes per treated group. Bars not connected by the same letter are significantly different (p < .05) by 1-way ANOVA followed by Tukey’s post hoc test. Overall ANOVA p = 0.0159. The full-color image is available online.
Real-time RT-PCR targets were selected based on evidence of response to ATRA (Spade et al., 2018) or known responsiveness to phthalates in Leydig and germ cells. Following 1 day of MEHP exposure (Figure 2), expression of the retinol binding protein and known RA signaling target, Rbp1, was induced in a concentration-dependent manner. The expression levels of Wnt4 and Nr0b1, involved in gonadal sex determination, were also elevated in an apparently concentration-dependent manner, with statistical significance at the highest MEHP dose. The Leydig cell-expressed steroidogenesis marker Star, and the germ cell-expressed genes, Pou5f1 and Ddx4, were decreased in a concentration-dependent manner by MEHP.
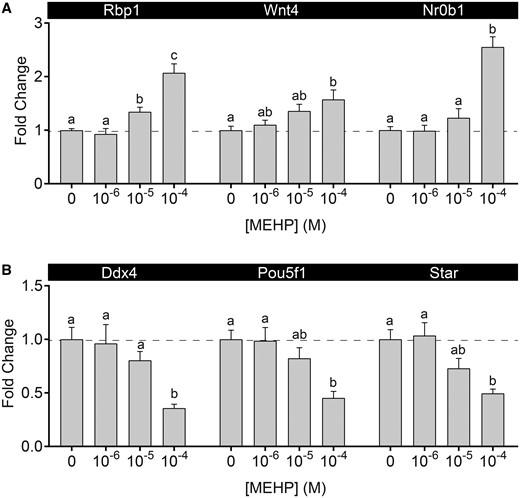
MEHP altered testicular gene expression. A, MEHP exposure led to a concentration-dependent increase in Rbp1, Wnt4, and Nr0b1 expression. B, MEHP exposure led to a concentration-dependent decrease in Ddx4, Pou5f1, and Star. Values are mean ± SEM. Dashed line at y = 1. n = 19 testes in vehicle control group and 6–7 testes per treated group. Within each gene, bars not connected by the same letter are significantly different (p < .05) by 1-way ANOVA followed by Tukey’s post hoc test.
MEHP-Opposed ATRA Effect on Cord Structure and Enhanced ATRA-Driven Gene Expression
Following MEHP/ATRA co-exposure, ATRA reduced seminiferous cord number per section, as previously reported. ATRA-treated cultures showed ACTA2 expression exclusively in the periphery of the sample. However, MEHP attenuated the seminiferous cord loss in a concentration-dependent manner. At the highest MEHP co-exposure concentration (10−4 M), cord number was significantly greater than ATRA alone, and there was no significant difference between co-exposure and vehicle-treated cultures. MEHP co-exposure also restored the ACTA2-expressing cells, presumably peritubular myoid cells, surrounding the basement membrane region of seminiferous cords (Figure 3).
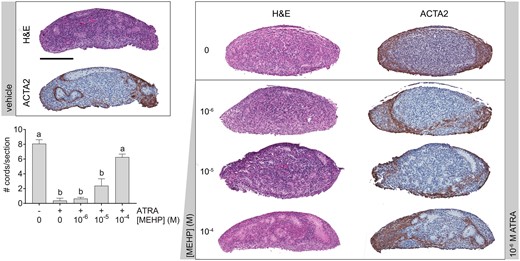
MEHP opposed the seminiferous cord disrupting effect of ATRA. Representative images of cultured fetal testes exposed to a concentration range of MEHP with or without 10−6 M ATRA for 3 days, labeled with hematoxylin and eosin, or immunohistochemically labeled for smooth muscle alpha actin (ACTA2). 10−6 M ATRA caused seminiferous cord disorganization and loss of cord structure, but this effect was opposed by MEHP. ACTA2 was expressed only at the periphery of the ATRA-treated testes. MEHP co-exposure prevented the loss of ACTA2 deposition at seminiferous cord basement membranes that was observed in ATRA-treated cultures Scale bar = 200 µm. ATRA decreased the number of identifiable seminiferous cords per section, but cord number was increased in a concentration-dependent manner by MEHP. On x-axis, “−” indicates no ATRA; “+” indicates 10−6 M ATRA. Values reported in chart are mean ± SEM. n = 24 testes in vehicle control group and 6–7 testes per treated group. Bars not connected by the same letter are significantly different by 1-way ANOVA followed by Tukey’s post hoc test. Overall ANOVA p < .0001. The full-color image is available online.
Following 1 day of culture, MEHP/ATRA co-exposure samples provided evidence of interaction between ATRA and MEHP on gene expression (Figure 4), with the presence of MEHP predominantly enhancing the gene expression effects of ATRA. MEHP decreased expression of Star, Pou5f1, and Ddx4, and this was exacerbated in degree and significance by ATRA. The increased expression of Rbp1 appeared to be enhanced by the combination of MEHP and ATRA; however, this effect appeared to plateau at approximately 6-fold induction of Rbp1 and was not significantly different from ATRA alone. The concentration-response curves for Nr0b1 and Wnt4 were nonlinear. Mean fold-induction of these genes increased with 10−6 and 10−5 M MEHP, but fell to a level similar to ATRA alone in the ATRA/10−4 M MEHP exposure, producing an inverted U-shaped concentration response. For Nr0b1, the difference between the 10−5 M and 10−6 M MEHP co-exposure groups was statistically significant.
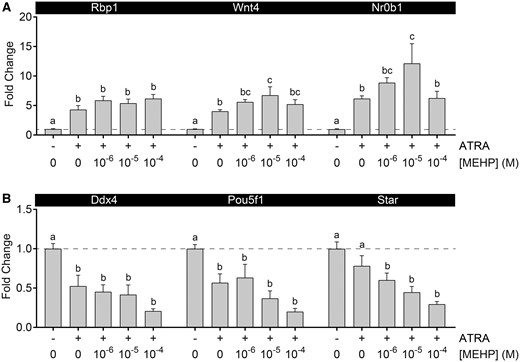
MEHP and ATRA altered testicular gene expression interactively. A, All MEHP and ATRA treatment groups enhanced expression of Rbp1. ATRA induction of Wnt4 and Nr0b1 expression was enhanced by 10−5 M MEHP, but not 10−4 MEHP, resulting in an inverted U-shaped concentration-response. B, All treatment groups resulted in suppression of Ddx4 and Pou5f1 expression. Star expression was not significantly suppressed by 10−6 M ATRA alone, but was significantly reduced with addition of all MEHP concentrations. On x-axis, “−” indicates no ATRA; “+” indicates 10−6 M ATRA. Values reported in chart are mean ± SEM. Dashed line at y = 1. n = 26 testes in vehicle control group and 6–7 testes per treated group. Within each gene, bars not connected by the same letter are significantly different (p < .05) by 1-way ANOVA followed by Tukey’s post hoc test.
Co-Exposure to MEHP and ATRA Resulted in Germ Cell Depletion and Loss of Leydig Cell Function
Intact seminiferous cords from samples cultured in vehicle media had a normal distribution of basally located DMRT1-expressing Sertoli cells and contained DDX4-positive germ cells. Exposure to the high dose of ATRA alone resulted in sections with only a small number of germ cells, not contained within well-defined seminiferous cords, and dispersion of DMRT1-positive cells throughout the section. Exposure to the high dose of MEHP, with or without ATRA, resulted in widespread depletion of germ cells but retention of seminiferous cords with basally located DMRT1-positive Sertoli cells (Figure 5). Cleaved caspase-3 IHC in samples treated for 1–3 days with high dose of MEHP, alone or in combination with ATRA, confirmed that the mechanism of germ cell loss was apoptosis (Supplementary Figure 1). Germ cell apoptosis was significantly higher in the co-exposure group than ATRA alone day 1 and higher than vehicle on day 3. In all treatments, cleaved caspase-3 labeling of cultured testes persisted longer than in the vehicle samples, in which cleaved caspase-3 labeling was greatest on day 1 and was barely detected on days 2 and 3. MNGs were rarely observed and were not induced by any treatment. In the MEHP concentration-response experiment, 2 of 19 vehicle-treated samples and 1 of 19 MEHP-treated samples (from the 10−6 M MEHP treatment) had at least 1 MNG. In the co-exposure experiment, 2 of 25 vehicle samples, 3 of 6 ATRA slides, and 4 of 19 samples from any co-exposure group had at least one MNG.
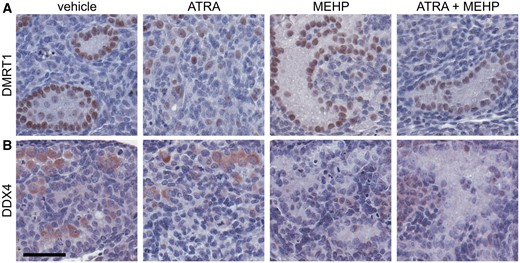
Germ cells were depleted by MEHP and co-exposure. A, Following 3 days of culture, DMRT1-expressing Sertoli cells are present in all samples. In ATRA treatment group, Sertoli cells are dispersed throughout section, but in all other groups, defined cords can be observed. B, Immunohistochemical labeling of the germ cell cytoplasmic marker DDX4 confirms that germ cells are present in seminiferous cords of 3-day cultures treated with vehicle media. Germ cells are largely present within well-defined cords, but occasionally present outside of cords. Following treatment with 10−6 M ATRA, fewer germ cells are visible, and they are outside of defined cords. 10−4 M MEHP and 10−6 M ATRA + 10−4 M MEHP cultures are largely devoid of germ cells, and sections show prominent germ cell-depleted cords. Scale bar = 50 µm. The full-color image is available online.
Testosterone concentration in tissue culture media was significantly reduced by all treatments tested on day 3 of culture (Figure 6). Reduction of testosterone by the co-exposure was at least additive, with testosterone levels equal to 44.3%, 11.0%, and 4.4% of control in ATRA, MEHP, and co-exposure groups, respectively. All differences between groups were statistically significant except for the difference between MEHP and the co-exposure. Both the ATRA and MEHP factors were significant in the 2-way ANOVA, as was the interaction term. This indicates reduced production of testosterone by Leydig cells in the presence of ATRA, MEHP, or the co-exposure.
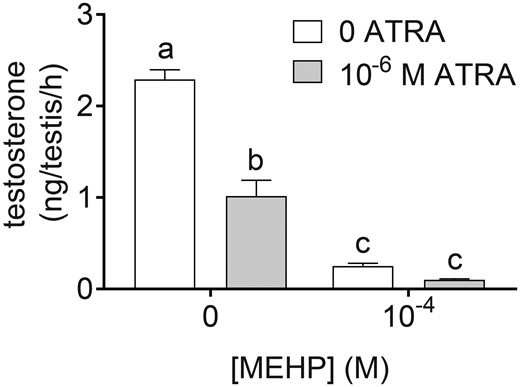
ATRA and MEHP reduced testosterone secretion from cultured testes. Testosterone was measured by ELISA in media from the third day of culture, representing secretion from 48–72 h following the initiation of the experiment, in media containing 10−4 M MEHP, 10−6 M ATRA, or 10−4 M MEHP + 10−6 M ATRA, or vehicle control. On the third day of culture, testosterone secretion was decreased in all treatment groups, relative to vehicle. MEHP and co-exposure groups had significantly lower testosterone than ATRA alone. All groups differed from each other, except for 10−4 M MEHP and the co-exposure. Groups not connected by the same letter are significantly different (p < .05) by 2-way ANOVA followed by Tukey’s multiple comparison test. ATRA (p < .0001), MEHP (p < .0001), and the interaction term (p = .0002) were all significant by 2-way ANOVA. n = 7–9 samples per treatment group and 25 vehicle samples.
ATRA and MEHP Altered Testicular FOXL2 Expression
Following 3 days of culture, exposure of ex vivo fetal testes to 10−6 M ATRA increased the number and intensity of testicular somatic cells expressing the ovarian transcription factor FOXL2 (Figure 7). With the addition of MEHP to cultures in the co-exposure study, this effect of ATRA was reduced in magnitude. FOXL2-positive cell count was not significantly greater than the vehicle control average for any co-exposure group, and the 10−5 M MEHP co-exposure group had significantly lower FOXL2-positive cell density than ATRA alone. Notably, the majority of FOXL2-positive cells in all cultures were found in the interstitium or in highly disorganized areas of ATRA-treated sections. No cells that could be morphologically identified as germ cells were FOXL2-positive. However, a small number of FOXL2-posive cells could be identified as possible Sertoli or peritubular myoid cells, based on morphology and localization relative to the basement membrane of seminiferous cords, especially in co-exposure samples where cord architecture was retained. This suggests that multiple somatic cell types account for at least some of the FOXL2-expressing cells in ATRA-treated testis cultures.
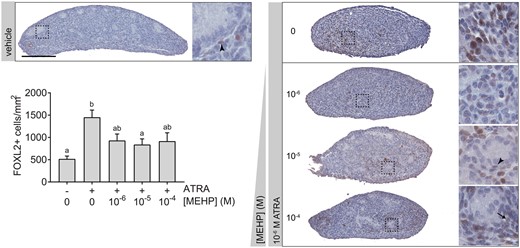
ATRA-mediated induction of FOXL2 was weakly opposed by MEHP. Vehicle-treated testis cultures exhibited little FOXL2 expression on day 3 of culture. Across all samples, most FOXL2-positive cells appeared in the interstitium. However, some weakly positive cells appeared to be possible Sertoli cells (arrow) or peritubular myoid cells (arrowheads) based on localization and nuclear morphology. ATRA exposure significantly increased the number of FOXL2-positive cells per unit area in 3-day fetal testis cultures. With addition of MEHP, FOXL2-positive cells appeared to be reduced in number and intensity. This decrease was significant with addition of 10−5 M MEHP. On x-axis, “−” indicates no ATRA; “+” indicates 10−6 M ATRA. Bars not connected by the same letter are significantly different (p < .05) by 1-way ANOVA followed by Tukey’s test. Overall ANOVA p = .0002. Chart depicts mean ± SEM of 12 testes in vehicle control group and 3 testes per treated group. Scale bar = 200 µm. The full-color image is available online.
DISCUSSION
Using this ex vivo testis culture model, we found that ATRA exposure resulted in disorganization of seminiferous cords in cultured fetal testes through a process in which expression of genes involved in ovary development is enhanced and testicular somatic cell fate maintenance is disrupted (Spade et al., 2018). In this study, high-concentration MEHP exposure resulted in some of the same effects, including moderate activation of RA signaling, as evidenced by Rbp1 expression, and enhanced expression of Wnt4 and Nr0b1 (Figure 2). However, MEHP exposure slightly increased seminiferous cord number (Figure 1). In co-exposure experiments, MEHP opposed the disorganizing effect of ATRA (Figure 3), but still resulted in germ cell loss (Figure 5), while gene expression results provided further evidence of interaction between ATRA and MEHP (Figure 4). These results demonstrate that MEHP interacts with ATRA, resulting in both enhancement and inhibition of some ATRA effects, indicating that RA signaling is disrupted by phthalate exposure, contributing to phthalate toxicity.
Phthalate exposure is known to alter seminiferous cord morphology in vivo, resulting in localization of Sertoli cells outside of seminiferous cords and increased seminiferous cord diameter, which is related to inhibition of late gestational seminiferous cord maturation (Barlow and Foster, 2003; Gaido et al., 2007; Lara et al., 2017; Spade et al., 2015). Paradoxically, MEHP exposure in utero enhances basement membrane development and thickness (Klinefelter et al., 2012). The enhancement of basement membrane thickness suggests effects of phthalates on Sertoli or peritubular myoid cells, which jointly secrete basement membrane components (Skinner et al., 1985). Seminiferous cord development is a critical event in the development of the bipotential gonad into testis, driven by the expression of Sox9 in Sertoli cells and the self-assembly of Sertoli cells around clusters of germ cells (Brennan and Capel, 2004). That phthalates oppose the seminiferous cord disorganizing effects of ATRA (Figure 3) presents significant evidence that altered seminiferous cord development is a significant component of the fetal testis toxicity of phthalates, which may be directly mediated by effects on Sertoli or peritubular myoid cells. This mechanism is consistent with recent publications that have argued that phthalates disrupt signaling for sex determination in the testis by disrupting expression of sex determination factors, such as Sry and Sox9 (Du et al., 2017; Wang et al., 2015,, 2016).
The gene expression panel tested in this study was chosen based on the effects of ATRA in this ex vivo testis culture system and genes known to respond to phthalates. Increased Rbp1 is a canonical response to activated RAR signaling (Balmer and Blomhoff, 2002). It was therefore a novel and surprising finding that MEHP alone enhanced Rbp1 expression. The combination of MEHP and ATRA increased Rbp1 expression slightly beyond the level induced by ATRA alone, but this difference was not statistically significant. More surprisingly still, exposure to the combination of ATRA and MEHP produced complex concentration-response curves for expression of Wnt4 and Nr0b1 (Figure 4). These two genes, the products of which are critical for determining the balance of signaling between testis and ovary development pathways in gonadal sex determination (Brennan and Capel, 2004; Minkina et al., 2014; Nicol and Yao, 2015; Uhlenhaut et al., 2009), were up-regulated by ATRA alone. The addition of MEHP produced a nonlinear response in which expression of Wnt4 and Nr0b1 increased, then decreased, indicating that the mechanism by which ATRA-induced expression of these genes can be saturated, or that ATRA and MEHP can act cooperatively or antagonistically at different concentrations.
The nonlinear gene expression response for Wnt4 and Nr0b1 is consistent with the concentration-response of the seminiferous cord number. The presence of FOXL2-expressing cells in testes cultured with ATRA (Figure 7) is evidence that the disorganized phenotype associated with ATRA exposure is related to a disruption of testicular cell fate maintenance, as our companion paper reported (Spade et al., 2018), and as has been supported by other recent studies (Bowles et al., 2018; Nicol et al., 2018). In this process, too, there is an antagonistic relationship between the two compounds. The saturation or antagonism observed with exposure to the highest MEHP concentration in the co-exposure study may explain this paradox. Notably, some published studies conducted in in utero exposure models have shown an influence of phthalates on the expression of Nr0b1 (Euling et al., 2013; Liu et al., 2005; Plummer et al., 2007; Ungewitter et al., 2017), as well as targets of RA signaling including Crabp2 (Plummer et al., 2007). In fact, of the 328 genes identified as differentially regulated by DBP in 1 of 4 phthalate studies in a meta-analysis (Euling et al., 2013), 11 are known or suspected targets of RARs (Balmer and Blomhoff, 2002), including Cryab, Dab2, Egr1, Fbp2, Fos, Gja1, Mapk1, Serpinh1, Star, Tgfb3, and Vcam1.
Exposure of cultured fetal testes to both MEHP and ATRA caused germ cell loss (Figure 5). Cleaved caspase-3 IHC confirmed the mechanism as apoptosis, and indicated that the co-exposure resulted in the highest rate of germ cell apoptosis over 3 days of culture (Supplementary Figure 1). Notably, during normal testis development, germ cells become quiescent at approximately GD 17.5, after which time both germ cell proliferation and apoptosis cease (Culty, 2009, 2013). In this experimental system, germ cell death in vehicle-treated samples is consistent with in vivo data, with germ cell death decreasing from the onset of culture at GD 15 over the course of the 3-day culture. In MEHP and co-exposure-treated samples, cleaved caspase-3 IHC labeling persisted into days 2–3 of culture, unlike the vehicle. We did not observe induction of MNGs following exposure to MEHP and/or ATRA in the present study. MNGs have been reported to be induced by MEHP in cultured GD 20 and PND 3 rat testes (Boisvert et al., 2016; Jones et al., 2015). However, the absence of MNGs in rat testes cultured beginning on GD 15 is consistent with the late gestational window of sensitivity for induction of MNGs by phthalates, which appears to begin around the start of the germ cell quiescent period on GD 18 (Ferrara et al., 2006; Spade et al., 2015).
Another previously demonstrated effect of both ATRA and MEHP in the rat fetal testis is a reduction in testicular testosterone (Habert et al., 2014b; Livera et al., 2000). We observed testosterone reduction by both ATRA and MEHP on day 3 of culture, and the interaction term of the 2-way ANOVA was significant (Figure 6). This is consistent with Star gene expression measured in this study: MEHP alone decreased Star expression, consistent with the in vivo mechanism by which phthalates decrease testicular testosterone, and the addition of MEHP enhanced the reduction of Star expression by ATRA. A possible explanation of this effect is the altered expression of Nr0b1 produced by both MEHP and ATRA exposure, as DAX1, the gene product of Nr0b1, inhibits expression of steroidogenesis genes by inhibiting the transcription factor SF-1 (Crawford et al., 1998; Goodfellow and Camerino, 1999). The anti-androgenic effect of phthalates has been reliably reproduced by in utero exposure of fetal rats to phthalates with C4-C8 aliphatic side chains (Furr et al., 2014; Hannas et al., 2011; Parks et al., 2000). However, some of the morphologic effects of phthalates on fetal testis development have been observed in mice or in human tissue models, without significant decreases in testosterone (Gaido et al., 2007; Habert et al., 2014b; Heger et al., 2012; Johnson et al., 2012; Mitchell et al., 2012; Spade et al., 2014). Therefore, it appears that reduced testosterone is not necessary or sufficient to produce the seminiferous cord effects that are observed following phthalate exposure. This suggests that the mechanisms of phthalate toxicity depend on other molecular initiating events, potentially including disruption of RA signaling.
This study provided several lines of evidence for interactions between MEHP and ATRA in the fetal testis. However, further mechanistic studies will be required to identify the nature of those interactions, particularly given that some effects of ATRA appeared to be inhibited by MEHP, while others were enhanced. While the genes and proteins selected for analysis in this study are known to respond to both phthalates and RA, it is likely that additional factors mediate this interaction. Phthalates are likely to have primary targets in Leydig, Sertoli, and/or germ cells. Phthalates are peroxisome proliferators, and PPAR activation is involved in phthalate toxicity in the adult testis (Gazouli et al., 2002; Ward et al., 1998), but there is little evidence that phthalates activate canonical PPAR signaling in the fetal testis (Hannas et al., 2012). It has been reported that phthalate exposure can result in inhibition of RA signaling through PPAR-RAR crosstalk in mouse Sertoli cells (Bhattacharya et al., 2005; Dufour et al., 2003), that phthalates can interfere with RA metabolism (Chen and Reese, 2016), and that phthalates might bind directly to RXRs (Sarath Josh et al., 2014), all of which indicates that RA signaling is a likely mechanism by which phthalates could exert effects on the testis. This hypothesis is consistent with the interactive effects of ATRA and MEHP on testicular development and function observed in this study. This is also consistent with recent observations that phthalate exposure alters expression of sex determination genes, including Sry, Sox9, and Dazl (Du et al., 2017; Wang et al., 2016), as well as research on the central role of the transcription factor COUP-TFII in both the response to RA and phthalates and in regression of the male reproductive tract (Jorgensen et al., 2015; van den Driesche et al., 2012; Zhao et al., 2017). Alteration of RA signaling by phthalates during development would also potentially be consistent with observations of teratogenic effects in some phthalate studies (Gray et al., 2016; Ungewitter et al., 2017). Further, phthalates have been shown to interact with glucocorticoid receptor signaling (Drake et al., 2009) and estrogen signaling (Jones et al., 2015), indicating that disruption of multiple nuclear receptor-mediated signaling pathways could contribute to phthalate toxicity. Future research efforts will investigate the testicular cell types in which ATRA and MEHP alter RA signaling during testis development and the mechanisms by which those changes are initiated.
SUPPLEMENTARY DATA
Supplementary data are available at Toxicological Sciences online.
ACKNOWLEDGMENTS
The authors thank Melinda Ankerman for processing and cutting of histological samples and Timothy Nolan for assistance with immunohistochemistry scoring. KB does occasional expert consulting with chemical and pharmaceutical companies. KB and SH own stock in a small start-up biotechnology company (Semma Therapeutics) developing a cell-based therapy for diabetes.
FUNDING
This work was supported by The National Institute of Environmental Health Sciences (grant numbers R01ES017272; T32ES007272; K99ES025231), National Institute of General Medical Sciences (grant number T36GM101995), Brown University Royce Fellowship, and Leadership Alliance SR-EIP. The University of Virginia Center for Research in Reproduction Ligand Assay and Analysis Core is supported by the Eunice Kennedy Shriver NICHD/NIH (NCTRI) Grant P50-HD28934.
Comments