-
PDF
- Split View
-
Views
-
Cite
Cite
Allison I. Hoch, J. Kent Leach, Concise Review: Optimizing Expansion of Bone Marrow Mesenchymal Stem/Stromal Cells for Clinical Applications, Stem Cells Translational Medicine, Volume 3, Issue 5, May 2014, Pages 643–652, https://doi.org/10.5966/sctm.2013-0196
- Share Icon Share
Abstract
Bone marrow-derived mesenchymal stem/stromal cells (MSCs) have demonstrated success in the clinical treatment of hematopoietic pathologies and cardiovascular disease and are the focus of treating other diseases of the musculoskeletal, digestive, integumentary, and nervous systems. However, during the requisite two-dimensional (2D) expansion to achieve a clinically relevant number of cells, MSCs exhibit profound degeneration in progenitor potency. Proliferation, multilineage potential, and colony-forming efficiency are fundamental progenitor properties that are abrogated by extensive monolayer culture. To harness the robust therapeutic potential of MSCs, a consistent, rapid, and minimally detrimental expansion method is necessary. Alternative expansion efforts have exhibited promise in the ability to preserve MSC progenitor potency better than the 2D paradigm by mimicking features of the native bone marrow niche. MSCs have been successfully expanded when stimulated by growth factors, under reduced oxygen tension, and in three-dimensional bioreactors. MSC therapeutic value can be optimized for clinical applications by combining system inputs to tailor culture parameters for recapitulating the niche with probes that nondestructively monitor progenitor potency. The purpose of this review is to explore how modulations in the 2D paradigm affect MSC progenitor properties and to highlight recent efforts in alternative expansion techniques.
Introduction
Mesenchymal stem/stromal cells (MSCs) are nonhematopoietic cells with tremendous potential for use in cell-based therapies because of their proliferation, multilineage potential, homing, proangiogenic capabilities, immunosuppression, and relative lack of ethical concerns compared with embryonic stem cells [1–3]. Although the community still lacks one specific identification marker for MSCs, minimal criteria have been universally used in its stead: adherence to plastic; specific surface antigen expression (including but not limited to CD105+, CD73+, CD90+, and CD45−); and in vitro differentiation toward osteoblasts, adipocytes, and chondrocytes [4]. MSCs exhibit promise in the clinical treatment of osteogenesis imperfecta [5], graft-versus-host disease [6], myocardial infarction [7], Crohn's disease [8], and both neurological [9] and inherited diseases [10]. Cellular therapies treating the breadth of these diseases require an exorbitant number of MSCs that varies among several million cells per kilogram of body weight [5, 6, 8]. However, only a limited number of bone marrow-derived MSCs (BMSCs) can be extracted from adult tissue. Specifically, BMSCs make up approximately 0.01% of mononuclear cells (MNCs) in the bone marrow, a small percentage that decreases with age [11]. Since their discovery in 1976 by Friedenstein et al. [12], MSCs have been almost exclusively expanded in vitro on tissue culture plastic (TCP) formed from processed polystyrene. Given that classic in vitro culture is distinctly different from the bone marrow niche from which the cells were extracted, it is not surprising that MSC progenitor properties deteriorate as a function of two-dimensional (2D) expansion [13–18]. Classic monolayer culture notably lacks the three-dimensional (3D) architecture and composition of bone, oxygen tension, mechanical stimuli, and paracrine signaling with other cell types. Robust proliferation, multilineage potential, and colony-forming efficiency (CFE) can be compromised by 2D culture [13–18]. By mimicking the niche, MSC progenitor potency has been enhanced amid growth factors [19–26], in reduced oxygen tension [27–37], and through alternative expansion in 3D [38–46]. Ample evidence over the past 30 years has demonstrated that the 2D paradigm compromises the potency of MSCs, yet these classically expanded MSCs are still regularly used in clinical trials. The goal of this review is to provide an impetus for furthering community efforts in both optimization and standardization so that the true benefit of MSCs in clinical therapies can be realized. This review primarily focuses on studies involving human bone marrow-derived MSCs and will explicitly specify studies using MSCs from other species or tissue compartments.
2D Expansion Negatively Affects MSC Progenitor Potency
MSCs are commonly expanded in monolayer culture to yield sufficient clinically relevant numbers of cells from aspirates and biopsies. Successful expansion techniques aim to facilitate significant increases in cell number without negatively affecting MSC therapeutic potential. As described below, an emergence of trends across the literature establishes that progenitor properties deteriorate as a function of 2D expansion.
Proliferation
MSC expansion in culture is necessary to address the shortage of MSCs found in a bone marrow aspirate. MSCs can be cultured in vitro for 8–15 passages, corresponding to approximately 25–40 population doublings and 80–120 days [13, 18, 47]. MSCs demonstrate a marked decrease in proliferation as a function of duration in culture and passage number [13, 15, 16], becoming senescent and ceasing to proliferate on reaching their Hayflick limit [47]. The average population doubling time increases from 1.3 days at primary culture to 7.7 days at the first passage and 14.7 days at the third passage [13]. Concurrent with a decreased proliferation rate, MSCs exhibit a transformation in morphology from a thin spindle shape to a flattened square shape [13]. Although it has been established that MSCs succumb to senescence during extensive in vitro culture, the optimal cell density for growth is unclear. Using rat MSCs, Neuhuber et al. found optimal cell growth when plating at 200 cells per cm2 compared with 20 cells or 2,000 cells per cm2 [48]. However, other studies suggest even lower plating densities (~1.5–200 cells per cm2) favor proliferation [25, 49, 50]. Slow growth of cells after high-density plating may be attributed to alterations in autocrine secretion by the cells or cell-to-cell contact inhibition.
Although MSCs are touted for their simplistic in vitro expansion requirements, many common 2D culture parameters exert prominent effects on proliferation. After 4-week expansion on 75-cm2 flasks, Sotiropoulou et al. found greater numbers of MSCs in Falcon flasks compared with Nunc, Greiner, and Costar. Enhanced growth on Falcon's TCP may be a result of Falcon's unique processing of polystyrene, which facilitates a consistent hydrophilic surface [25]. However, Barlow et al. found that Nunc flasks were optimal for MSC proliferation [51]. Despite the differences among TCP flasks, the two studies corroborated that α-modified minimum essential medium (α-MEM), which contains both greater concentrations and varieties of amino acids than Dulbecco's modified Eagle's medium, enhanced MSC proliferation [25, 51]. Many laboratories culture MSCs in 10% fetal bovine serum (FBS) or fetal calf serum as a nutritional supplement for essential proteins. Some laboratories use 17%–20% FBS [49], which is generally regarded as a way to enhance proliferation through increased concentrations of mitogenic factors [51]. There is a scarcity of literature examining the effects of FBS concentration on BMSC differentiation, perhaps because FBS alternatives may be more efficient in achieving bench-to-bedside cellular therapies. It remains to be seen whether human platelet lysate, chemically defined medium, and autologous serum, which circumvent xenogeneic responses, can be modified to preserve MSC progenitor properties as well as or better than FBS. In summary, MSC proliferation may be altered by isolation method, TCP, medium composition, and seeding density.
Multilineage Potential
As the nomenclature indicates, MSCs are derived from the mesenchyme and can theoretically differentiate toward the three mesenchymal lineages: bone, fat, and cartilage. Multilineage potential is universally used by the community as a way to identify an MSC [4] as well as to quantify progenitor potency. Cryopreservation [16], density gradient isolation [52], and seeding density [48] do not yield appreciable effects on MSC multilineage potential. Despite some disparities [14, 15], osteogenic potential is the most commonly retained lineage after passage 5 [13, 16–18, 48, 53] (Table 1). This collective finding across several studies using different isolation protocols, culture media, growth factor supplements, and expansion seeding density supports the theory that osteogenesis is the default differentiation pathway for MSCs [18]. It is unknown whether osteogenesis remains the default pathway for MSCs cultured on substrates other than TCP. Although MSCs retain the ability to differentiate toward the osteogenic lineage, the magnitude is compromised by 2D culture [13, 16]. Using MSCs as early as the first confluence, in vivo ectopic bone formation in nude mice was decreased 36 times compared with using fresh bone marrow [13]. Multilineage potential differs not only across bone marrow donors but also within a population of MSCs from the same donor. Only a low fraction of cells is capable of tripotential differentiation [18]. MSC applications involving prior in vitro differentiation bear a profound challenge in that knowledge of donor or clone differentiation potency cannot be obtained a priori. Regardless, MSCs may drastically lose differentiation robustness during the time in culture to assess multilineage potential.
Effect of prolonged monolayer expansion on mesenchymal stem/stromal cell multilineage potential
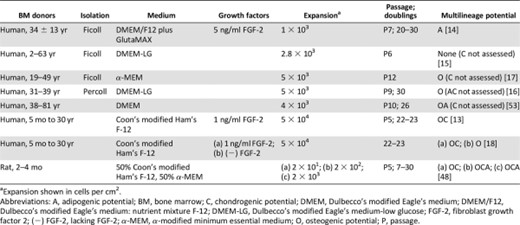
Effect of prolonged monolayer expansion on mesenchymal stem/stromal cell multilineage potential
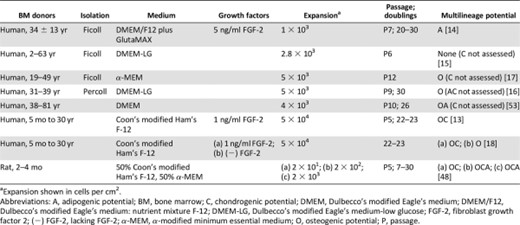
Colony-Forming Efficiency
Two parameters of colonies reveal biologically distinctive implications: colony size reflects clone proliferation, and colony density reflects clone mobility [54]. Although macrophages, endothelial cells, and lymphocytes all adhere to plastic like MSCs, only fibroblastic cells form colony forming-unit fibroblasts (CFU-F). Donor age is an important factor in CFU-F; MSCs from younger donors generated twice as many colonies as MSCs from older donors [11]. After 3-week expansion of fresh bone marrow, only 14% of the BMSCs remained clonogenic [13]. Furthermore, not every cell was capable of colony formation upon passaging [55]. Whereas CFU-F exclusively describes the number of primary cell clones, CFE is defined as the percentage of colonies per plated MSC. Following bone marrow extraction, direct plating of MSCs yielded higher CFE following subsequent plating compared with Ficoll and Percoll density gradient isolation [52]. The observed decrease in CFE following the density gradient isolations cannot be attributed to a reduced number of plated cells from purification losses because the trend describes a subsequent passage wherein the plated cell number was normalized. In contrast to density gradient isolation, direct plating may enable the initial retention of hematopoietic cells, which secrete signals that maintain a higher fraction of clonogenic MSCs [56]. CFE decreases with increasing passage [17, 55, 57], and especially at higher passages, CFE accurately correlates with remaining cumulative population doublings [57]. In addition, high CFE has been associated with strong multilineage potential [17, 58]. Specifically, the final number of clonogenic MSCs—not the final number of MSCs—correlated positively to bone formation in an ectopic nude mouse model [58]. Like proliferation [25, 49, 50], CFE increased as a result of low seeding densities [50].
Despite converging efforts at protocol standardization, several 2D culture parameters (perhaps regarded as trivial) may influence MSC properties. MSC progenitor potency may be optimized by the following protocols: directly plating MNCs without density gradient isolation [52], culturing in α-MEM [25, 51], and reseeding passaged MSCs at low densities (~1.5–200 cells per cm2) [25, 49, 50]. However, MSC progenitor potency can be improved only transiently. The potency of MSCs rapidly deteriorates as a function of 2D expansion, and this underscores the demand for alternative expansion techniques.
Alternative Approaches to Preserve MSC Progenitor Potency
Bone is a 3D substrate composed of water, organic collagen, and inorganic hydroxyapatite (HA). MSCs reside within crevices of the blood-submerged bone and among several cell types with which they engage in a complex orchestra of crosstalk (Fig. 1). MSCs respond via receptors to the potent mitogen fibroblast growth factor-2 (FGF-2) [59], which is secreted by osteoblasts and trapped in the extracellular matrix (ECM) [60, 61]. Furthermore, MSCs are subjected to the two types of mechanical stimuli of bone: strain (<2,000 microstrain) and fluid shear stress (~0.8–3 Pa), caused by interstitial fluid movement through lacunae generated during compression and tension under loading [62]. Within the bone marrow, the average oxygen (O2) tension is approximately 5% [63]. However, classic 2D culture is devoid of comparable mechanical stimuli and occurs almost exclusively in ambient air (21% O2). After the first few passages in vitro, hematopoietic cells [2] as well as an intrinsically nonadherent population of mesenchymal progenitors (NAMPs) [64] are washed away with media changes, carrying along the vital cues they communicate to MSCs [65]. In essence, numerous aspects of the bone marrow niche that delicately regulate MSC behavior [66] are egregiously absent in 2D culture. As such, many endeavors to preserve MSC progenitor potency have focused on techniques to recreate characteristics of the elaborate niche through expansion amid growth factors [19–26], in hypoxia [27–37], and through 3D expansion [38–46].
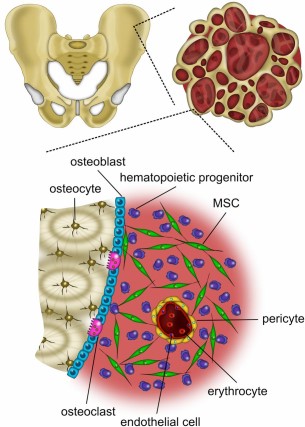
The bone marrow niche is a complex environment. Bone marrow-derived MSCs are withdrawn from the iliac crest and reside within crevices of the blood-submerged bone and interact with various cell types. Abbreviation: MSC, mesenchymal stem/stromal cell.
Growth Factors
Several efforts have sought to supplement media with growth factors that are found in the bone marrow niche. Fibroblast growth factor-4 has been shown to enhance MSC proliferation [23]. Epidermal growth factor (EGF) increased MSC proliferation and CFE [67]; however, the impact of EGF on multilineage potential is inconclusive [68, 69]. Martin et al. found that 1 ng/ml FGF-2 exerted the most profound effects on MSC progenitor potency compared with EGF, platelet-derived growth factor AA (PDGF-AA), platelet-derived growth factor BB (PDGF-BB), growth hormone (GH), insulin-like growth factor 1 (IGF-1), dexamethasone (DEX), and transforming growth factor-β1 (TGF-β1) [21]. After 8 weeks, FGF-2-conditioned MSCs yielded the greatest amount of bone formation compared with MSCs conditioned in other growth factors [21]. Moreover, FGF-2-conditioned MSCs were more spindle shaped and produced the largest size colonies when plated clonally, reflecting greater proliferation [21]. In contrast to FGF-2 and DEX, the absence of effect from other factors may be the result of maximally stimulatory concentrations found in FBS or due to MSC autocrine secretion of these factors. When compared with other stimuli, FGF-2 exhibits a more profound effect on MSC proliferation compared with EGF [21, 22, 70], PDGF-AA [21], PDGF-BB [21, 22, 70], GH [21], IGF-1 [21, 22], DEX [21], TGF-β1 [21], ascorbic acid [70], Wnt3a [70], transferrin [70], and interleukin-6 [70]. Interestingly, FGF-2 along with PDGF-BB, and insulin are included in a chemically defined medium as an alternative to FBS [71].
Table 2 summarizes the effects of FGF-2 on MSC progenitor potency during expansion. All studies in Table 2 were performed on 2D polystyrene and showed that 1–10 ng/ml FGF-2 enhances MSC proliferation [19–21, 23–26]. Furthermore, most studies found FGF-2 supplementation enhanced multilineage potential [18, 19, 21–26]. Locklin et al. found that the effects of FGF-2 on MSC proliferation and osteogenic differentiation were magnified by a seeding density of 1,000 cells per cm2 compared with 5,000 cells per cm2 [26]. FGF-2 decreased alkaline phosphatase activity [20]; however, alkaline phosphatase is cyclical and is typically lowered during mineralization [72]. Nagai et al. investigated the effects of systemically administered FGF-2 for 7 days in mice and found that 0.1 mg/kg per day FGF-2 may have increased the peak bone mass by stimulating the proliferation and differentiation of progenitor cells [73]. Although FGF-2 can enhance proliferation and multilineage potential, it is unclear whether FGF-2 enhances CFE [20–22]. CFE was decreased using 1 ng/ml FGF-2 [21, 22], but increased using 5 ng/ml FGF-2 [20]. This may suggest a biphasic response to FGF-2 concentration, yielding different effects at different concentrations.
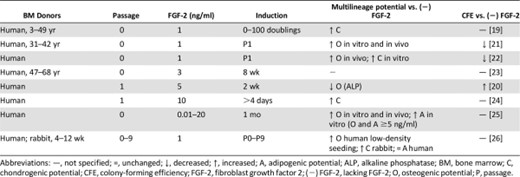
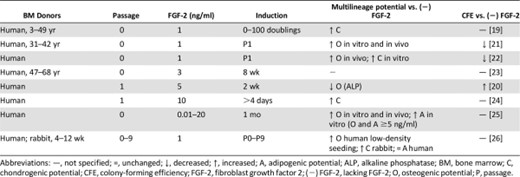
Hypoxia
Most in vitro culture occurs in 20%–21% O2 (normoxia), yet as early as 1958, Cooper et al. discovered that animal cells proliferate more rapidly in oxygen concentrations lower than 20% O2 (hypoxia) [74]. Since that observation, enhanced proliferation as a function of hypoxic culture has been observed in a diversity of cell types including pericytes [75], osteoblasts [76], hematopoietic cells [77], and MSCs [28–37]. MSCs reside in the bone marrow (4%–7% O2) [78] with an oxygen tension approximately half that of arterial blood (8%–15% O2) [79]. Emerging evidence indicates that MSCs sense oxygen concentrations through hypoxia-inducible factor (HIF) proteins such as HIF-1α [36, 37] and HIF-2α [27, 32]. Although organisms have developed complex enzymes to defend against the toxic effects of free radicals derived from oxygen [80], it is unlikely these mechanisms sufficiently protect cells at the abnormally high oxygen tensions used in vitro. Table 3 summarizes the effects of hypoxic culture on MSC progenitor potency compared with normoxic culture. MSC proliferation was enhanced by hypoxia in all studies [30, 33–37]. Primary MSCs cultured in hypoxia initially exhibited a lag phase in proliferation, after which they superseded primary MSC growth cultured in normoxia [28, 29]. The increase in MSC number can be quite profound. A 30-fold increase in MSCs was found after 6-week expansion in hypoxia [32]. Enhanced proliferation may be attributed to an observation that MSCs cultured in hypoxia maintained growth rates after reaching confluence and formed multiple cell layers, perhaps because of a lack of contact inhibition [32]. Interestingly, even a varied history of hypoxic culture augmented rat MSC proliferation [35]. Improved MSC proliferation as a function of hypoxia has also been observed using 3D poly(ethylene terephthalate) (PET) scaffolds [33].
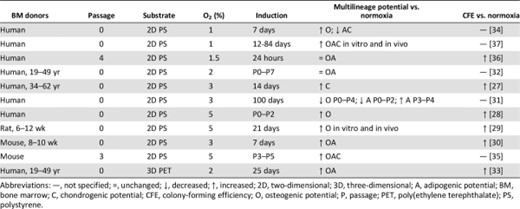
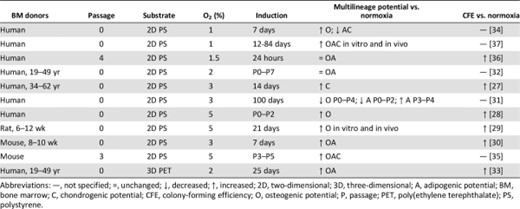
Hypoxia can also preserve multilineage potential and CFE (Table 3). MSCs conditioned in hypoxia through six passages exhibited improved multilineage differentiation in vitro and bone, fat, and cartilage formation in vivo [37]. On 3D PET scaffolds, MSCs expanded in hypoxia for 14 days exhibited enhanced osteogenic differentiation [33]. Lennon et al. found increased osteogenic potential when rat MSCs were cultured in any hypoxic conditioning regimen compared with normoxia [35]. In contrast, the history of hypoxic culture affected multilineage potential [29]. Specifically, mouse MSC multilineage potential after 1 week was improved by culture in hypoxia switched to normoxia (H-N) but when suppressed by culture in normoxia, switched to hypoxia (N-H) [29]. Hypoxia significantly increased the expression of stemness genes [29, 33, 34] and maintained a morphologically undifferentiated fibroblastic phenotype [28, 32, 37]. Collectively, hypoxia can preserve MSC progenitor properties despite variations in oxygen tensions ranging from 1% to 5% O2 and culture durations ranging from a few hours to a few months.
3D Expansion
MSCs can be expanded using scaffolds or scaffoldless approaches, usually in combination with a bioreactor (Table 4). Collectively, 3D MSC expansion has been performed on hydroxyapatite (HA) [39, 42, 58], collagen [41], chitosan gelatin and HA/chitosan gelatin [45], PET [44, 45], and gelatin microcarriers [43, 81]. This varied collection of scaffolds reflects the diverse array of tissues targeted for therapies. Because the assortment of scaffolds used in tissue engineering strategies requires distinct processing methods for construction, it is challenging to obtain a range of materials—metal, polymer, ceramic, and/or composite—with identical geometries. Within the realm of scaffolds, many parameters are pertinent to the experimental outcome including scaffold composition, pore size, porosity, pore interconnectivity, and mechanical properties [82]. Table 4 lists composition and pore size for 3D MSC expansion studies because those are two of the most consistently specified scaffold parameters. In addition to scaffold-based approaches, 3D MSC expansion can also occur in suspension [38, 83] and within spheroids [40].
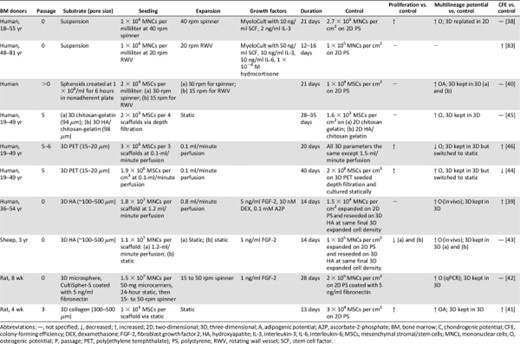
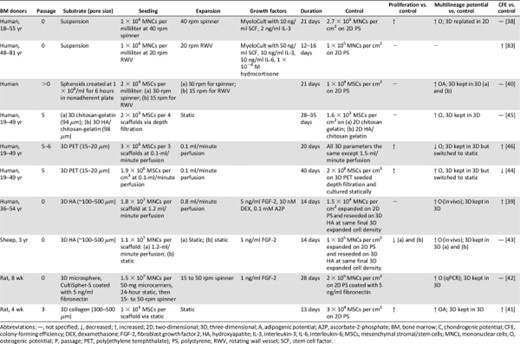
3D MSC expansion has been paired with dynamic stimulation because cell viability is restricted to 100–200 μm from the surface because of diffusive nutrient transport limitations when cultured statically [84]. Bioreactors are devices that facilitate the development of biological and/or biochemical processes through closely monitored and user-controlled operating parameters such as pH, temperature, nutrient supply, and waste removal [85]. The development and description of bioreactors has been reviewed in depth elsewhere [86]. Although spinner flasks and rotating wall vessels (RWVs) have improved convection, both strategies fail to eliminate mass transport issues within the scaffold interior [86]. Perfusion bioreactors have tackled this obstacle by driving culture medium through a scaffold that is tightly fixed to the reactor walls. MSCs have been expanded in all three bioreactors: spinner flasks [38, 43, 81, 83, 87], RWVs [40, 83], and perfusion bioreactors [39, 42, 44, 46, 58] (Table 4). Dynamic culture begets many variables such as cell-seeding density, cell-seeding technique, and cell-expansion method. Although static loading is still common, this approach exposes limitations such as low seeding efficiency [88–90] and nonuniform cell distributions within the scaffold [88, 89, 91]. Perfusion bioreactors enhanced seeding efficiencies compared with spinner flasks and static loading [91]. Table 4 reveals that many studies seeded and cultured cells using a bioreactor, likely because dynamic seeding imparts enhanced seeding efficiency [91] and streamlines the engineering process by reducing contamination risks associated with excessive scaffold handling [85]. Furthermore, Table 4 highlights that many studies specified cells per scaffold and rarely cells per surface area because surface area, especially cell-accessible surface area, is not easily determined. The lack of specified seeding density per surface area exposes a crucial limitation in consistency and collective understanding because seeding density can influence MSC differentiation in 2D [50] and 3D [88, 92]. Specifically in 3D, high-density seeding imparts increased bone mineralization [88] and increased cartilage matrix production [92]. In addition to seeding density, mechanical stimuli during 3D expansion can be tailored to achieve a desired progenitor phenotype [44, 46]. This mirrors efforts to modulate perfusion flow rates to induce MSC differentiation toward the osteogenic [93] and chondrogenic lineages [94]. Higher shear stresses induced MSC differentiation toward the osteogenic lineage [44, 93]. Finite element analysis has been used to approximate shear stress by simulating fluid flow through scaffolds [44]. However, it is difficult to empirically confirm shear stresses sensed by cells positioned on various x- and y-planes and z-depths. Velocity can be confirmed using less-than-trivial techniques such as laser Doppler velocimetry and particle-image velocimetry or using histology that corresponds to the respective geometry.
In general, 3D expansion culture—including many modulations in scaffold, bioreactor, seeding, and culture—enhanced progenitor properties compared with 2D culture. Unlike multilineage potential, proliferation and CFE assays are fairly consistent across 3D studies (Table 4). For example, cells expanded in 3D can be differentiated in 3D or cells expanded in 3D can be replated in 2D and then differentiated. The result from the former protocol incorporates facets from both cells and the microenvironment, whereas the result from the latter protocol decouples the cellular response from effects of the microenvironment. By plating 3D-expanded cells in 2D for multilineage assessment, the cell phenotype developed during the 3D expansion may be compromised because the multilineage assessment requires 2–3 weeks. Nevertheless, 3D-expansion studies demonstrate a collective trend toward preserving multilineage potential.
In contrast to expanding MSCs, some studies have expanded a fresh bone marrow aspirate by seeding a known density of MNCs in 3D [38, 39, 42, 43, 58, 83]. This enables a user to bypass the deleterious trypsinization step required before implantation. In addition to a streamlined engineering process, 3D expansion enables the retention of hematopoietic cells, which are lost in 2D culture because they do not readily adhere to TCP. In the bone marrow niche, MSCs and hematopoietic cells dwell as neighbors that support each other through secretion of various growth factors, cytokines, and chemokines [65, 83]. Cocultured hematopoietic cells may regulate the phenotype of MSCs, possibly by maintaining a higher fraction of clonogenic MSCs [56]. After 3-week perfusion expansion of a fresh bone marrow aspirate, hematopoietic cells identified as CD45+ (common leukocyte antigen) were retained on a hydroxyapatite scaffold. Furthermore, increasing hematopoietic culture medium supplements increased the percentage of CD45+ cells from 30% to 90%. Despite the modified hematopoietic culture medium, MSC proliferation capacity was sustained [39]. Mechanisms by which 3D scaffolds can preserve hematopoietic cells are unknown and merit further investigation. Other studies confirm that modified hematopoietic culture mediums can be used to retain a large hematopoietic presence in the absence of scaffolds in bioreactors [38, 83]. In addition to hematopoietic cells, the bone marrow contains nonadherent mesenchymal progenitors, which can eventually adhere [38, 64]. Both MSCs and NAMPs can differentiate toward the three mesenchymal lineages [38], yet NAMPs appear to exhibit a less committed phenotype [64]. To investigate the potency of NAMPs, Peter et al. developed a pour-off technique for expansion of this population [87]. Compared with monolayer and stirred suspension cultures, pour-off cultures that contained NAMPs supported the largest expansion of freshly harvested rat MSCs, as measured by CFE [87]. Although definitive implications of hematopoietic cell and NAMP cocultures with MSCs are not fully comprehended, the re-creation of this aspect of the niche is a promising avenue for enhancing MSC progenitor potency. Three-dimensional expansion of MSCs paired with dynamic stimulation and signals from native bone marrow populations better recapitulates the niche and may provide a streamlined alternative to standard expansion methods.
Notably, the aforementioned expansion techniques (growth factors, hypoxia, and 3D expansion) share the commonality of re-creating aspects of the bone marrow niche. It remains to be seen whether the complex combination of these efforts can synergistically preserve MSC potency.
Achieving Clinical Relevance With MSCs
MSCs have yielded improved clinical outcomes when used to treat diseases of the musculoskeletal, immune, digestive, and neurological systems. Current clinically applied doses range from 1 to 5 × 106 cells per kilogram for the treatment of patients with osteogenesis imperfecta, graft-versus-host disease, and Crohn's disease [5, 6, 8]. A bone marrow aspirate contains roughly 5–30 × 106 MNCs per milliliter and 500–3,000 MSCs per milliliter, the latter determined through CFU-F [11]. Because CFU-F frequency decreases with increasing aspirate volume [95], a 5-ml volume is generally drawn, which may contain only 2,500–6,000 MSCs in total. Thus, the demand for a fast and reliable ex vivo expansion method is necessary. The 2D paradigm exposes many weaknesses in preserving the inherent robustness of MSCs [13–18], even during extremely short in vitro culture durations [13]. MSCs have been genetically modified to overexpress human telomerase reverse transcriptase to combat telomere shortening and overcome the onset of senescence in culture [96]. However, vital limitations to human telomerase reverse transcriptase transfection include the potential to form tumors and the clinical risk of transplanting transfected cells [96]. Several efforts hold promise in preserving MSC progenitor potency during expansion by recapitulating the bone marrow niche from which BMSCs are derived.
Figure 2 illustrates a streamlined closed system of 3D MSC expansion for clinical applications. There exists a profound interplay among alternative expansion efforts and progenitor properties. Fluid flow from dynamic 3D culture, for example, enhances ECM deposition [97], which has been shown to preserve progenitor potency [98] or to induce MSCs toward a selected lineage [99]. Furthermore, perfusion culture of MSCs stimulates the synthesis of growth factors including bone morphogenetic protein-2 (BMP-2), FGF-2, TGF-β1, and vascular endothelial growth factor (VEGF) [100]. Hypoxia also enhances MSC expression and secretion of VEGF [101]. The Michaelis-Menten constitutive equations for oxygen consumption assume the rate constant is independent of time and material, and cell density primarily influences the oxygen profile [102]. However, recent studies demonstrate cellular metabolic activity and oxygen consumption rate depend on scaffold composition and geometry, presumably because of the altered cell-material interactions in the scaffold [103]. Consequently, the role of oxygen tension in 3D expansion merits further examination. It remains to be seen whether large-scale systems of bioreactors can appreciably reduce cell culture space to be attractive technology for Good Manufacturing Practice facilities. Total space allotted to cell culture may become a consideration when tens of millions of cells are required for therapies. Rafiq et al. achieved MSC expansion in 5-l spinner flasks equal to 65 fully confluent T-175 flasks [81].
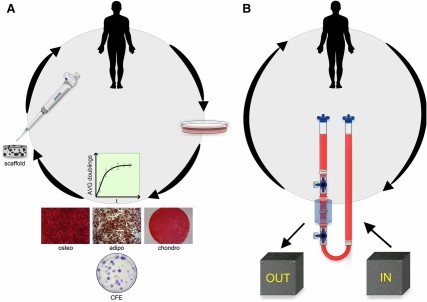
Streamlined mesenchymal stem/stromal cell (MSC) expansion. (A): Paradigm two-dimensional MSC expansion: harvested, expanded in two dimensions, analyzed for progenitor potency, trypsinized, and implanted or reinjected scaffoldless. (B): Alternate MSC expansion: harvested, expanded within a closed system with input culture controls and nondestructive output monitors for progenitor potency, implanted or reinjected scaffoldless. Abbreviations: adipo, adipogenic; AVG, average; CFE, colony-forming efficiency; chondro, chondrogenic; osteo, osteogenic.
Novel advancements in sensor and probe technology can lead to online monitoring of progenitor properties such as proliferation, multilineage potential, and CFE. A holistic evaluation of MSC progenitor properties will likely reflect the true therapeutic potential of the expanded population. Unfortunately, the assessment of MSC progenitor properties is oftentimes destructive and time consuming. Innovative sensors and probes are being developed such as the enzyme-linked immunosorbent assay-inspired “laboratory on a chip” to detect biomolecules at the point of care to revolutionize medicine in remote and resource-limited areas [104]. Commercially available “strip tests” can diagnose tuberculosis via antigens in HIV-infected patients within 30 minutes. A nondestructive multimodal diagnostic system combining time-resolved fluorescence and ultrasound imaging has been used to detect changes in ECM that correlate with biochemical and mechanical properties [105]. In addition, the number of cells can be extrapolated from a drop in oxygen tension across a perfused construct using a nondestructive methodology [106]. The intersection of cell and sensor advancements will likely spawn clinical breakthroughs.
Conclusion
Monolayer expansion is not wholly standardized, does not retain progenitor potency, and requires a deleterious trypsinization step before implantation. Alternative expansion techniques are promising avenues to better preserve progenitor properties of MSCs. When combining novel techniques to optimize MSCs for clinical applications, it is important to recognize that many parameters are delicately intertwined. Improvements in expansion technology may open the door to enhancing the efficacy of MSCs for clinical applications.
Acknowledgments
A.I.H. is grateful for financial support through the Whitaker International Program, administered by the Institute of International Education, ARCS Foundation, Inc., Northern California Chapter, and an industry/campus-supported fellowship under the Training Program in Biomolecular Technology (T32-GM008799) at the University of California, Davis.
Author Contributions
A.I.H.: conception and design, financial support, manuscript writing; J.K.L.: financial support, final approval of manuscript.
Disclosure of Potential Conflicts of Interest
The authors indicate no potential conflicts of interest.