-
PDF
- Split View
-
Views
-
Cite
Cite
Angus C Burns, Andrew J K Phillips, Martin K Rutter, Richa Saxena, Sean W Cain, Jacqueline M Lane, Genome-wide gene by environment study of time spent in daylight and chronotype identifies emerging genetic architecture underlying light sensitivity, Sleep, Volume 46, Issue 3, March 2023, zsac287, https://doi.org/10.1093/sleep/zsac287
- Share Icon Share
Abstract
Light is the primary stimulus for synchronizing the circadian clock in humans. There are very large interindividual differences in the sensitivity of the circadian clock to light. Little is currently known about the genetic basis for these interindividual differences.
We performed a genome-wide gene-by-environment interaction study (GWIS) in 280 897 individuals from the UK Biobank cohort to identify genetic variants that moderate the effect of daytime light exposure on chronotype (individual time of day preference), acting as “light sensitivity” variants for the impact of daylight on the circadian system.
We identified a genome-wide significant SNP mapped to the ARL14EP gene (rs3847634; p < 5 × 10−8), where additional minor alleles were found to enhance the morningness effect of daytime light exposure (
This study is the first to assess light as an important exposure in the genomics of chronotype and is a critical first step in uncovering the genetic architecture of human circadian light sensitivity and its links to sleep and mental health.
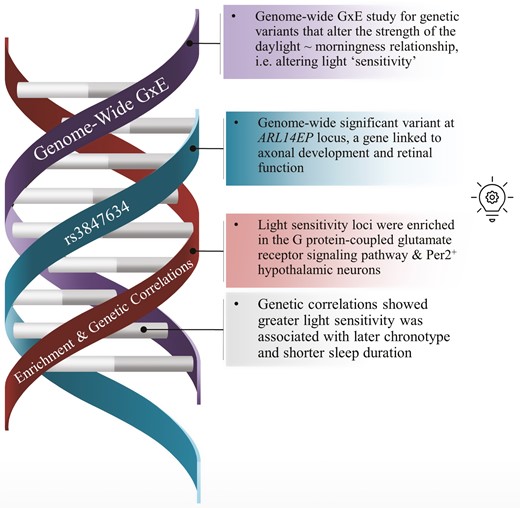
Circadian rhythms are regulated by daily light exposure patterns and there are large inter-individual differences in the sensitivity of the human circadian clock to light. We performed a genome-wide gene-by-environment interaction study of daylight exposure and chronotype and characterize an emerging genetic architecture of light sensitivity. We identified a genome-wide significant light sensitivity locus mapped to the ARL14EP gene and demonstrate that light sensitivity loci are enriched in circadian-relevant pathways and neuronal cell types. Greater light sensitivity significantly genetically correlated with later chronotype and shorter sleep duration after Bonferroni adjustment, and nominally associated with increased post-traumatic stress disorder (PTSD) and insomnia risk. This is the first study to assess the genomics of human circadian light sensitivity and its links to sleep and mental health.
Introduction
In humans, daily rhythms in gene expression, physiology, and behavior are regulated by the circadian clock located in the suprachiasmatic nuclei [1] (SCN). The circadian clock is primarily synchronized by ocular light exposure [2]. These rhythms are the overt manifestation of the activity of well-described molecular feedback-loops. Light information transmitted to the SCN from the retina via the retinohypothalamic tract (RHT) is translated into shifts in the phase of molecular biological clocks [3] by altering the expression of core clock genes [4].
There are large interindividual differences in the sensitivity of the human circadian clock to light. The circadian phase shifting response of humans to the same light stimulus can vary by as much as two hours [5] and recent work has shown that the melatonin suppression response, a measure of circadian light sensitivity, can vary between individuals by over an order of magnitude [6]. These interindividual differences play a role in the etiology of delayed sleep-wake phase disorder (DSWPD) [7], major depressive disorder [8, 9], bipolar disorder [10, 11], and other sleep disturbances [12]. Light sensitivity has also been related to tolerance of shift work, with individuals varying in their ability to adjust their biological clock so that their biological day aligns with the environmental night [13–15].
Circadian light sensitivity is a heritable trait [16], as measured by twin MZ-DZ correlations. Chronotype, a proxy-measure of circadian phase [17], has significant SNP-heritability (SNP h2 range = 0.12–0.37) in published GWAS and loci for this trait are enriched for circadian clock and glutamate signaling pathways [18, 19]. These findings indicate that both circadian light sensitivity and the downstream traits it impacts are in part determined by genetic variation in the human population. A common variable-number tandem repeat polymorphism in the PER3 gene has been associated with light sensitivity [20] in a small sample; however, no other work has yet examined the genomics of circadian light sensitivity.
The timing of light exposure has a differential impact on the timing of circadian rhythms, as described by the human light phase-response curve (PRC). Light exposure in the late night and early day advances the rhythm while light exposure in the evening and early night delays the rhythm [21]. Consistent with the light PRC, daytime light exposure tends to produce advances in the phase of the circadian clock, and thus is robustly associated with earlier chronotype and greater ease of awakening [22–24].
Here, we leverage the phenotypic association between daytime light exposure and chronotype to conduct a Genome-Wide Interaction Study (GWIS) of loci that dampen or enhance this effect. Using unrelated individuals from the UK Biobank, we test for gene-by-environment (GxE) interactions between SNP alleles and daytime light exposure on chronotype to identify the genetic architecture of light sensitivity.
Methods
UK Biobank and genetic data
The UK Biobank prospective general population cohort contains more than 502 000 UK residents (aged 37–73 years; ~54% women) recruited via National Health Service (NHS) patient registers from 2006 to 2010, with the study population described in detail elsewhere [25, 26]. Participants provided extensive demographic, lifestyle, health, mood, and physical information via assessment and touch-screen questionnaires as well as physiological samples for the purpose of genome-wide array genotyping. Participants who accepted the invitation to join the UK Biobank cohort provided written, informed consent, and the UK Biobank has generic ethical approval from the North West Multi-Center Research Ethics Committee (ref 11/NW/03820). The current analyses were conducted under UK Biobank application number 6818 (Martin Rutter).
In brief, blood, saliva, and urine were collected from participants, and DNA was extracted from the buffy coat samples. Participant DNA was genotyped on two arrays, Affymetrix UK BiLEVE Axiom array (initial 50 000 participants) and the Affymetrix UK Biobank Axiom Array with >95% common content and genotypes for ~800 000 autosomal SNPs imputed to two reference panels (Haplotype Reference Consortium (HRC) and UK10K haplotype resource). Genotypes were called using Affymetrix Power Tools software. Sample and SNPs for quality control were selected from a set of 489 212 samples across 812 428 unique markers. Sample quality control (QC) was conducted using 605 876 high-quality autosomal markers. Samples were removed for high missingness or heterozygosity (968 samples) and sex chromosome abnormalities (652 samples). Genotypes for 488 377 samples passed sample QC (~99.9% of total samples). Marker-based QC measures were tested in the European ancestry subset (n = 463 844), which was identified based on principal components of ancestry. SNPs were tested for batch effects (197 SNPs/ batch), plate effects (284 SNPs/batch), Hardy–Weinberg equilibrium (572 SNPs/ batch), sex effects (45 SNPs/batch), array effects (5417 SNPs), and discordance across control replicates (622 on UK BiLEVE Axiom array and 632 UK Biobank Axiom array; p value < 10−12 or <95% for all tests).
For each batch (106 batches total), markers that failed at least one test were set to missing. Before imputation, 805 426 SNPs passed QC in at least one batch (>99% of the array content). Population structure was captured by principal component analysis on the samples using a subset of high-quality (missingness < 1.5%), high-frequency (>2.5%) SNPs (~100 000 SNPs) and identified the subsample of white British descent. In addition to the calculated population structure by the UK Biobank, we locally further clustered participants into four ancestry clusters using K-means clustering on the principal components, identifying 453 964 participants of European ancestry. Related individuals were identified by estimating kinship coefficients for all pairs of samples, using only markers weakly informative of ancestral background. For the current analysis, individuals of non-white ethnicity and related individuals were excluded to limit confounding effects. The UK Biobank centrally imputed autosomal SNPs to UK10K haplotype, 1000 Genomes Phase 3, and Haplotype Reference Consortium (HRC). Autosomal SNPs were pre-phased using SHAPEIT3 and imputed using IMPUTE4. In total ~96 million SNPs were imputed. We used ~15.0M HRC imputed variants with an imputation r2 ≥ 0.3, MAF ≥ 0.001 (0.1%), and with a Hardy–Weinberg equilibrium (HWE) p > 1 × 10−12 (chi-squared; 1 degree of freedom). We excluded non-HRC imputed variants. Further details on the UK Biobank genotyping, quality control and imputation procedures can be found elsewhere [27].
Measurement of time spent in daylight, chronotype, and other self-reported phenotypes
At the baseline assessment, all participants self-reported how many hours they spent outdoors during the day on a typical day in both summer and winter (UK Biobank data-fields 1050 and 1060). Participants reported an integer using a touch-screen number pad or selected among alternate options including “Less than an hour a day”, “Do not know”, or “Prefer not to answer”. Initial cleaning of the data involved excluding participants that rated “Prefer not to answer” or “Do not know”, recoding “Less than an hour a day” as zero (n = 98 431), and excluding values larger than the typical day length in the United Kingdom during summer (16 h; n = 253) and winter (8 h; n = 5474). Summer and winter reports were strongly correlated (Pearson’s r = 0.65), indicating people who spent more time in outdoor light in winter also tended to do so in summer. Furthermore, a subset of approximately 20 000 participants completed a repeat assessment of this question from 2012 to 2013. Both summer and winter reports were strongly correlated (both r > 0.6) across repeat assessments, indicating good reliability of the measure. To yield a single measure of time spent in outdoor light, winter and summer reports were averaged within participants. For analysis, extreme bins (9–12 h spent outdoors) with low density [total n = 1016 (0.2%)] were collapsed into a single bin. Finally, due to the rightward skew in the distribution of the time spent in outdoor light, a log10 transformation was applied. We extracted self-reported chronotype from the baseline assessment (“Morningness-Eveningness”; data-field 1180) which was measured with the question “Do you consider yourself to be?” with six options: “Definitely a ‘morning’ person”, “More a ‘morning’ than ‘evening’ person”, “More an ‘evening’ than a ‘morning’ person”, “Definitely an ‘evening’ person”, “Do not know” or “Prefer not to answer”. The latter two categories were coded as missing. Participants further self-reported age and sex. Finally, weight and height were measured, and body-mass index (BMI) was calculated as weight (kg)/height2 (m2; UK Biobank data field 21001).
Genome-wide association studies for light sensitivity in the UK Biobank
A genome-wide interaction study (GWIS) for circadian light sensitivity was conducted in unrelated individuals of white British ancestry (n = 280 897) to reduce confounding by stratification and kinship using the –gxe function in PLINK [28]. The logarithm of time spent in daylight (continuous) was used as the moderating exposure for the genotype effect on chronotype (continuous) with the following equation:
Here, SNP refers to the allelic dosage at a given SNP coded additively, daylight refers to the log-transformed average time spent outdoors during the day and the covariates included in the model were age, sex, genotyping array (UK BiLEVE or UK Biobank Axiom), BMI, and the top ten principal components of ancestry to control for population stratification. The interaction term (
In supplement to the GWIS, we conducted two separate GWAS of chronotype stratified by time spent in daylight to examine whether light exposure modifies the genetic architecture of chronotype. Low (≤1.5 h; n = 121 922) and high (≥3.5 h; n = 118 429) daytime light exposure groups were derived by applying a quartile split of the time spent in daylight variable, with the two intermediary quartiles excluded. Within these stratified samples, genetic association analysis across the autosomes was performed in European participants with BOLT-LMM [29] linear mixed models allowing for relatedness using an additive genetic model adjusting for age, sex, ten principal components of ancestry, genotyping array, and genetic correlation matrix.
A maximum SNP missingness of 10% and per-sample missingness of 40% was tolerated. A SNP imputation quality threshold of 0.80 and minor allele frequency of 0.001 (0.1%) were used for stratified GWAS analyses. A genome-wide significance threshold of 5 × 10−8 was used to identify significant SNPs.
Gene, pathway, tissue, and single-cell expression
Post-GWIS gene based and pathway analyses were carried out with MAGMA [30] in FUMA [31]. Gene based analysis was performed using default MAGMA parameters and a SNP-wide mean model of SNPs mapped to 18 960 protein coding genes (Bonferroni p = .05/18 960 = 2.64 × 10−6). Pathway analysis was conducted using MAGMA [30] gene-set analysis in FUMA, which tests 10 894 gene sets (curated gene sets = 4728; gene ontology terms = 6166) for enrichment using the full distribution of SNP p values from the GWIS (Bonferroni p = .05/10 894 = 4.69 × 10−6). Tissue enrichment analyses (GTEx V8) were conducted using MAGMA gene-property analysis in FUMA using default parameters to test the relationship between tissue-specific gene-expression profiles and the GWIS light sensitivity associations. Cell-type specificity analysis was conducted in the same manner, using single-cell RNA-seq gene-expression profiles as the outcome, on the three circadian hypothalamic cell subtypes (Per2+, VIP+, & NMS+) identified in Romanov, Zeisel, Bakker, Girach, Hellysaz, Tomer, Alpár, Mulder, Clotman, Keimpema, Hsueh, Crow, Martens, Schwindling, Calvigioni, Bains, Máté, Szabó, Yanagawa, Zhang, Rendeiro, Farlik, Uhlén, Wulff, Bock, Broberger, Deisseroth, Hökfelt, Linnarsson, Horvath, and Harkany [32]. This single-cell dataset was chosen given the critical role of the hypothalamus in circadian photoreception and to improve power as superordinate brain tissues can have highly heterogenous gene expression profiles [33] that impact power to detect true associations. Queries of expression, methylation, and histone modification QTLs for genome-wide significant loci were done using QTLbase [34] and EyeGEx via FUMA [35].
Genetic correlations
Linkage Disequilibrium (LD) score regression was implemented in the R package GenomicSEM[36] to examine the genetic correlation (rg) between GWIS light sensitivity genetic architecture (indexed by
Results
Phenotypic results
The median time spent in daylight among UK Biobank participants was 2.5 h (IQR = 1.5–3.5 h) and time spent in daylight was correlated with chronotype, such that greater daylight exposure was associated with greater morningness (r = −0.10, p < 5 × 10−150). This association has been previously reported [24] and is independent of lifestyle, demographic, and employment covariates. Demographic characteristics of the GWIS sample are presented in Supplemental Table 1.
Genome-wide interaction study
The Manhattan and Q-Q plots for the GWIS of light sensitivity SNPs using 14 055 103 imputed variants in unrelated white British UK Biobank participants (n = 280 897; λGC = 1.04) are presented in Figure 1, A and B. A single genome-wide significant hit, rs3847634 (see Supplemental Figure 1 for locus plot), was identified that mapped to the ARL14EP/FSHB genomic region on chromosome 11 (chr11:30,344,598-30,359,774),
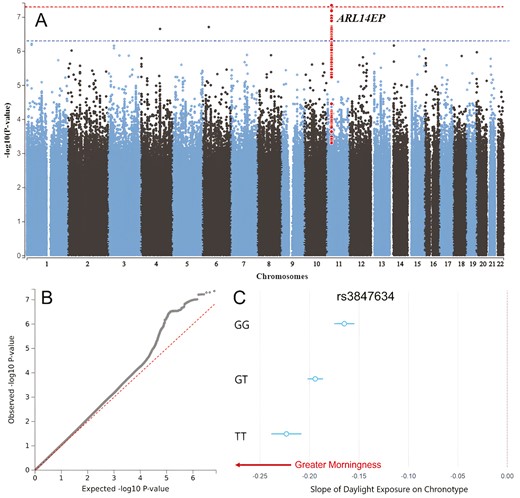
Genome-wide interaction study of light sensitivity. (A) Manhattan and (B) Q–Q plots of genome-wide interaction study (GWIS) for daylight exposure on chronotype, horizontal red dotted line indicates genome-wide significance threshold p < 5 × 10−8. The genome-wide significant SNP rs3847634 at the ARL14EP locus and SNPs in LD with it are highlighted in red (C) Conditional regression plot demonstrating the interaction between genotype at rs3847634 and daylight on chronotype, such that additional T alleles confer a stronger morningness effect of daylight.
QTL, gene, and pathway analysis
The rs3847634 SNP is an expression quantitative trait locus (eQTL; QTLbase & EyeGEx) for ARL14EP in the hippocampus (β = 0.061, p = 1.15 × 10−7) and retina (β = .44, p = 5.49 × 10−10), such that additional T alleles were associated with increased ARL14EP expression in these tissues. It was also associated with increased FSHB gene expression in the brain (β =.25, p = 5.23 × 10−12; Supplemental Tables 4 & 5). Analysis of histone and methylation QTLs revealed that the T allele is associated with increased H3K27ac histone modifications (β = 0.06, p = 2.52 × 10−6, blood) and reduced methylation (cg06241208; chr11:30,344,200; β = −0.34, p = 3.76 × 10−16, blood monocytes; Supplemental Table 6) at the promoter region of the ARL14EP gene.
MAGMA gene-based tests combining SNP-level information within a gene did not identify any Bonferroni-significant genes (see Supplemental Figure 2; Supplemental Table 7). However, suggestive signals were identified in the HOMER1 (p = 1.37 × 10−5), GOLIM4 (p = 3.34 × 10−5), and ARL14EP (p = 4.43 × 10−5) genes. Pathway analysis combining gene-level information identified a single Bonferroni-significant Gene-Ontology pathway, the G Protein-Coupled Glutamate Receptor Signaling Pathway (p = 1.87 × 10−6; see Supplemental Table 8), which contains 13 genes, including HOMER1 and the glutamate metabotropic receptor 1 gene (MgluR1/GRM1; Gene-based test p = .006; see Supplemental Table 8). Additionally, genes identified in the light sensitivity GWIS by QTL and positional mapping (RGS12 & RIC8B) significantly overlapped with the Gene Ontology G Protein Alpha Subunit Binding (padj =.01; see Supplemental Figure 3).
Tissue and single-cell expression analysis
MAGMA tissue-expression analysis did not identify significant enrichment in any of the 53 superordinate GTEx tissue types (Supplemental Figure 4), nor any of the brain samples across the stages of development in the BrainSpan database (Supplemental Figure 5). Single-cell RNA-seq gene-property analysis of circadian subtypes of hypothalamic neurons [32] revealed a Bonferroni-significant enrichment in Per2+ hypothalamic cells (p = .007; Bonferroni p = .017), but not in VIP+ or NMS+ circadian subtypes (Supplemental Table 9).
Genetic correlations
We performed LD-score regression [41] (LDSC) analyses to assess the genetic overlap of light sensitivity (i.e. the genetic architecture of loci moderating the effect of daylight on chronotype) and publicly available sleep, circadian, psychiatric, and metabolic traits (see Figure 2 and Supplemental Table 10). Light sensitivity (h2 =.2%, SE = 0.1%) had a strong negative genetic correlation with chronotype (rg = −0.62, SE = 0.10, p = 1.47 × 10−9), indicating that SNPs which tend to confer greater light sensitivity (i.e. enhancing the morningness effect of daylight on chronotype) tend to also be eveningness SNPs. Sleep duration also significantly correlated with light sensitivity (rg = 0.35, SE = 0.11, p = .002), indicating that SNPs that tend to confer lower light sensitivity tend to also confer longer sleep duration. There was a negative genetic correlation between light sensitivity and post-traumatic stress disorder (PTSD) (rg = −0.71, SE = 0.30, p = .01), indicating that SNPs associated with higher light sensitivity tended to confer greater risk for PTSD; however, the uncertainty for this estimate was large. Similarly, frequent insomnia had a significant genetic correlation with light sensitivity (rg = −0.26, SE = 0.12, p = .03), such that greater light sensitivity was associated with greater insomnia symptomology. However, the PTSD and insomnia genetic correlations were not Bonferroni significant. No other traits were significantly genetically correlated with light sensitivity.
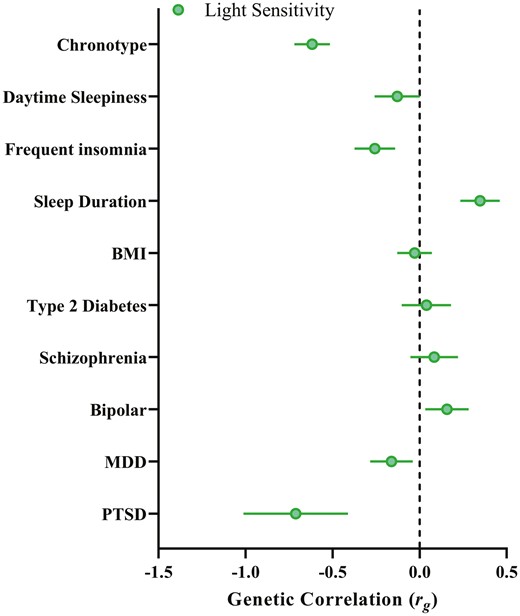
Light sensitivity genetic correlations. Genetic correlations between the light sensitivity GWIS and sleep, psychiatric and metabolic traits previously linked to light sensitivity. Note: as negative values of the βGxE term indicate an enhancing effect of the SNP on light, negative genetic correlations denote the correlation between greater light sensitivity with a given trait.
We then tested if the amount of daytime light exposure moderates the genetic correlations of chronotype with the same set of sleep, circadian, psychiatric, and metabolic traits by performing LDSC on chronotype GWAS stratified by daylight exposure (bottom quartile, low light ≤ 1.5 h, n = 121 922; top quartile, high light ≥ 3.5 h, n = 118 429; see Supplemental Figures 6 & 7 for Manhattan and Q-Q plots). The SNP-h2 of chronotype was moderated by light exposure as calculated by BOLT-REML, with heritability being higher in the low daylight exposure group (ChronotypeLow Lighth2 = 0.20, SE = 0.003; ChronotypeHigh Lighth2 = 0.16, SE = 0.003). The genetic correlation between chronotype in those exposed to low and high daytime light was strong (rg = 0.93, SE = 0.05, p = 6.92 × 10−88). The lead SNP of the low light chronotype GWAS was rs12424741, mapped to the ALG10 locus (p = 5.29 × 10−16) and the lead SNP of the high light chronotype was rs3769124 mapped to the ASB1 locus (p = 2.6 × 10−10), both previously reported [18]. Consistent with the light sensitivity genetic correlations, there was a significant difference between the genetic correlation of chronotype and sleep duration in the high (rg = 0.11, SE = 0.03, p = 1.5 × 10−4) versus low (rg = 0.02, SE = 0.03, p = .49; χ2 = 6.06, p = .01) light exposure groups, such that eveningness was associated with longer sleep duration in those exposed to high daytime light but not in those exposed to low daytime light (see Figure 3 and Supplemental Table 11). Similarly, the genetic correlations between chronotype and PTSD (χ2 = 4.81, p = .03) and chronotype and daytime sleepiness (χ2 = 4.12, p = .04) were significantly different, such that eveningness was correlated with reduced risk for PTSD and daytime sleepiness among those exposed to high levels of daytime light. Eveningness was more strongly associated with major depressive disorder among those with low daylight exposure (rg = 0.10, SE = 0.03, p = .0004) compared to those with high daylight exposure (rg = 0.06, SE = 0.03, p = .03), though this difference was only suggestive (χ2 = 2.99; p = .08).
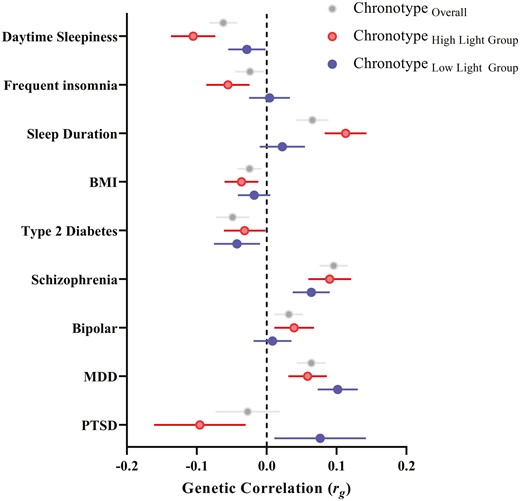
Chronotype genetic correlations stratified by daylight exposure. Genetic correlations between chronotype (eveningness) and sleep, psychiatric, and metabolic outcomes for GWAS split by environmental exposure to daylight (bottom quartile, blue points, low light ≤ 1.5 h, n = 121 922; top quartile, red points, high light ≥ 3.5 h, n = 118 429). Grey points depict the genetic correlations of the published chronotype GWAS with sleep, psychiatric, and metabolic outcomes for comparison.
Discussion
Here we report the first study of genome-wide gene by environment interactions for daytime light exposure on chronotype and uncover an emerging genetic architecture of human circadian light sensitivity. We identified a novel locus, candidate genes, and pathways for the modulation of the advancing effect of daytime light exposure on chronotype. We also observed enrichment for light sensitivity genes in hypothalamic Per2+ cell subtypes. Genetic correlations indicated that the genetic architecture of light sensitivity significantly overlapped with chronotype and sleep duration, while being nominally associated with sleep and psychiatric disturbance. This study provides important insights into the biological basis of human light sensitivity and its link to sleep and psychiatric traits.
We found that one locus, rs3847634, mapped to the ARL14EP gene on chromosome 11, reached genome-wide significance in the GWIS. Additional T alleles at this locus were associated with a stronger morningness effect of daytime light exposure on chronotype, thus conferring enhanced light sensitivity. In addition to identifying this GxE effect, we observed that the rs3847634 SNP reached genome-wide significance for its marginal effect on chronotype, such that additional T alleles at this locus were associated with greater eveningness, an effect previously unobserved [18, 19]. An independent SNP in the ARL14EP gene (rs621421, r2 = 0.0075 with rs3847634) that has previously been associated with chronotype [18], provides additional evidence for this gene playing a role in circadian timing. This SNP did not interact with light in the present study. Replication of this interaction in independent datasets with light exposure, chronotype, and genetic data will be important.
This result highlights the importance of considering relevant environmental exposures when examining the genomics of a given trait. While potentially counterintuitive, this result is consistent with a variant that enhances the overall effect of light on the timing of the circadian clock, independent of the timing of light exposure. Enhanced sensitivity to light would boost phase-advancing effects of daytime light exposure, thereby strengthening the association between daylight exposure and morning chronotype. However, the same enhancement of light sensitivity would also boost phase-delaying effects of evening light exposure [42]. Given modern human lighting conditions are characterized by reduced bright light exposure during the day and bright electric light exposure at night [43–46] a SNP that enhances light sensitivity would therefore be expected to confer both of these properties.
We found that rs3847634 is associated with reduced CpG methylation and increased h3k27ac histone modifications in the promoter region of the ARL14EP gene. Such epigenetic modifications are consistent with a more active gene promoter by diminishing the repressive effects of DNA methylation and opening chromatin to transcriptional machinery, respectively [47, 48]. These modifications may therefore explain our observation that rs3847634 is an eQTL for increased ARL14EP gene expression in brain and retinal tissues. Together, these findings hint at a plausible molecular mechanism for the effect of rs3847634 on ARL14EP gene expression and subsequent phenotypic effects.
The ARL14EP gene is important for appropriate brain and axonal development. In humans, ARL14EP haploinsufficiency is associated with callosal hypoplasia while its knockdown in animal models results in severe axonal arborization deficits and the disruption of transcollosal connectivity [49]. WAGR syndrome (Wilms’ tumour, Aniridia, Genital abnormalities, and mental Retardation) is caused by an 11p14-12 deletion that includes the ARL14EP gene and is characterized by a photosensitivity and intellectual disability phenotype [50]. Furthermore, an independent SNP at the ARL14EP locus is associated with retinal refractive error [51] and non-synonymous mutations in the CRD domain of the ARL14EP protein are associated with neurodevelopmental disorders [52, 53]. There appears to be overlap in biological function of ARL14EP with other genes that encode for axon development/guidance (via semaphorins, ephrins). The semaphorin Sema6a is hyper-expressed in ARL14EP-knockdown neurons and epigenetic editing of Sema6a gene promoters has been shown to rescue axon function in ARL14EP knockdown animal models [49]. Semaphorins and ephrins have been shown to be important in the development of retinal ganglion cells and a SNP at the Sema6a locus is associated with chronotype [18, 54, 55]. While indirect, collectively these data in concert with our findings point toward a putative role for ARL14EP in axonal development pertinent to the retina and phototransduction – for example, by altering retinohypothalamic tract development and subsequently the strength of retinal inputs to the circadian clock.
Pathway analysis identified enrichment of GWIS SNPs in the G protein-coupled glutamatergic signaling pathway. Nominal enrichment at the gene level was also observed for the HOMER1 and MgluR1 genes that are constituents of this pathway. These results are consistent with a large body of research that demonstrates the critical role of glutamatergic signaling in circadian photoreception, in particular via metabotropic glutamate receptors 1 and 5 expressed in the SCN [56, 57]. Similarly, there is strong evidence for the role of HOMER1 in circadian phototransduction. HOMER1 participates in activity-dependent control of metabotropic glutamate receptors, including MgluR1 and MgluR5, is rhythmic in the SCN with a peak in the subjective night when the clock is most sensitive to phase shifts, and its expression is induced by light exposure in the subjective night [58–60]. Furthermore, cell-type specificity analysis focusing on hypothalamic neurons expressing circadian-related genes [32] identified significant enrichment in SCN neurons expressing the core clock gene Per2. Together, these findings provide convergent evidence towards a circadian light sensitivity understanding of the genetic architecture uncovered by the present GWIS.
Finally, genetic correlation analyses identified significant genetic overlap between light sensitivity and chronotype, such that greater light sensitivity was associated with greater eveningness. This genetic correlation was strong and is likely explained by a similar phenomenon described above, whereby modern human lighting environments are depleted for daytime light [44] and enriched with night-time light [45, 61] – hence resulting in light sensitivity SNPs being associated with eveningness as individuals with a genetic load for greater light sensitivity experience greater delaying phase shifts to evening light and a later chronotype [21, 62].
Light sensitivity was also found to genetically correlate with sleep duration, such that greater light sensitivity genetic load was associated with lower sleep duration genetic load. This finding is consistent with greater light sensitivity amplifying the phase delaying effect of evening light exposure, resulting in the delayed timing of sleep and, given a constant wake time, shortened sleep [12, 45]. Greater light sensitivity may also shorten sleep via direct effects on arousal [63, 64], thereby enhancing the disruptive effects of evening light on subsequent sleep [65]. A novel association between greater light sensitivity and PTSD diagnosis was also observed. This finding may elucidate recent work demonstrating circadian dysrhythmia is linked to symptom severity in PTSD and that melatonin and phase advancing light may be effective treatments for PTSD symptoms [66–68].
We note that there are limitations of both the sample and the self-report nature of predictors and outcome measures used in this study. Firstly, there are significant changes in the properties of the circadian system that occur with age [69], including earlier phase [70], dampened amplitude [70], and reduced light sensitivity [71–73]. Older adults also spend a larger portion of their day in bright (>1000 lux) light [74]. Given these changes and the makeup of the UK Biobank sample being largely middle-older aged adults, it is feasible that the genetic architecture of light sensitivity, defined here as the moderating effect of genotype on the daylight-chronotype relationship, may differ in younger adults. Secondly, the present work relies on a self-report measure of daytime light exposure and chronotype as a proxy measure of circadian phase. Self-reported daylight exposure may broadly capture time spent in bright light during the day, however, it does not capture objective intensity and duration of exposure at the ocular level and chronotype is an imperfect proxy. These issues may have contributed to the low heritability of the observed light sensitivity trait in the present study and the low number of genome-wide significant loci due to error variance in the exposure and outcomes of interest. Future work should address these limitations by testing examining genetic light sensitivity across the lifespan using objective measures of light and, if feasible, circadian phase. Finally, it is important to note that selective gene-environment correlation may provide an alternate explanation for our results, such that individuals with a greater genetic propensity to seek out more daylight may also therefore tend to have earlier chronotype.
The present work is the first to identify common genetic variation underlying light sensitivity in the human genome; its links to molecular pathways and cell subtypes in the hypothalamus; and genetic overlap with sleep duration, sleep disturbance, and PTSD. This study emphasises the relevance of light as a critical environmental exposure when studying the genomics of circadian rhythms traits and provides the basis for future lines of research to improve the methodology beyond self-report measures of light and circadian phase.
Acknowledgments
This research and Angus C. Burns was supported by a Research Training Program (RTP) scholarship from the Australian Government.
Author Contributions
Study conceptualisation and design: A.C.B., J.M.L., R.S., S.W.C., A.J.K.P., M.K.R.; Analysis: A.C.B., J.M.L.; Overseeing project: A.C.B., J.M.L., R.S., S.W.C., A.J.K.P.; Writing and editing: A.C.B., J.M.L., R.S., S.W.C., A.J.K.P., M.K.R.; Critical revisions of the manuscript: A.C.B., J.M.L., R.S., S.W.C., A.J.K.P., M.K.R.
Disclosure Statements
Financial statement. None.
Conflicts of interest statement. The funder had no role in study design, data collection and analysis, decision to publish, or preparation of the manuscript. AJKP and SWC have received research funding from Delos and Versalux, and they are co-founders and co-directors of Circadian Health Innovations PTY LTD. SWC has also received research funding from Beacon Lighting and has consulted for Dyson.
Data Availability Statement
This work utilized the UK Biobank resource (application 26209; Martin Rutter).
Comments