-
PDF
- Split View
-
Views
-
Cite
Cite
Gonzalo M Vazquez-Prokopec, Amy C Morrison, Valerie Paz-Soldan, Steven T Stoddard, William Koval, Lance A Waller, T Alex Perkins, Alun L Lloyd, Helvio Astete, John Elder, Thomas W Scott, Uriel Kitron, Inapparent infections shape the transmission heterogeneity of dengue, PNAS Nexus, Volume 2, Issue 3, March 2023, pgad024, https://doi.org/10.1093/pnasnexus/pgad024
- Share Icon Share
Abstract
Transmission heterogeneity, whereby a disproportionate fraction of pathogen transmission events result from a small number of individuals or geographic locations, is an inherent property of many, if not most, infectious disease systems. For vector-borne diseases, transmission heterogeneity is inferred from the distribution of the number of vectors per host, which could lead to significant bias in situations where vector abundance and transmission risk at the household do not correlate, as is the case with dengue virus (DENV). We used data from a contact tracing study to quantify the distribution of DENV acute infections within human activity spaces (AS), the collection of residential locations an individual routinely visits, and quantified measures of virus transmission heterogeneity from two consecutive dengue outbreaks (DENV-4 and DENV-2) that occurred in the city of Iquitos, Peru. Negative-binomial distributions and Pareto fractions showed evidence of strong overdispersion in the number of DENV infections by AS and identified super-spreading units (SSUs): i.e. AS where most infections occurred. Approximately 8% of AS were identified as SSUs, contributing to more than 50% of DENV infections. SSU occurrence was associated more with DENV-2 infection than with DENV-4, a predominance of inapparent infections (74% of all infections), households with high Aedes aegypti mosquito abundance, and high host susceptibility to the circulating DENV serotype. Marked heterogeneity in dengue case distribution, and the role of inapparent infections in defining it, highlight major challenges faced by reactive interventions if those transmission units contributing the most to transmission are not identified, prioritized, and effectively treated.
Pathogen super-spreaders are generally inferred by quantifying the number of secondary infections generated by an infectious individual. Here, we analyze a comprehensive data set comprising six years of contact-cluster investigations to quantify ZAS, a place-based measure of transmission heterogeneity suitable for vector-borne pathogens, to describe the distribution of acute dengue virus infections in the city of Iquitos, Peru. Up to 8% of contact-clusters were identified as dengue super-spreaders due to the predominance of inapparent infections and the interaction between high Ae. aegypti mosquito abundance and high human susceptibility to dengue. Asymptomatic contribution to DENV heterogeneity was more marked for DENV-2 than for DENV-4. The marked heterogeneity in dengue, caused by the disproportionate role of inapparent infections, highlight important difficulties faced by existing interventions responding to reported symptomatic cases.
Introduction
For mosquito-borne diseases, the number of vectors per host has long been assumed to be a proxy for pathogen transmission risk (1–4). This assumption, however, presumes an equivalent risk-per-vector for each host, a situation that appears unlikely based on recent and ongoing research (2, 5). For dengue virus (DENV), evidence of strong heterogeneity in human biting attractiveness to Aedes aegypti mosquitoes (6, 7), the occurrence of contacts in locations other than an individual's home given the daytime biting behavior of Ae. aegypti (8), and spatial and temporal variations in the number of vectors and infectious human–mosquito contacts (9, 10), critically challenge the hypothesized association between vector density and risk of pathogen transmission (11–13). Revisiting the approaches used to quantify transmission risk will require accounting for multiple sources of heterogeneity that influence where, when, and how human-vector contacts occur (5).
Longitudinal dengue cohort studies performed in the Amazon city of Iquitos, Peru, identified a person's “activity space” as an appropriate scale to quantify DENV transmission (8, 14–17). An activity space (AS), often quantified using surveys or GPS technology, is defined as all locations in addition to the home that are visited by an individual during daytime routine movements that can lead to her/his exposure to bites from Ae. aegypti (8). Contact-cluster investigations also revealed that dengue illness modulates human mobility, including time spent at residential locations (18, 19), and modifies the composition of their AS, overall human–mosquito contacts, and the probability of onwards DENV transmission (11). Furthermore, such contact-cluster investigations quantified a strong epidemiological link between DENV infection in a cluster index case and the intensity of DENV transmission within contacts sampled at the AS (15, 16), implying that dengue transmission is stronger within AS units than within isolated households. Other sources of variability in the DENV system that potentially influence transmission are associated with important biological differences between the four immunologically distinct, but related, virus serotypes as well as genetic differences within individual serotypes (20, 21). Complex within-host immune responses to sequential serotype infections lead to a strong correlation between age and DENV seroprevalence and influence the rate of symptomatic DENV illness (22–24), which can range from fully asymptomatic (with no perceptible symptoms), to symptomatic illness with severity ranging from inapparent, where symptoms are mild or often unrecognized, to severe illness, which requires hospitalization and intensive care. Two factors, the predominance (70–80%) of asymptomatic and inapparent DENV infections (25, 26), and the demonstrated ability of asymptomatic DENV carriers to be infectious to Ae. aegypti (21), highlight the need to include this “silent majority” in estimates of virus transmission (11, 27). Approaches to quantify transmission intensity at the individual level, that also detect the presence of inapparent infections while accounting for other sources of heterogeneity, are needed to better describe DENV epidemiology.
The distribution of secondary infections (also called the “offspring distribution”, Z (28)), has been used to characterize empirically the transmission structure of a myriad of directly transmitted pathogens (28, 29). By fitting a negative-binomial distribution, with variance σ2 = μ(1 + μ/k) and mean equal to µ, to the observed offspring distribution Z, and exploring the value of its dispersion parameter (k), studies have revealed the occurrence of strong heterogeneity in pathogen transmission emerging from a small number of super-spreaders, which exert a strong influence on the structure and explosiveness of epidemics (28, 30). A lower k is evidence of a higher degree of super-spreading, and values of k lower than 1 imply substantial super-spreading, as recently shown for SARS-CoV-2 (31, 32) and other pathogens (28, 29).
For vector-borne diseases, inferring Z is significantly challenged by the indirect nature of pathogen transmission. Vectors acquiring an infection from a primary case remain infectious for life and can move between premises, extending the temporal and spatial dimensions of pathogen transmission. To overcome these limitations, we propose the quantification of the number of acute infections linked to an individual's activity space, ZAS (see Methods), rather than the number of cases generated from direct contact with the individual (Z). This is a subtle but relevant difference (place-based rather than individual-based distribution), to adapt the estimate of Z to the complexities of vector-borne disease systems. More specifically, ZAS should not be interpreted as Z, in that the metric is not estimated for an individual (the index case) or a specific location (the index case's home). Instead, ZAS aggregates all active infections occurring within all locations reported as visited by an index case to generate a measure of the intensity of pathogen circulation within that activity space. Analyzing ZAS data from hundreds of index cases can provide insights into the drivers of pathogen circulation within socially defined transmission units (15). For pathogens for which the index case house is not a strong predictor of risk (due to an important proportion of the exposure occurring outside the home), ZAS may thus prove to be an alternative to location-specific effective reproduction numbers (Rt) (e.g. (33)).
Here, we analyze a unique dataset comprising six years of contact-cluster investigations (the prospective identification of individuals living in houses visited by a DENV febrile individual prior to symptom onset with the goal of identifying all potential virus exposure locations within an AS) in Iquitos, Peru, and two different DENV serotype invasions (see online supplementary material Text) to quantify ZAS for DENV, and assess the role of epidemiological, immunological, and entomological characteristics in shaping virus transmission heterogeneity. Our primary goal for the study was to characterize transmission heterogeneity for DENV, not the reconstruction of virus transmission chains and the role of index cases in onwards transmission (a common inference from Z for directly transmitted pathogens). Instead, we sought to test the hypotheses that (1) inapparent infections shape the distribution of DENV within AS units and (2) entomological measures correlate with epidemiological data only after accounting for inapparent infections and different measures of human DENV susceptibility.
Results
From October 2008 through September 2014, active surveillance of the longitudinal cohort led to the initiation of 1,094 contact-cluster investigations due to the detection of a febrile participant. A total of 257 febrile participants were confirmed by PCR as infected with DENV, leading to the activation of the contact-cluster investigation and to consider them as “index cases’ of each contact cluster (Fig. 1). The breakdown of serotypes among the 257 index cases was: DENV-1 (N = 4), DENV-2 (N = 174), DENV-3 (N = 3), DENV-4 (N = 72), or an unidentified DENV serotype (N = 4) (Table S1). The study occurred during a period of rapid serotype transition, when DENV-4 (a novel introduction in Iquitos (16)) was replaced by DENV-2 during the third year of the DENV-4 invasion (Fig. 1). This replacement occurred despite high DENV-4 susceptibility in the study population (Fig. S1 and (34)). The relatively high DENV-2 attack rate compared to DENV-4 (Fig. S2) helps explain why most of the AS analyzed were initiated by index cases infected with DENV-2. Consequently, DENV-1 and DENV-3 were excluded from further analyses given the low number of infections detected (Fig. S2, Table S1). A total 2,034 contacts provided a blood specimen that was tested by PCR (out of 3,974, 51%, reported to be actively living in all contact locations). Of the 2,034 contacts, 235 (11.6%) were found infected with DENV. When assessed by study physicians in their homes at the time of enrollment and in subsequent follow-up visits, more than half the DENV-infected contacts reported not having any detectable DENV symptoms (Table S1, Fig. S3). As they could either have been fully asymptomatic or to have manifested undetermined DENV symptoms, we will conservatively treat these infections as “inapparent” (Table S1). When looking at contacts, the inapparent:symptomatic ratio was higher for DENV-2 than for DENV-4 and varied by age (Fig. S3).
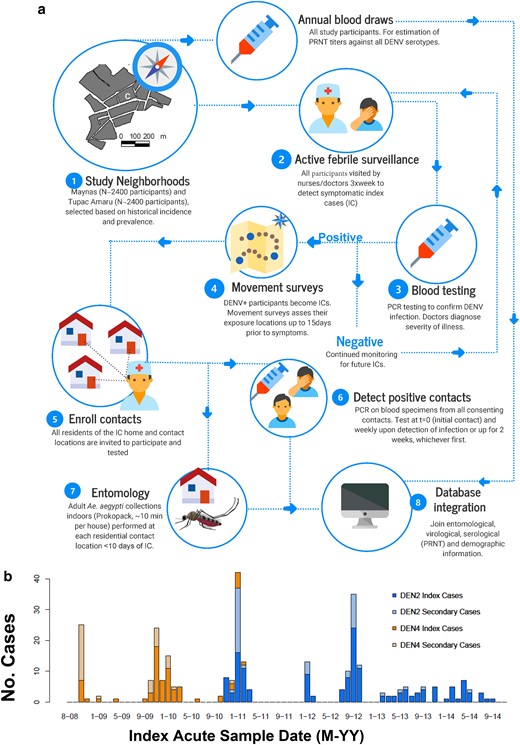
Quantifying DENV transmission heterogeneity. (a) The contact-cluster study design incorporated data from active surveillance in Iquitos, Peru, with two entomological surveys to quantify the offspring distribution of DENV infections. (b) Temporal distribution of the 253 index cases and their associated secondary cases by serotype.
Over the entire study period and neighborhoods, both DENV-2 and DENV-4 exhibited overdispersion in ZAS (Fig. 2a and b). ZAS estimates excluded the index cases and were neither associated with the number of samples nor the number of residents per activity space (Fig. S4). For each serotype and both combined, overdispersion in ZAS (k < 0.5) was quantified for all time separations between the onset of symptoms of contacts and the index case, Dt (Fig. 2c, Table S2). Extreme overdispersion (k < 0.1) was quantified only when inapparent infections were accounted for (Fig. 2d, Table S2). For most Dt values, the value of k and its 95%CI were lower for inapparent than for symptomatic individuals, and for DENV-2 compared to DENV-4 (Table S2). When only symptomatic individuals were considered, ZAS showed no signs of extreme overdispersion for Dt values up to 10 days (Fig. 2d).
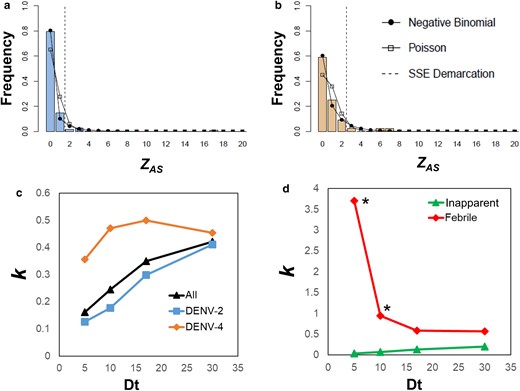
Heterogeneities in DENV transmission. The number of acute infections per activity space, ZAS, was estimated for 257 index cases detected in Iquitos from 2008 to 2014. Histograms in (a) and (b) show ZAS values across all clusters for DENV-2 and DENV-4, respectively. (c) Estimates of k, the dispersion parameter for a negative-binomial distribution, for each virus and the time of detection of an acute DENV infection (Dt) in a different person in the same activity space. (d) Estimates of k as a function of Dt for symptomatic and inapparent infections. All data points, with the exception of those with * had their parameter k being higher than unity (Table S2).
We identified 21 super-spreading units (SSUs, AS that had extremely high number of cases), 10 DENV-2 and 11 DENV-4, comprising 8.2% of all ASs, but containing 55% of all detected acute infections. Table S3 shows attributes for these SSUs and Fig. S4 shows the distribution of the number of locations in both SSU and non-SSU AS. Inapparent infections constituted the majority (71.2%) of infections in SSUs (Table S3). There was no significant correlation between ZAS and the number of residents (Pearson's correlation, t = 1.29, df = 19, P = 0.21) or the number of locations (t = 0.92, df = 19, P = 0.37) per AS (Fig. S5). While significant overdispersion was found at the AS level, we found no evidence of it when the count of acute DENV infections by house was analyzed (Fig. S6), indicating that transmission heterogeneity occurs at the AS level, rather than at the level of individual locations.
An alternative approach to empirically measure transmission heterogeneity, the Pareto fraction of ZAS (representing the fraction of active DENV infections that occurred in a given fraction of ASs), quantified a significant deviation from the null hypothesis of no overdispersion for both DENV serotypes and illness levels (Table S4). For symptomatic infections only, the Pareto fraction was always below the 60:40 ratio (meaning, 40% of the AS accumulated 60% of all infections) (Fig. 3, Table S4). When inapparent infections were included in the analysis, the Pareto fraction increased for both virus serotypes and symptoms, with overall (DENV 2–4) fractions of 66:34 for symptomatic + inapparent infections combined (Fig. 3b).
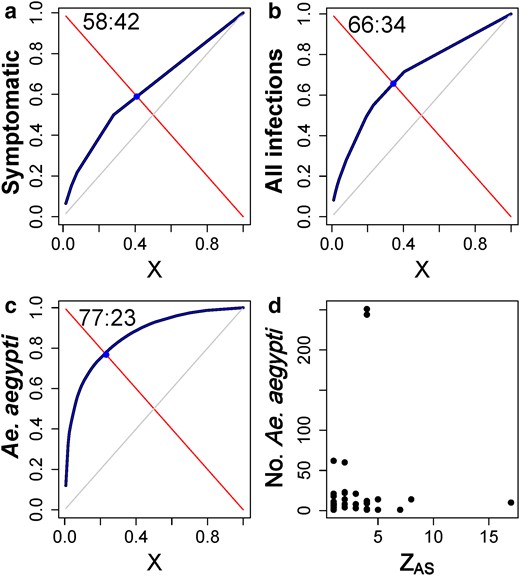
Fraction of AS units contributing with most DENV cases. (a–c) Plots showing the estimation of the Pareto fractions of ZAS (shown as cumulative fraction, blue) representing the %th fraction of cases occurring in the %th fraction of AS units. The Pareto fraction is shown in ratio format (##:##). Gray lines show 50:50 Pareto fraction. Fits were performed for (a) symptomatic infections, (b) all infections (symptomatic and inapparent) and (c) the number of Ae. aegypti per AS. Panel (d) shows the number of Ae. Aegypti and ZAS.
Applying the Pareto fraction to the total number of Ae. aegypti per AS resulted in 25% of AS accounting for 75% of all mosquitoes (Fig. 3c). ASs with the highest contribution to ZAS were not the ones with the largest Ae. aegypti infestations (Fig. 3d; Spearman Rank correlation, S = 13,999, P = 0.93). Only 15% of SSUs showed extreme overdispersion of the total number of Ae. aegypti adults collected indoors with no significant association among them (χ2 = 0.1, df = 2; P = 0.999). These results indicate that the distribution of Ae. aegypti alone is insufficient to explain DENV transmission heterogeneity and SSEs at the activity space level.
We hypothesized that DENV entomological risk is confounded by the level of susceptibility to each serotype in the human population (13, 35). For example, in areas of low human susceptibility, vector density may not be predictive of DENV infection risk. We calculated two measures of AS-level susceptibility, the Shannon susceptibility index (SHd) and the susceptibility to the circulating serotype (Si, see Methods). We found that, in univariate analyses, only Si had a moderate association with ZAS (Fig. S7). When SSU was considered a binary variable (0 = no SSU; 1 = SSU), the probability of an activity space being detected as an SSU was significantly predicted by Si and the total number of Ae. aegypti collected per AS (Table S5, Fig. 4). At low DENV susceptibility, Ae. aegypti density did not explain the heterogeneity of DENV transmission (Fig. 4). Increases in human susceptibility to the circulating DENV serotype (≥50%) co-occurring with increasing Ae. aegypti densities were, however, associated with a sharp increase in the probability of an AS becoming a SSU (Fig. 4).
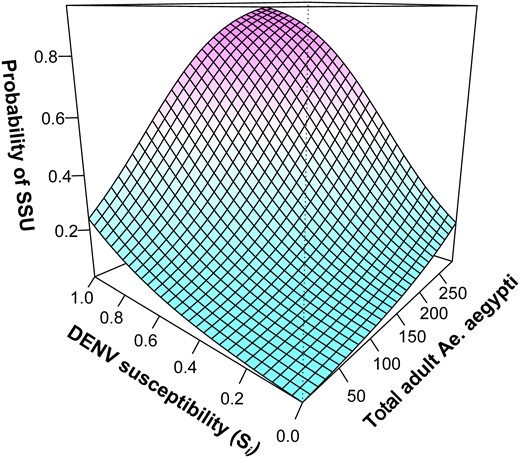
Factors influencing the emergence of DENV SSUs. GAMM-predicted association between the probability of an activity space being a DENV super-spreading unit, the total number of Ae. aegypti mosquitoes sampled in the AS, and the proportion of individuals in the AS susceptible to the circulating serotype (Si).
Discussion
Quantifying and accounting for heterogeneities in vector-borne pathogen transmission systems is of utmost relevance for improved disease prevention strategies (3, 5, 36–38). We show that AS are relevant units for characterizing dengue epidemiology and that a place-based measure of transmission heterogeneity, ZAS, can help identify vector-borne disease super-spreading and identify factors relevant to pathogen transmission. When both symptomatic and inapparent infections were accounted for, remarkable levels of aggregation in the distribution of DENV infections emerged. Approximately 8% of AS were identified as SSUs contributing more than 50% of infections. Furthermore, we show that transmission activity in the house of a symptomatic individual, alone, is a poor predictor of transmission intensity. The description of the dispersion in ZAS is, thus, a novel approach to characterize dengue epidemiology.
Beyond dengue, most of the literature describing vector-borne disease transmission heterogeneity has focused on the role of human-vector contacts rather than the distribution of human infections. For instance, Pareto fractions ranging from 60:40 and 70:30 across transmission settings in Uganda were inferred for Plasmodium falciparum-infected mosquito counts per house (36), yet such measures were not validated using human infection data. Woolhouse et al. (4) described measures of transmission heterogeneity for multiple vector-transmitted pathogens (e.g. malaria, leishmaniasis, schistosomiasis), but their finding of an 80:20 rule was based on proxies of human-vector contacts rather than actual pathogen transmission events. Given that for a variety of vector-borne diseases human mobility plays an important role in defining pathogen exposure (2, 8), contact-cluster investigations could be extended beyond dengue to refine estimates of transmission heterogeneity, including ZAS, and empirically quantify transmission heterogeneity and its causes.
A key component in the heterogeneity of dengue epidemiology is the well-established phenomenon that typically half or more of all infections are inapparent or fully asymptomatic (25, 39) and, thus, not detected by public health surveillance systems. Mathematical models suggest that people with asymptomatic and inapparent infections, given their proven ability to infect Ae. aegypti mosquitoes (21), may contribute up to 80% of the force of DENV infection (27) and onwards DENV transmission (11). Results from mathematical models fitted to longitudinal pediatric cohort data from Nicaragua indicate the role of an understudied mechanism behind the variable ratios of inapparent to symptomatic infections; i.e. immune boosting by subsequent DENV infections (22, 23). Specifically, recent exposure to homotypic or heterotypic DENV infections may “boost” an individual's immune response by moderately increasing antibody titers, which may reduce viremia and the severity of a subsequent DENV infection (22, 23). Similarly, we found that ASs that were identified as SSUs (where high rates of DENV infections were quantified) had a higher proportion of inapparent infections compared to the rest of the ASs, indirectly supporting the idea that high transmission intensity in those ASs may be underlying their lower rates of symptomatic DENV illness. Our study, combined with emerging scientific evidence from other longitudinal cohort studies (21–23, 40) and mathematical models (11, 22, 27), highlight the need to study the complex human immune response to DENV and its role in the occurrence of asymptomatic and inapparent carriers more explicitly. For example, people with asymptomtic SARS-CoV-2 infections contribute disproportionally to virus transmission and pre-symptomatic infections can be a driver of epidemics (41).
Integrating entomological and epidemiological information collected at the activity space level identified a previously hypothesized, but untested, interaction between Ae. aegypti abundance and human susceptibility to virus infection as the main drivers of DENV transmission (13). As hypothesized, entomological indices alone had no predictive utility for DENV transmission but were significantly associated with the occurrence of SSUs when human susceptibility to a serotype was measured and was high. In Iquitos, Ae. aegypti adult and pupal density calculated from cross-sectional entomological surveys separated every four months was not associated with an increase in the risk of DENV infection at the household level (42), in part due to the challenge in obtaining entomological information that is concurrent with the time when virus exposure occurred. Conversely, a detailed simulation model predicted that an individual's positive contribution to onwards transmission during an outbreak was a function of the interaction between an individual's suitability to being bitten by a mosquito and the number of mosquitoes in their home (11). Our findings emphasize the relevance of an accurate characterization of human susceptibility to DENV infection in combination with entomological information at appropriate spatial and temporal scales as an approach to improve estimates of DENV transmission risk. Also, as more studies like ours are conducted, refinements in estimates of k and the association between Ae. aegypti and DENV transmission risk may help better characterize transmission heterogeneity across settings.
We acknowledge several limitations that impacted our study. We did not sequence virus genomes and, therefore, did not examine heterogeneity in virus populations within and between ASs. Our finding that susceptibility to the circulating strain was a strong predictor of the presence of SSUs could have been refined by knowing more about specific genotypes that may have circulated in Iquitos. For instance, a study with the same cohort suggested incomplete protection against DENV-2, meaning that 43% of the detected infections had elevated DENV-2 antibodies titers prior to infection (34). It may be possible that different DENV-2 genotypes were circulating at the same time, explaining the more elevated inapparent ratio for DENV-2 than for DENV-4. The low finding of DENV-1 and DENV-3 cases also limited knowing how transmission heterogeneity is influenced in the context of polytypic DENV circulation. In Iquitos, a single serotype typically dominates (39, 43). Having more years of data may have given a chance to detect enough DENV-1 and DENV-3 cases to run analysis. Similarly, even with the high effort put in our study, only about 150 DENV cases were detected in ∼50% of the contacts providing a blood sample. Increasing the coverage of blood draws to more participants would have reduced the uncertainty in the estimates of k. Simulation studies show that, when few clusters are detected, estimates of k from a negative-binomial distribution tend to be underestimated (30). Testing mosquitoes for DENV infection would have provided an independent measure of the distribution of infection and its relationship with acute DENV human infections. Our design prevented us from identifying visitors to contact locations who may have been concurrently infected. Although study participation was high, not all house members consented to provide a blood sample (see Methods). Our estimates of heterogeneity, therefore, are likely an underestimate of the true distribution of DENV at the AS level.
Theory predicts that, for directly transmitted pathogens, the existence of marked overdispersion in Z would render blanket interventions less effective (28, 44). Experience validates such prediction. Even the most stringent COVID-19 social distancing rules have become ineffective in the presence of large gatherings that served as super-spreading events; e.g. (31, 45, 46). The strong heterogeneity in pathogen transmission has also helped guide public health efforts for the proactive local containment of pathogens such as Ebola virus or TB bacteria; e.g. contact tracing, ring vaccination (28, 29). Vector control is generally implemented in and around premises of confirmed or probable DENV cases (47). Spatial and temporal heterogeneity in transmission, and the influence of inapparent infections on virus transmission (21, 27), reveal a fundamental flaw in reactive dengue prevention programs (48). Our results show that the distribution of infections at the individual house level is indistinguishable from a random distribution. Infections tend to aggregate in specific AS that act as SSUs and are driven by an excess in the number of inapparent infections. Our findings, combined with recent results from mathematical models (48–50) indicate that, in endemic cities like Iquitos, pre-emptive interventions (i.e. performed before the beginning of a transmission season) or combining vector control and vaccines (50) may be more effective at curtailing transmission and sustainably reducing disease than a reactive intervention that is initiated after detection of symptomatic dengue cases.
Methods
Study design
Details of the contact-cluster study design, case ascertainment, basic epidemiology of DENV in Iquitos and protocols for human subjects research are described in the online supplementary material Text and elsewhere (16). Figure 1 summarizes all the methods followed to layer an active febrile surveillance with a longitudinal cohort involving ∼2,400 participants aged 5 years and older living in Maynas and Tupac Amaru, two neighborhoods of Iquitos, Peru (population ∼370,000). For the purposes of this study, we considered all individuals from the active surveillance that had a confirmed DENV infection (by PCR) as an “index case” for contact-cluster investigations (Fig. 1). All individuals residing in locations reported by index cases as visited during the 15–30 days prior to onset of the index case's symptoms and providing a blood specimen were considered as “contacts” of each contact cluster (Fig. 1). Entomological evaluations were conducted in the houses of all index cases and contacts using ∼10-minute Prokopack adult aspirators indoors (10) within 1–2 weeks of confirmation of infection of an index case (Fig. 1).
Human subjects approvals
The study protocol was approved by the University of California at Davis (Protocol #2007-15244) and Naval Medical Research Unit Six (Protocol #NMRCD.2007.0007) Institutional Review Boards (IRBs), with the latter including Peruvian representation, in compliance with all US Federal and Peruvian regulations governing the protection of human subjects. IRB authorization agreements were established for Emory University, San Diego State University, and Tulane University. The protocol was reviewed and approved by the Loreto Regional Health Department (LRHD), which oversees health research Iquitos. In all instances, consent was provided by study participants or their parent or guardian.
Data analysis
For directly transmitted diseases, Z is inferred from the array of contacts with active infections within a period compatible with the incubation period of the pathogen (28, 29). Here, we extended the approach of estimating Z to a vector-borne disease system by exploring a place-based estimate across all locations found within index cases’ AS. ZAS is thus the number of acute DENV infections found across all residential locations reported as visited by an index case during the 15-days that preceded their onset of the index case's symptoms. We estimated ZAS for each DENV serotype by location, and separately for symptomatic and inapparent DENV infections. Maximum likelihood was used to fit Poisson and negative-binomial distributions to ZAS (online supplementary material Text) and assess the value of the dispersion parameter, k, as a measure of transmission heterogeneity (30). We identified SSUs as those AS that had a number of acute DENV infections greater than the 99th centile of the equivalent Poisson distribution, with the distribution mean equal to the mean number of infections per AS (28).
Heterogeneity was also described by the Pareto fraction (36). We define Pareto fraction for ZAS from its empirical CDF (eCDF) as Cooper et al. (36), with X denoting the proportion of N observations and Y the empirical cumulative distribution: . Y(X) monotonically increases with X and intersects the line 1 − X. The point X where Y(X) = 1 − X defines the Pareto fraction. At that point, there is a X:1 − X rule, for instance if X = 0.8 then the Pareto fraction corresponds to the well-known 80:20 rule. The Pareto index for a given fraction can be calculated as We calculated the Pareto fraction and Pareto index for (1) ZAS, (2) ZAS calculated only considering symptomatic individuals and (3) the distribution of Ae. aegypti per AS. Spearman correlation was used to relate Y(X) estimated for ZAS and for the count of Ae. aegypti per AS. The code for calculating the Pareto fraction can be found in the online supplementary material Text.
Generalized additive mixed models (GAMM) were fitted to ZAS (negative-binomial link) or to a binary variable indicating whether an AS was a SSU (binomial). In using the binomial GAMM, we were interested in understanding what drives extreme overdispersion and the emergence of SSUs. We used Ae. aegypti counts per AS and a measure of human susceptibility to DENV as independent variables in univariate and as a non-linear interaction. The susceptibility to the prevailing DENV serotype, Si, was calculated as: , where NPRNT70 was the count of individuals within an AS whose PRNT70 (see online supplementary material Text for laboratory methods) was positive for the DENV serotype infecting the AS index case relative to N, which was the total individuals tested in that AS. Si takes values from 0 to 1, where 1 implies full susceptibility to the circulating serotype and 0 full protective immunity. While other measures of susceptibility were calculated (see online supplementary material Text) none had a significant fit to the data. Splines were used to fit the interaction terms, using the mgcv package in R (51). Models with single and interaction terms were compared using AIC.
Acknowledgments
We thank the residents of Iquitos for their support and participation in this study. We greatly appreciate the support of the Loreto Regional Health Department, including Drs. Hugo Rodriguez-Ferruci, Christian Carey, Carlos Alvarez, Hernan Silva and the Lic. Wilma Casanova Rojas, who all facilitated our work in Iquitos. A special thanks to Gloria Talledo for her ongoing support with the preparation of IRB protocols and reports for this project.
A special thank you goes to our Iquitos movement team (Jhonny Jorge Cordova-López, Esther Jennifer Rios López, Wendy Lorena Quiroz Flores, Alfonso Simone Vizcarra Santillan), data management personnel (Gabriela Vasquez De La Torre, Magaly Ochoa Isuiza), and the nurse technicians involved in case capture (Llerme Armas Pisco, Cesar Augusto Banda Chavez, Linder Maria Canayo Zavaleta, Clara Chávez López, Karina Chuquipiondo Vasquez, Laury Dacia Cuespan Camus, Leny Curico Manihuari, Nadia Rocio Del Rio Chavez, Salome Elespuru, Junnelhy Mireya Flores López, Juan Flores Michi, Luz Angelica Galvez, Huayllahua, Rina Gonzales Jaba, Deisy Irene Huiñapi Cambunugue, Maria Edith Juárez Baldera, Xiomara Mafaldo García, Nora Marín Romero, Nadia Tereshkova Montes Criollo, Johnni Mozombite Flores, Sandra Ivonne Moñoz Perez, Lucy Navarro Sánchez, Geraldine Ocmin Galán, Zenith María Pezo Villacorta, Fiona Stefani Pinedo Zevallos, Iris Reátegui Carrión, Zoila Martha Reategui Chota, Sadith Jovita Ricopa Manuyama, Liliana Rios López, Rubiela Nerza Rubio Briceño, Ysabel Ruiz Berger, Miranda Angela Rocio Soplin, Rosana Magaly Sotero Jiménez, Rosa Tamani Babilonia, Zenith Tamani Guerrero, Moises Tanchiva, Sarita Del Pilar Tuesta Dávila, Flora Vargas Ceras, Rita Gabriela Vasquez Orbe).
Supplementary material
Supplementary material is available at PNAS Nexus online.
Funding
This study was funded by the US National Institute of Allergy and Infectious Diseases (NIH/NIAID) award numbers P01 AI098670 and R01 AI069341 (PI TWS) and R03AI107446 (PI ACM). Further support was provided by Bill and Melinda Gates Foundation (BMGF) to the University of Notre Dame (Grant# OPP1081737), the Defense Threat Reduction Agency (DTRA), Military Infectious Disease Research Program (MIDRP, S0520_15_LI and S0572_17_LI), and the Armed Forces Health Surveillance Branch Global Emerging Infections Systems research program (GEIS) ProMIS ID: 20160390169, P0090_17_N6_1.1.1, P0106_18_N6_01.01, and P0143_19_N6. G.V.P. was supported by the US NIH/NIAID (U01AI148069).
Copyright statement
Authors Helvio Astete, Crystyan Siles, and Isabel Bazan are US Government Employees. This work was prepared as part of their official duties. Title 17 U.S.C. § 105 provides that “Copyright protection under this title is not available for any work of the United States Government”. Title 17 U.S.C. § 101 defines a US Government work as a work prepared by a military service members or employees of the US Government as part of those persons’ official duties.
Distribution statement
This manuscript has been approved for Public Release. The data on the ZAS and entomological measures per activity space are available from: http://dx.doi.org/10.17632/ktngcmv6gk.1
Disclaimer
The views expressed in this article are those of the authors and do not necessarily reflect the official policy or position of the Department of the Navy, Department of Defense, nor the US Government.
Data availability
The data on the ZAS and entomological measures per activity space are available from: http://dx.doi.org/10.17632/ktngcmv6gk.1
References
Author notes
Competing interest: The authors declare no financial interests or connections, direct or indirect, or other situations that might be considered to be a source of bias in the work reported or the conclusions, implications or opinions stated.