-
PDF
- Split View
-
Views
-
Cite
Cite
Xumei Jia, Shuo Xu, Yuting Wang, Lu Jin, Tengteng Gao, Zhijun Zhang, Chao Yang, Yubin Qing, Chao Li, Fengwang Ma, Age-dependent changes in leaf size in apple are governed by a cytokinin-integrated module, Plant Physiology, Volume 195, Issue 3, July 2024, Pages 2406–2427, https://doi.org/10.1093/plphys/kiae201
- Share Icon Share
Abstract
Plants undergo various age-dependent changes in leaf morphology during juvenile to adult vegetative stage. However, the precise molecular mechanisms governing these changes in apple (Malus domestica) remain unknown. Here, we showed that CYTOKININ OXIDASE/DEHYDROGENASE5 (MdCKX5), an age-dependent gene, encodes a functional CKX enzyme and serves as the common downstream target of SQUAMOSA PROMOTER BINDING PROTEIN-LIKE (SPL) transcription factor MdSPL14 and WRKY transcription factor MdWRKY24 to control the degradation of cytokinin (CK). As the target of mdm-microRNA156a, MdSPL14 interacts with MdWRKY24 to coordinately repress the transcription of MdCKX5 by forming the age-mediated mdm-miR156a–MdSPL14–MdWRKY24 module, which regulates age-dependent changes in CK during the juvenile-to-adult phase transition. We further demonstrated that MdARR6, a type-A ARABIDOPSIS RESPONSE REGULATOR (ARR), is a negative feedback regulator in the CK signaling pathway. Silencing of MdARR6 in apple resulted in large leaves with smaller epidermal cells and a greater number of epidermal cells. Biochemical analysis showed that the mdm-miR156a–MdSPL14–MdWRKY24 module acts as a transcriptional repressor to directly regulate MdARR6 expression, thus controlling the age-dependent changes in leaf size by reducing CK responses. These findings established a link between the age pathway and CK signaling and revealed the molecular mechanism underlying age-dependent changes during the juvenile-to-adult phase transition; our results also provide targets for the genetic improvement of the vegetative phase transition in apple.
Introduction
As plants age, they undergo 2 crucial developmental changes, including the juvenile-to-adult phase transitions and floral changes in their long life cycles (Khan et al. 2014). Many perennial woody plants flower through a prolonged juvenile phase, such as pine (Pinus tabuliformis), which takes approximately 5 to 7 years (yr) to reach reproductive age (Ma et al. 2021). Apple (Malus domestica), an economically important fruit tree, requires 3 to 5 yr to complete the juvenile-to-adult transition due to its long juvenile phase and highly heterozygous genetic background. Much progress has been made in our understanding of the mechanisms underlying the ageing pathway in the model plant Arabidopsis (Arabidopsis thaliana), but the regulatory mechanisms underlying the age-dependent changes caused by the vegetative phase transition in apple remain unclear.
The transition from the juvenile to the adult phase is known as vegetative phase change, and several leaf morphological, anatomical, and physiological traits are associated with this transition (Usami et al. 2009). Measuring these traits is a major problem in plant development because this transition is difficult to characterize in some plants that show subtle changes in leaf traits (Poethig 2013). Visible age-dependent changes can be easily distinguished by leaf shape and size, trichome production on the abaxial of the leaf, and serration of the leaf margin (Huijser and Schmid 2011). For example, the vegetative phase transition is accompanied by the appearance of trichomes on the abaxial side of leaves, an increase in blade size, the appearance of serration on the leaf margin, and a decrease in cell size in Arabidopsis (Telfer et al. 1997; Poethig 2009, 2013) and an increase in the density of stomata and leaf veins in poplar (Lawrence et al. 2021). These results indicate that a developmental decline in microRNA156 (miR156) in the age pathway is necessary for inducing these age-dependent changes (Wang et al. 2011; Wang and Wang 2015; He et al. 2018; Ahsan et al. 2019). The expression of microRNA156 gradually declines as plants age, thereby eliminating the inhibition of its target transcription factor SQUAMOSA PROMOTER BINDING PROTEIN-LIKEs SPLs (Xu et al. 2016). The molecular mechanisms by which miR156-targeted SPLs facilitate cell growth anisotropy in the abaxial epidermis at the base of the leaf blade in Arabidopsis have been recently revealed (Tang et al. 2023). However, how the miR156–SPL module precisely controls this change in leaf shape during the vegetative phase transition remains unclear.
Several studies have shown that phytohormones including gibberellin (GA), abscisic acid (ABA), brassinosteroids (BRs), jasmonic acid (JA), and cytokinin (CK) mediate the vegetative phase transition in plants (Beydler et al. 2016; Hibara et al. 2016; Werner et al. 2021; Zhou et al. 2023). For example, DELLA proteins are the central hubs of GA signaling and can promote the interaction of BRAHMA (BRM) with NUCLEAR FACTOR Y-C (NF-YC) to repress Arabidopsis flowering (Zhang et al. 2023). INSENSITIVE5 (ABI5), a key regulator in the ABA signaling pathway, regulates the transition from the juvenile to the adult phase by acting downstream of miR159 (Guo et al. 2021a). A recent study has established a connection between BRs and the age pathway and showed that BRs stabilize SPL9 and TOE1 simultaneously by BIN2-mediated phosphorylation, thereby regulating vegetative phase change in Arabidopsis (Zhou et al. 2023). CK promotes cell division and plays a crucial role in regulating the size and activity of the shoot apical meristem as well as cell proliferation and differentiation in plants (Kieber and Schaller 2018; Cortleven et al. 2019). The levels of active CKs in plants are dependent on their biosynthesis, metabolism, degradation, and transport. Active tZ-type and iP-type CKs are mainly synthesized in the roots; grafting experiments have confirmed that root-synthesized CKs can be transported to the shoot via a long-distance transport process (Kiba et al. 2013; Zhao et al. 2021). CK degradation is irreversibly catalyzed by CK oxidase/dehydrogenase (CKX) to generate adenine or adenosine (Schmulling et al. 2003). Studies of rice (Oryza sativa) have shown that knockout of OsCKX4 results in fewer crown roots and the overaccumulation of CK in the root meristem, and OsCKX11 mutants show delayed leaf senescence and increases in the number of grains (Zhang et al. 2021; Geng et al. 2023). Overexpression of type-A OsRR6 leads to dwarf phenotypes with poorly developed root systems and panicles in rice (Hirose et al. 2007), whereas the loss of type-A ARABIDOPSIS RESPONSE REGULATOR (ARR) function in ARR7 and ARR15 mutants strongly promotes shoot regeneration in Arabidopsis (Buechel et al. 2010). Nevertheless, the precise molecular mechanism underlying how the miR156–SPL module and CK metabolism control the age-dependent changes in leaves has not been previously reported in apple. Thus, identifying the components downstream of the miR156–SPL module and establishing a direct link between the miR156–SPL module and CK signaling could reveal a previously unknown molecular mechanism in the age pathway.
Vegetative phase change is a highly conserved developmental transition (Lawrence-Paul et al. 2023). As plants age, they transition through different developmental phases that result in experiencing diverse environments during those phases. Although developmental stage has a major effect on stress responses in plants, this fact is often overlooked in studies of responses to abiotic stress. To date, several studies have demonstrated the important roles of age-dependent changes in responses to various abiotic stresses in Arabidopsis and annual plants (Arshad et al. 2017; Rankenberg et al. 2021; Lawrence-Paul et al. 2023). However, little is known regarding vegetative phase change responses to the environment in woody plants, especially in apple, which has a long juvenile phase and highly heterozygous genetic background. In vitro tissue culture has been widely used for the large-scale culture of many individual woody plants (Wang et al. 2022b). There is thus a need to characterize age-dependent changes using plants at different ages from in vitro tissue culture, as this would provide insights that could be used to shorten the vegetative growth period and provide important materials for future studies of age-dependent responses to abiotic stress.
In this study, we showed that vegetative phase change-related traits in the source tree can be maintained in tissue-cultured apple plants. Omics analysis revealed that mdm-miR156a, MdCKX5, MdWRKY24, and MdARR6 are critical regulators of the age-dependent changes during the juvenile-to-adult phase transition. Molecular and biochemical studies indicated that MdCKX5 plays an important role in controlling CK levels via the age-mediated mdm-miR156a–MdSPL14–MdWRKY24 module. Furthermore, the MdSPL14–MdWRKY24 module acts as a key transcriptional hub that integrates CK signaling at the transcriptional level to coordinately regulate the transition between cell expansion and proliferation required for leaf development, thereby leading to changes in leaf size with age in apple. These findings revealed the molecular mechanism underlying age-dependent changes during the juvenile-to-adult phase transition and provide previously unknown insights that will aid studies of the vegetative phase transition, efforts to shorten the juvenile period, and improvements in the breeding of apple.
Results
Distinct leaf morphological changes during the vegetative phase change in apple
The plant vegetative phase change is characterized by various morphological changes. In the source tree, we observed that the leaves in the 1-yr-old stage (juvenile phase) were small and ellipsoid in shape, and they gradually became larger and longer with age (Supplementary Fig. S1A). The abaxial surface of the leaf blade gradually produced more trichomes and stomata with age as indicated by the much denser distribution of trichomes in 5-yr-old stages (adult phase; Supplementary Fig. S1, B and C). The epidermal cells became smaller with age (Supplementary Fig. S1D). The measurement results revealed that leaf area, stomatal density, abaxial trichome density, epidermal cell number, and the tZ content gradually increased with age, but the specific leaf area (SLA), epidermal cell area, and the expression of mdm-miRNA156 and MdCKX5 gradually decreased (Supplementary Fig. S1, E to M), indicating that age-dependent changes in leaves are key traits of vegetative phase transition in apple.
To determine whether age-dependent changes in the leaf morphology of the source tree can be maintained in tissue-cultured apple plants, explants of 3 age stages from the source tree were generated (tissue-cultured apple plants at different ages, hereafter referred to as 1y, 3y, and 5y, respectively; Fig. 1A), and subculture rooting was completed in 2 generations to minimize variation associated with long-term culture. As expected, significant differences in various leaf morphological traits, including leaf size, the area of abaxial and adaxial epidermal cells, and leaf thickness, were observed in 1y, 3y, and 5y plants (Fig. 1). We found that the leaves gradually became larger with age, and the leaf area was significantly lower in 1y plants than in 3y and 5y plants (Fig. 1, B and C). Microscopic observations showed that the shape of abaxial and adaxial epidermal cells in 1y plants was convoluted; however, cells were smaller and had barely noticeable indentations in 5y plants (Fig. 1D), which reflects a transition from cell expansion to proliferation during the vegetative phase transition. The number of abaxial and adaxial epidermal cells was significantly lower in 1y plants than in 3y and 5y plants, but the area of epidermal cells was significantly higher in 1y plants than in 3y and 5y plants (Fig. 1, F and G). Paraffin sections revealed that the palisade tissue cells of leaves in different positions of 1y plants were loosely arranged, whereas those of 3y and 5y leaves were tightly arranged (Fig. 1E); the leaves became thicker with age, but the upper epidermis became thinner (Fig. 1, H and I). Overall, these observations demonstrated that these age-dependent traits of the source tree can be maintained in tissue-cultured plants after undergoing the clonal process.
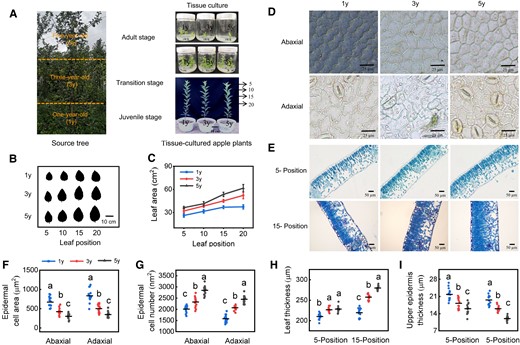
Morphological difference in 1y, 3y, and 5y tissue-cultured apple plants. A) The phenotypes of 1y, 3y, and 5y tissue-cultured apple plants. We collected stem tips of apple plants at 3 ages (1-yr-old, juvenile phase; 3-yr-old, transition phase; and 5-yr-old, adult phase, represent the transition from the juvenile to the adult phase) from the same 5-yr-old source tree and obtained apple plants at different ages via in vitro tissue culture (tissue-cultured apple plants at different ages, hereafter referred to as 1y, 3y, and 5y, respectively). B, C) Leaf area at different positions. Data are means ± Sd (n = 3). Images in B) were digitally extracted for comparison. D) Microscopic observation of adaxial and abaxial epidermal cells. Scale bar = 25 μm. E) Microscopic observations of leaf anatomy in different positions. Scale bar = 50 μm. F, G) The number and area of adaxial and abaxial epidermal cells. Data are means ± Sd (n = 14). H, I) The thickness of leaf and upper epidermis for 1y, 3y, and 5y apple leaves. Data are means ± Sd (n = 14). Values with different letters are significantly different according to 1-way ANOVA followed by Tukey's test (P < 0.05).
Multiomics analysis in leaves during the vegetative phase change in apple
To investigate the molecular mechanism underlying variation in leaf morphology during the vegetative phase transition, we performed RNA-seq and small RNA sequencing to quantify genome-wide mRNA and miRNA levels in the fully expanded leaves at 5 positions of 1y, 3y, and 5y plants (Supplementary Table S1). Three biological replicates of each age stage were sequenced, and the principal component analysis (PCA) among biological replicates was high, which indicates the high-quality and reproducibility of our sequencing results (Supplementary Fig. S2, A and B). The transcriptome analysis showed that 211, 648, and 560 differentially expressed genes (DEGs) were identified in 3 pairwise comparisons (1y vs. 3y, 1y vs. 5y, and 3y vs. 5y, respectively; Fig. 2A). Sequencing of small RNA analyses revealed that the length distribution of the miRNA ranged from 18 nt to 23 nt, and 21-nt miRNAs were the most abundant (Fig. 2B). A total of 89, 154, and 107 differentially expressed miRNAs (DEMs) were identified in the 1y vs. 3y, 1y vs. 5y, and 3y vs. 5y comparison groups, respectively (Fig. 2C). To characterize the roles of DEGs and DEMs during the vegetative phase change, we first divided them into 8 profiles based on their dynamic expression patterns among 1y, 3y, and 5y plants (Supplementary Fig. S2, C and D). The expression of DEGs and DEMs in profiles 1, 3, 4, and 6 all had linear phases starting or ending with a stable expression pattern, and the peak expression pattern of DEGs and DEMs was observed in profiles 2 and 5. In contrast, DEGs and DEMs in profiles 0 and 7 showed a linear expression pattern with age; they decreased linearly in profile 0 and increased linearly in profile 7 with age (Supplementary Fig. S2, C and D, and Table S2), indicating that the respective genes and miRNAs in the 2 profiles play continuously roles during the vegetative phase transition in apple.
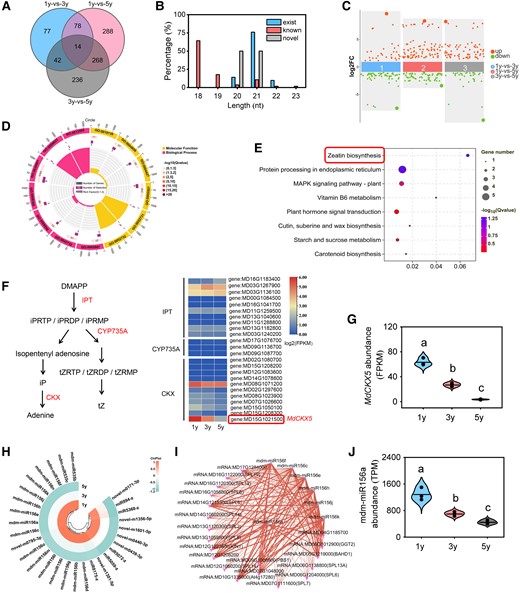
Sequencing analysis of transcriptome and small RNAs for 1y, 3y, and 5y tissue-cultured apple plants. A) Venn diagram analysis of the DEGs. B) A bar chart shows the length distribution of the identified small RNAs. C) Scatter diagram analysis of the DEMs. D) GO enrichment analysis of DEGs for profile 0. Cycle 1 (Cy1, the outermost cycle) shows 20 enriched GO terms. Cycle 2 (Cy2, the secondary outer cycle) shows the number of DEGs corresponding to GO Classify2. The color of the rectangles below the number indicates the q value (−log10). Cycle 3 (Cy3, the subinternal cycle) shows the number of upregulated and downregulated genes corresponding to GO Classify2. Cycle 4 (Cy4, the innermost cycle) shows the enriched terms in GO Classify2, which includes terms in the biological process and molecular function categories. E) KEGG pathway enrichment analysis of DEGs in profile 0. The size of the dots indicates the number of significant DEGs associated with each KEGG term. The color of the dots indicates false discovery rate (FDR)-corrected P values. FDR ≤ 0.05 as a threshold. Pathways meeting this condition were defined as significantly enriched pathways in DEGs by multiple-testing procedure. F) Schematic models for the zeatin biosynthesis pathway. DMAPP, dimethylallyl diphosphate; iPRTP, isopentenyladenosine-5′-triphosphate; iPRDP, isopentenyladenosine-5′-diphosphatei; iPRMP, N6-(delta2-isopentenyl)-adenosine 5′-monophosphate; tZRTP, trans-zeatin riboside triphosphate; tZRDP, trans-zeatin riboside diphosphate; tZRMP, trans-zeatin riboside monophosphate. Enzymes encoded by genes were marked in red, and the heatmaps show log2 (FPKM) values of each DEG. FPKM reflects the level of gene expression. Fold change ≥ 2 and P < 0.05. G) Expression patterns of MdCKX5 for 1y, 3y, and 5y tissue-cultured apple plants. Data are means ± Sd (n = 3). H) Expression patterns of DEMs for profile 0. I) Regulatory networks of DEMs and their predicted targets. Purple corresponds to miRNAs, and red corresponds to genes. The size of dots in the networks was proportional to the number of miRNAs and genes. J) Expression patterns of mdm-miR156a for 1y, 3y, and 5y tissue-cultured apple plants. TPM, transcript per million. Data are means ± Sd (n = 3). Values with different letters are significantly different according to 1-way ANOVA followed by Tukey's test (P < 0.05).
The GO analyses showed that DEGs were mainly associated with CK dehydrogenase activity (GO: 0019139), CK metabolic process (GO: 0009690), and CK catabolic process (GO: 0009823) in profile 0 (Fig. 2D); DEGs were mainly associated with protein phosphorylation (GO: 0006468), protein kinase activity (GO: 0004672), and phosphotransferase activity (GO: 0016773) in profile 7 (Supplementary Fig. S3A). KEGG enrichment analysis revealed that zeatin biosynthesis (ko00908) was the most significantly enriched pathway in profile 0 (Fig. 2E), and the MAPK signaling pathway-plant (ko04016) was the most significantly enriched pathway in profile 7 (Supplementary Fig. S3B). Previous studies have revealed that CK plays critical roles in leaf development and the juvenile-to-adult phase transition (Shani et al. 2010; Werner et al. 2021; Wang et al. 2022a); thus, the zeatin biosynthesis in profile 0 was selected as the candidate pathway for subsequent assays. Although no significance differences in the expression of all CK synthesis genes (MdIPTs, MdCYP735As) were observed among 1y, 3y, and 5y plants (Fig. 2F), a highly age-dependent expression pattern was observed for CK oxidase/dehydrogenase, MdCKX5 (Fig. 2G). To further investigate the role of MdCKX5 in the zeatin biosynthesis pathway (ko00908), gene set enrichment analysis (GSEA) was performed for all genes in the pathway (Supplementary Fig. S4A). The zeatin biosynthesis pathway was enhanced with age, and MdCKX5 was the bottom of the list L in 3 pairwise comparisons (Supplementary Fig. S4B), demonstrating that MdCKX5 plays a critical role in CK homeostasis.
Moreover, miRNA analysis revealed that 29 miRNAs, including 17 mdm-miRNA156 family members, 5 previously known members, and 7 novel members, were identified in profile 0 (Fig. 2H). Given that miRNAs perform their functions by regulating the expression of downstream target mRNAs, we used Cytoscape to analyze the associations between DEmiRNAs and predicted target genes; 192 miRNA–mRNA interactions in profile 0 were identified, which involved 9 miRNAs and 59 targets (Fig. 2I). The network contained 8 mdm-miRNA156 family members, including mdm-miR156a, mdm-miR156c, mdm-miR156e, mdm-miR156f, mdm-miR156h, mdm-miR156j, mdm-miR156k, and mdm-miR156n (Fig. 2I; Supplementary Table S3). Given that these miRNAs had the same mature sequences and cleavage sites of their targets, we detected the precursor level of these miRNAs and MdCKX5 expression level. Reverse transcription quantitative PCR (RT-qPCR) analysis showed that MdCKX5 was highly expressed in 1y plants and gradually decreased with age, which is consistent with the RNA-seq data (Supplementary Fig. S5); the pre-mdm-miR156a showed the same expression pattern with mdm-miR156 among 8 precursors of miR156 (Fig. 2J; Supplementary Fig. S6), indicating that the mdm-miR156 abundance may be mainly affected by pre-mdm-miR156a; thus, mdm-miR156a was used in subsequent experiments.
MdWRKY24 is required for the mdm-miR156a–MdSPL14 module
Mdm-miR156a was predicted to have 20 target genes in the network, including 11 MdSPLs (Fig. 2I), but no statistically significant differences in these genes were observed in our transcriptome data. The miR156–SPL module has been shown to be a conserved pathway that controls vegetative phase change in Arabidopsis (Rankenberg et al. 2021). We thus hypothesized that the miR156–SPL regulatory module might play a role in early morphological developmental stages of apple plants. To test this hypothesis, the expression of mdm-miR156a and MdSPLs at different time points (30, 120, and 210 d) of 1y, 3y, and 5y plants was analyzed by RT-qPCR. Only the expression of MdSPL14 out of the 11 MdSPLs at the 3 time points was upregulated as the mdm-miR156a level declined, but no significant differences in the expression of this gene were observed when they were grown to 210 d (Supplementary Fig. S7), which is consistent with the transcriptome data.
To validate whether MdSPL14 is the direct target of mdm-miR156a in apple (Fig. 3A), we overexpressed mdm-miR156a in leaves of 5y apple plants via transient infiltration with Agrobacterium tumefaciens EHA105 harboring mdm-miR156a-OE. This resulted in a significant decrease in the transcript levels of MdSPL14 (Fig. 3B). We next used a dual LUC-based miRNA sensor system to transiently coexpress mdm-miR156a as an effector with MdSPL14: LUC reporter in Nicotiana benthamiana leaves (Supplementary Fig. S8A). The LUC fluorescence intensity and activity of relative luciferase activity (LUC/REN) were significantly attenuated when mdm-miR156a and MdSPL14 were coexpressed (Fig. 3, C and D). This result was also confirmed by the GUS staining assay, which showed that apple calli coexpressing mdm-miR156a and MdSPL14 had lower GUS activity than calli expressing only MdSPL14 (Fig. 3E; Supplementary Fig. S8B). These results demonstrated that mdm-miR156a directly targets MdSPL14, thereby inhibiting its expression.
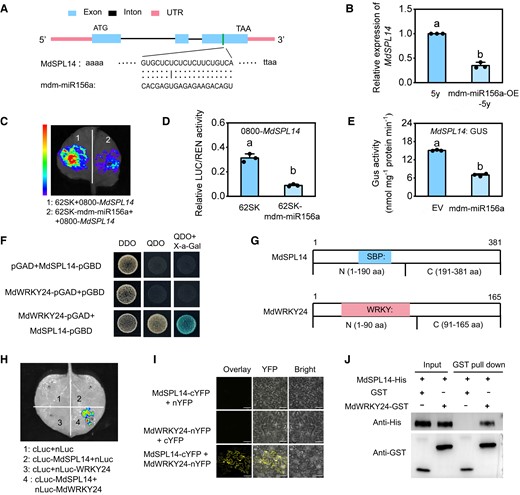
MdSPL14 is the direct target of mdm-miR156a. A) Sequence alignment of mdm-miR156a complementary sequences of MdSPL14 and schematic diagrams of the mdm-miR156a cleavage site in MdSPL14 transcripts. B) The relative expression levels of MdSPL14 in 5y and mdm-miR156-OE-5y leaves. Data are means ± Sd (n = 3). Values with different letters are significantly different according to 1-way ANOVA followed by Tukey's test (P < 0.05). C, D) LUC assay showing the suppression of mdm-miR156a on MdSPL14 in N. benthamiana. E) GUS staining assay showing that mdm-miR156a inhibits MdSPL14 expression in N. benthamiana. EV, empty vector. Data are means ± Sd (n = 3). Values with different letters are significantly different according to 1-way ANOVA followed by Tukey's test (P < 0.05). F) Y2H assay showing that MdSPL14 interacts with MdWRKY24 in yeast cells. DDO (double dropout supplements), SD medium without leucine and tryptophan; QDO (quadruple dropout supplements), SD medium without leucine, tryptophan, histidine, and adenine. G) Diagram of MdSPL14 and MdWRKY24 domain constructs. The blue and pink boxes indicated the conserved SBP and WRKY domains, respectively. H) Luciferase complementarity assay showing that fluorescence signal was only observed in sites coinjected with MdSPL14-Cluc and MdWRKY24-Nluc. I) BiFC assay showing that MdSPL14 interacts with MdWRKY24 in the nucleus and cell membranes. Scale bar = 77.3 μm. J) Pull-down assay showing that MdSPL14-His could be captured by MdWRKY24-GST.
The expression of MdWRKY24, an age-dependent transcription factor in our transcriptome data, increased linearly in 1y, 3y, and 5y plants (Supplementary Fig. S9). We asked whether the function of MdSPL14 in late developmental stages is associated with MdWRKY24 levels. To investigate this possibility, a yeast 2-hybrid (Y2H) assay was performed. Yeast cells with cotransformed MdSPL14 and MdWRKY24 grew normally (Fig. 3F). To identify the important regions of MdSPL14 and MdWRKY24 underlying the protein interaction, we divided the MdSPL14 protein into the N-terminal region (N, 1–190 aa) and the C-terminal region (C, 191–381 aa) and MdWRKY24 into the N-terminal region (N, 1–90 aa) and the C-terminal region (C, 91–165 aa; Fig. 3G). The Y2H results showed that the N regions of the MdSPL14 proteins could only interact with the N regions of MdWRKY24, indicating that the N-terminal of MdSPL14 and MdWRKY24 is a key region mediating the MdSPL14–MdWRKY24 interaction (Supplementary Fig. S10). A luciferase complementarity assay, bimolecular fluorescence complementation (BiFC), and glutathione S-transferase (GST) pull-down assay also further confirmed this interaction between MdSPL14 and MdWRKY24 (Fig. 3, H to J). These results demonstrated that MdWRKY24 physically interacts with MdSPL14 both in vitro and in vivo, and this interaction might be critical for the function of MdSPL14 in the age pathway.
MdCKX5 encodes a functional CKX enzyme
The full-length coding sequence (CDS) of MdCKX5 is 1,617 bp, and it has conserved FAD-binding and CK-binding structural domains. To investigate the cellular function of MdCKX5, the GFP-MdCKX5 fusion protein was transiently expressed in N. benthamiana leaves along with the endoplasmic reticulum (ER) marker protein RFP-p24 (Lerich et al. 2011). Laser scanning confocal microscopy revealed that the green signal of GFP-MdCKX5 merged with the red (mCherry) fluorescence of the ER marker, indicating that GFP-MdCKX5 was localized in the ER (Fig. 4A). We used RT-qPCR to analyze the tissue-specific expression of MdCKX5 in various apple organs and found that MdCKX5 was expressed in all the tissues; the expression of MdCKX5 was highest in apple roots (Fig. 4B). CKX enzymes regulate CK levels by catalyzing their irreversible degradation (Huang et al. 2023). To confirm the CK degradation activity of MdCKX5, we generated MBP-MdCKX5 fusion protein from Escherichia coli Rosetta (DE3) and purified it for enzyme assays. Given that isopentenyladenine (iP) and trans-zeatin (tZ) are the most abundant natural CKs in plants (Werner et al. 2021; Svolacchia and Sabatini 2023), the catalytic assays of the MBP-MdCKX5 protein were measured using 3 CK forms, including tZ, iP, and zeatin riboside (tZTR) as substrates. We monitored the depletion of substrates using liquid chromatography–tandem MS (LC-MS/MS). The degradation capacity of MBP-MdCKX5 fusion protein for the 3 substrates varied; MdCKX5 had higher activity for tZ and iP than for tZTR (Fig. 4C). We also treated 50-d-old seedlings with 10 µM tZ and iP and examined the expression of MdCKX5 at different time points by RT-qPCR. As expected, MdCKX5 expression in the leaves and roots was significantly upregulated after tZ and iP treatment (Fig. 4D), indicating that the expression of MdCKX5 was strongly induced by exogenous CK. These findings demonstrated that MdCKX5 degrades active CKs in apple.
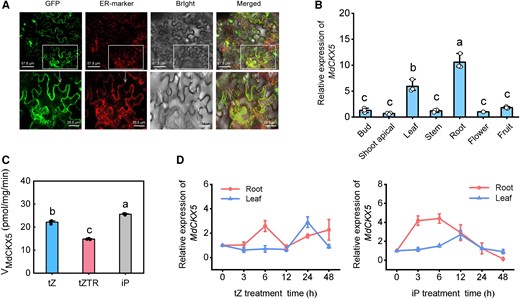
In vitro and in vivo enzyme activities of MdCKX5. A) Subcellular localization of MdCKX5-eGFP fusion protein in N. benthamiana leaves. B) Relative expression levels of MdCKX5 in different apple tissues. C) In vitro CKX activity assay of recombinant GST-MdCKX5. iP, isopentenyladenine; tZ, trans-zeatin; tZTR, zeatin riboside. D) Expression of MdCKX5 in leaves and roots following 10 μM tZ and iP treatment. Data are means ± Sd (n = 3). Values with different letters are significantly different according to 1-way ANOVA followed by Tukey's test (P < 0.05).
The MdSPL14–MdWRKY24 module controls CK levels by repressing MdCKX5 expression
In light of the above results of the multiomics analysis, we asked whether MdCKX5 plays a role in the age pathway to regulate leaf size during the vegetative phase transition in apple. We first analyzed the sequence of the 1,500-bp MdCKX5 promoter and found that it contained 4 GTAC-binding sites and 2 W-box-binding sites (Fig. 5A). To investigate the relationship between MdCKX5 and the mdm-miR156a–MdSPL14–MdWRKY24 module, we performed a yeast 1-hybrid (Y1H) assay and found that MdSPL14 and MdWRKY24 could bind to the MdCKX5 promoter, respectively (Fig. 5B). To validate the transcriptional regulatory activity of MdSPL14 and MdWRKY24 on the MdCKX5 promoter, MdSPL14 (35S: MdSPL14) and MdWRKY24 (35S: MdWRKY24) were coexpressed with the reporter pro-MdCKX5: LUC in N. benthamiana leaves, respectively, and MdSPL14 (35S: MdSPL14) and MdWRKY24 (35S: MdWRKY24) were coexpressed with the reporter 35S: LUC as a negative control, respectively (Fig. 5C). The LUC fluorescence intensity and the LUC/REN ratio were significantly lower in the experimental groups than in the control groups (Fig. 5, D and E), indicating that MdSPL14 and MdWRKY24 substantially repress MdCKX5 promoter activity. An electrophoretic mobility shift assay (EMSA) provided further evidence that MdSPL14 binds to 4 GTAC sites (P1, P2, P3, and P4) in the MdCKX5 promoter and that MdWRKY24 directly binds to 2 W-box sites (P1, P2) in vitro (Fig. 5F; Supplementary Fig. S11). These results demonstrated that both MdSPL14 and MdWRKY24 suppress MdCKX5 transcription by binding to the cis-element of the promoter.
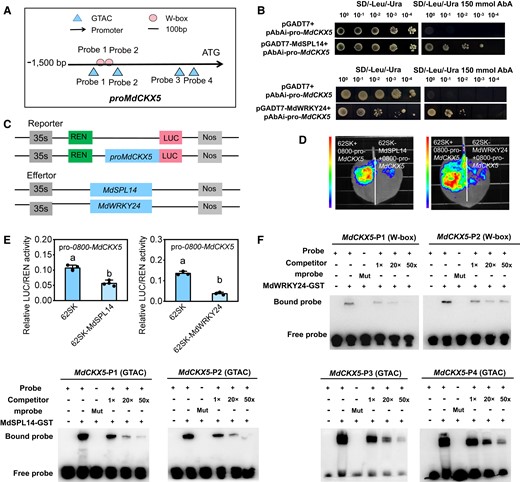
MdSPL14 and MdWRKY24 directly repress the expression of MdCKX5. A)MdCKX5 promoter sequence analysis reveals the presence of GTAC and W-box-binding elements. B) Y1H assay indicating that MdSPL14 and MdWRKY24 can bind to the MdCKX5 promoter in yeast cells. C) Schematic diagram of the constructed reporter and effector vectors. D, E) LUC assay indicating that MdSPL14 and MdWRKY24 inhibit the MdCKX5 promoter activity in N. benthamiana, respectively. Data are means ± Sd (n = 3). Values with different letters are significantly different according to 1-way ANOVA followed by Tukey's test (P < 0.05). F) EMSA confirming that MdSPL14 and MdWRKY24 bind to the MdCKX5 promoter in vitro, respectively. The probe was a biotin-labeled fragment of the MdCKX5 promoter, whereas the competitor probe was an unlabeled probe (10-, 50-, and 50-fold molar excess). The “+” represents the presence of relevant probes or proteins, and “-” represents the absence of relevant probes or proteins. “Mut” represents mutant probes with core sequences.
To determine the effect of the MdSPL14–MdWRKY24 interaction on the transcription of MdCKX5, we performed an EMSA using MdSPL14-GST and MdWRKY24-GST fusion proteins. The addition of MdSPL14 and MdWRKY24 protein substantially increased the brightness of the bands of the probe bound to the interacting protein when DNA fragments containing the MdSPL14- and MdWRKY24-binding site in the MdCKX5 promoter were used as probes, respectively (Fig. 6, A and B), indicating that the interaction between MdSPL14 and MdWRK24 enhances binding to the MdCKX5 promoter. At the same time, LUC assays in apple calli confirmed that the MdSPL14–MdWRKY24 interaction enhanced the repression of MdSPL14 and MdWRKY24 on MdCKX5 (Fig. 6, C and D). These observations demonstrated that the MdSPL14 and MdWRKY24 coordinately inhibit the MdCKX5 transcription.
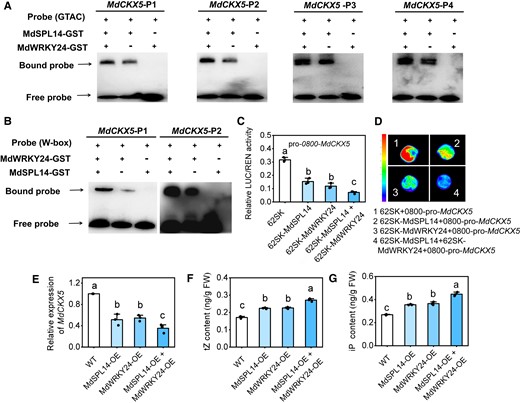
MdSPL14 and MdWRKY24 act coordinately to regulate CK levels by repressing MdCKX5 expression. A, B) EMSA indicating that the addition of MdSPL14 and MdWRKY24 enhances binding to biotin-labeled oligonucleotides of MdCKX5, respectively. C) LUC assay indicating that the coexpression of MdSPL14 and MdWRKY24 enhances the transcriptional repression activity of MdSPL14 and MdWRKY24 on MdCKX5 in apple calli. D) Relative LUC/REN activity of apple calli coexpressing MdSPL14, MdWRKY24, and proMdCKX5. Data are means ± Sd (n = 3). E to G) The relative expression levels of MdCKX5, and tZ and iP content in response to MdSPL14-OE, MdWRKY24-OE, and MdSPL14-OE + MdWRKY24-OE via agroinfiltration to leaves, with empty vector as control. WT, wild type; OE, overexpressing; FW, fresh weight. Data are means ± Sd (n = 3). Values with different letters are significantly different according to 1-way ANOVA followed by Tukey's test (P < 0.05).
To determine whether the mdm-miR156a–MdSPL14–MdWRKY24 module mediated the transcriptional repression of MdCKX5, which controls endogenous CK levels, we overexpressed MdSPL14, MdWRKY24, and both MdSPL14 and MdWRKY24 in apple leaves via transient infiltration. This increased the transcription of MdSPL14 and MdWRKY24 to a significantly higher level in MdSPL14-OE and MdWRKY24-OE leaves than in the empty vector control, which significantly reduced the expression of MdCKX5 (Supplementary Fig. S12; Fig. 6E). The tZ and iP content was higher in leaves with MdSPL14 and MdWRKY24 both overexpressed than in leaves with only MdSPL14 or MdWRKY24 overexpressed (Fig. 6, F and G), indicating that the overexpression of MdSPL14 and MdWRKY24 positively regulates endogenous CK levels by repressing MdCKX5 expression.
MdCKX5 negatively regulates leaf size by altering endogenous CK levels
To explore the physiological function of MdCKX5 in apple, 3 MdCKX5-overexpressing lines (#1, #7, and #11) were generated in Arabidopsis (Supplementary Fig. S13, A and B). Overexpression of MdCKX5 under control of the 35S-CaMV promoter (35S: GFP-MdCKX5) resulted in dwarfism, small leaf size, and delayed flowering (Supplementary Fig. S13, A to D). Given that leaf size is largely determined by the rate and patterns of cell division (Wang et al. 2022a), we observed epidermal cell size in fully developed rosette leaves. This result revealed a strongly reduced number of the 35S: MdCKX5 line epidermal cells, which were larger compared with those in WT plants (Supplementary Fig. S13, E to G). This indicates that MdCKX5 plays an essential role in regulating cell expansion. We then determined the level of endogenous CK in 35S: MdCKX5 line. The tZ content was much lower in 35S: MdCKX5 lines than in WT plants (Supplementary Fig. S13H), indicating that the 35S: MdCKX5 leaf phenotype might stem from the reduction in CK levels.
Next, we examined whether the altered leaf size was attributed to changes in CK levels. CKs (tZ-type) are mainly synthesized in the roots and can be transported to the aboveground plant organs via a long-distance transport process (Kiba et al. 2013; Zhao et al. 2021). As previously mentioned, MdCKX5 was mainly expressed in apple roots; we thus generated MdCKX5-overexpressing apple lines under a 5y plant background and MdCKX5-RNAi apple lines under a 1y plant background using Agrobacterium rhizogenes-mediated transformation technology (Supplementary Fig. S14, A and B). The MdCKX5-RNAi-1y plants had larger leaves compared with 1y plants, but the MdCKX5-OE-5y plants had smaller leaves compared with 5y plants (Fig. 7, A and B), indicating that the phenotype of MdCKX5-OE-5y plants can be partially restored to that of 1y plants; the tZ content was significantly higher in MdCKX5-RNAi-1y leaves than in 1y plants, but lower in MdCKX5-OE-5y plants than in 5y plants (Fig. 7C), indicating that leaf size variation in MdCKX5-OE/RNAi apple plants was caused by endogenous CK levels. Microscopic analysis showed that epidermal cells were larger in MdCKX5-OE-5y apple plants compared with 5y plants, but smaller in MdCKX5-RNAi-1y apple plants compared with 1y plants (Fig. 7D); the number of epidermal cells was significantly lower in MdCKX5-OE-5y apple plants compared with 5y plants, but significantly higher in MdCKX5-RNAi-1y apple plants compared with 1y plants (Fig. 7, E and F), which was consistent with previous observation of the epidermal cells of 1y, 3y, and 5y plants (Fig. 1D).
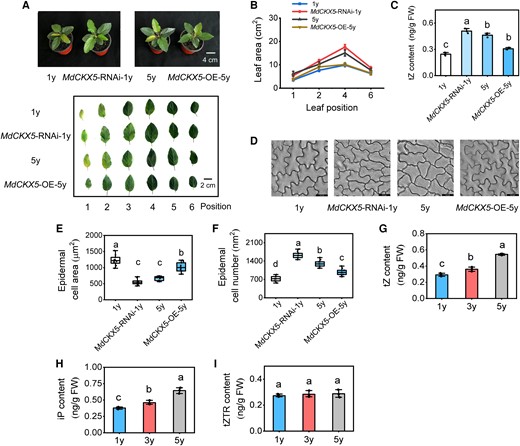
MdCKX5-RNAi and MdCKX5 overexpression alter leaf size in apple. A) The phenotypes of 3-mo-old MdCKX5-OE/RNAi apple plants. Images were digitally extracted for comparison. B) Leaf area. C) Endogenous tZ content in leaves. D) Microscopic observation of adaxial epidermal cells. Scale bar = 23.2 μm. E, F) The area and number of adaxial epidermal cells. Data are means ± Sd (n = 10). G to I) Endogenous tZ, iP, and zeatin riboside (tZTR) content in leaves (the fully expanded leaves at 5 positions) in 1y, 3y, and 5y tissue-cultured apple plants. FW, fresh weight. Center line, median; box limits, upper and lower quartiles; whiskers, 1.5× interquartile range; points, outliers. Data are means ± Sd (n = 3). Values with different letters are significantly different according to 1-way ANOVA followed by Tukey's test (P < 0.05).
We also tested whether the exogenous application of CK (6-benzyladenine [6-BA]) affects leaf size in 1y plants. Indeed, 6-BA treatment significantly increased the leaf size in 1y plants (Supplementary Fig. S15, A and B), demonstrating that CK signaling has a major effect on leaf morphology in the juvenile stage. To further confirm the role of CK in determining leaf size during the vegetative phase transition in apple, we determined CK levels in 1y, 3y, and 5y plants. The tZ and iP content in the leaves gradually increased with age, but the tZTR content remained unchanged (Fig. 7, G to I), indicating that CK is a positive regulator of vegetative phase change, which was consistent with the previous finding that 1y plants had smaller leaves than 5y plants when MdCKX5 was highly expressed in 1y plants. Overall, these results indicated that MdCKX5 modulates leaf size by controlling CK levels in apple.
The mdm-miR156a–MdSPL14 module is involved in the CK-dependent regulation of leaf size in apple
To further characterize the role of the mdm-miR156a–MdSPL14 module in the CK-dependent regulation of leaf size, we generated mdm-miR156a- and MdSPL14-overexpressing apple plants under a 1-yr-old plant background using A. rhizogenes-mediated transformation technology (Supplementary Fig. S16A). RT-qPCR analysis showed that MdCKX5 expression increased in mdm-miR156a-OE plants but decreased in MdSPL14-OE plants (Fig. 8B), further confirming that the mdm-miR156a–MdSPL14 module acts upstream of MdCKX5. Moreover, we observed that mdm-miR156a-OE plants had smaller leaves, and the MdSPL14-OE plants had larger leaves compared with 1y plants (Fig. 8, A and C); the tZ content was significantly lower in mdm-miR156a-OE plants but higher in MdSPL14-OE plants than in 1y plants (Fig. 8D), indicating that mdm-miR156a–MdSPL14 module is involved in the CK-dependent regulation of leaf size by altering the abundance of MdCKX5 transcripts. Consistent with previous observation of the epidermal cells of MdCKX5-OE/RNAi apple plants (Fig. 7), the epidermal cells were larger in mdm-miR156a-OE plants but smaller in MdSPL14-OE plants compared with 1y plants (Fig. 8E; Supplementary Fig. S16B); the number of epidermal cells was significantly lower in mdm-miR156a-OE plants and higher in MdSPL14-OE plants compared with 1y plants (Fig. 8F). Overall, these results demonstrated that the mdm-miR156a–MdSPL14 module plays an important role in the regulation of leaf size in apple.
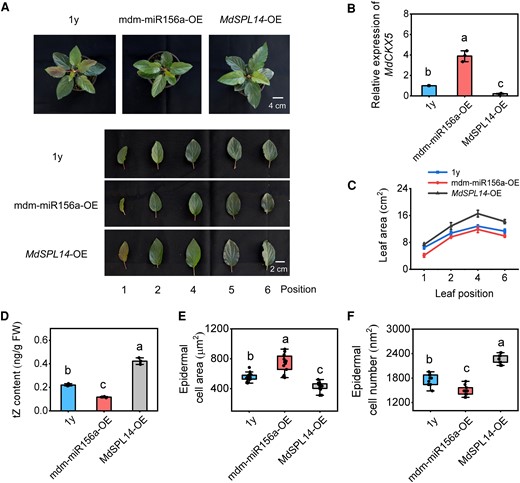
Mdm-miR156a and MdSPL14 are involved in the CK-dependent regulation of leaf size in apple. A) The phenotypes of mdm-miR156a-OE/MdSPL14-OE apple plants in the background of 1y plants. OE, overexpressing. B) Relative expression levels of MdCKX5 in roots. C) Leaf area. D) Endogenous tZ content in leaves. FW, fresh weight. Data are means ± Sd (n = 3). E, F) The area and number of adaxial epidermal cells. Data are means ± Sd (n = 10). Values with different letters are significantly different according to 1-way ANOVA followed by Tukey's test (P < 0.05).
MdSPL14 and MdWRKY24 act coordinately to repress MdARR6 expression
Although the above findings support the hypothesis that CK regulates leaf size by affecting cell division and cell expansion, the role of CK signaling in cell growth remains unknown. We further analyzed the RNA-seq data and identified 3 type-A response regulator genes (MdARRs) involved in the CK signal transduction pathway (ko04075). MdARR6, MdARR3, and MdRR10 all showed age-dependent expression patterns in apple (Supplementary Fig. S17, A to C); these genes were highly expressed in 1y plants, and their expression gradually decreased with age, suggesting that these genes play a negative role in regulating the vegetative phase change in apple. MdARR6 was used in subsequent experiments because its expression pattern was the most distinct among all 3 of these genes. RT-qPCR analysis revealed that MdARR6 was expressed in all tissues; the expression of MdARR6 was highest in apple roots, followed by the leaves (Supplementary Fig. S17D). We next analyzed MdARR6 expression in the leaves and roots of seedlings under treatment with tZ and iP, respectively, and found that the expression of MdARR6 was strongly downregulated by CK treatment (Supplementary Fig. S17, E and F), suggesting that MdARR6 is a functionally negative regulator of CK signaling in apple.
A previous study revealed that Arabidopsis SPL9 functions as a direct transcriptional repressor of ARR genes and regulates the shoot regenerative capacity by mediating the CK response (Zhang et al. 2015). To investigate whether MdARR6 transcription is regulated by the mdm-miR156a–MdSPL14–MdWRKY24 module, we performed Y1H, EMSA, and LUC assays. These assays revealed that MdSPL14 and MdWRKY24 could specifically bind to the MdARR6 promoter, thereby inhibiting the transcriptional activity of MdARR6 (Fig. 9, A to F; Supplementary Fig. S18). To determine the effect of the MdSPL14–MdWRKY24 interaction on the transcriptional regulation of MdARR6, we performed an EMSA using MdSPL14-GST and MdWRKY24-GST fusion proteins and different probes containing the MdSPL14-binding site and W-box-binding site in the MdARR6 promoter. The addition of MdSPL14 and MdWRKY24 protein substantially increased the brightness of the bands of the probe bound to the interacting protein (Fig. 9G). A LUC assay further demonstrated that the MdSPL14–MdWRKY24 interaction enhanced the repression of MdSPL14 and MdWRKY24 on MdARR6 expression in apple calli (Fig. 9H). Overall, these results demonstrated that MdSPL14 and MdWRKY24 act coordinately to directly regulate MdARR6 transcription.
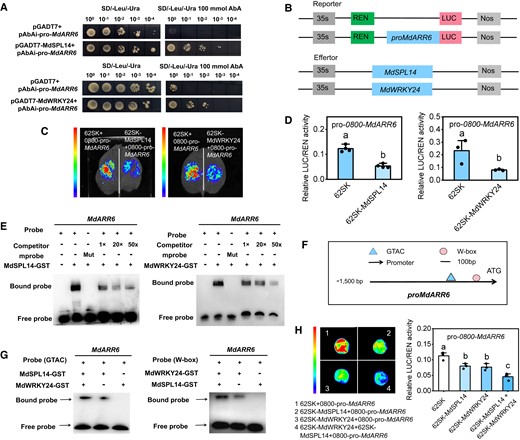
MdSPL14–MdWRKY24 module directly represses MdARR6 expression. A) Y1H assay showing that MdSPL14 and MdWRKY24 can bind to the MdARR6 promoter in yeast cells, respectively. B) Schematic diagram of the constructed reporter and effector vectors. C, D) LUC assay indicating that MdSPL14 and MdWRKY24 inhibit MdARR6 promoter activity in N. benthamiana, respectively. Data are means ± Sd (n = 3). Values with different letters are significantly different according to 1-way ANOVA followed by Tukey's test (P < 0.05). E) EMSA assay confirming that MdSPL14 and MdWRKY24 bind to the MdCKX5 promoter in vitro, respectively. F)MdARR6 promoter sequence analysis reveals the presence of GTAC and W-box-binding elements. G) EMSA assay showing that the addition of MdSPL14 and MdWRKY24 enhances binding to the biotin-labeled oligonucleotides of MdARR6, respectively. H) LUC assay showing that the coexpression of MdSPL14 and MdWRKY24 enhances inhibition of the MdARR6 promoter in apple calli, respectively.
Silencing of MdARR6 alters leaf size and enhances CK responses
To investigate the function of MdARR6 in leaf development, we generated 3 independent MdARR6-overexpression lines in Arabidopsis and obtained RNAi apple lines in the background of 1-yr-old plants using virus-induced gene silencing (VIGS). MdARR6-overexpressing Arabidopsis lines (#1, #2, and #3) exhibited delayed development compared with WT plants (Supplementary Fig. S19, A to C). We observed epidermal cells in fully developed MdARR6-overexpressing lines with rosette leaves and found that the shape of MdARR6-overexpressing cells was more convoluted than that of WT plants; the cells of MdARR6-overexpressing plants were larger and had more indentations than those of WT plants (Supplementary Fig. S19D). The number of epidermal cells was significantly lower in MdARR6-overexpressing lines than in WT plants, and the epidermal cell area was significantly higher in MdARR6-overexpressing lines than in WT plants (Supplementary Fig. S19, E and F). These results indicate that cell proliferation was delayed due to the promotion of cell expansion.
Apple seedlings with MdARR6 silenced in 1y plants via VIGS for 35 d had larger leaves compared with 5y plants (Fig. 10, A to C). Microscopic analysis revealed that TRV-MdARR6-1y plants had smaller cells compared with TRV-1y plants (Fig. 10D), indicating that MdARR6 is a negative regulator of cell proliferation. The number of epidermal cells was significantly higher in TRV-MdARR6-1y plants compared with TRV-1y plants, and the epidermal cell area was significantly lower in TRV-MdARR6-1y plants compared with TRV-1y plants (Fig. 10, E and F). These results indicated that silencing MdARR6 alters the leaf size in 1y plants. In contrast, the number of epidermal cells in TRV-MdARR6-1y was slightly lower compared with that of 5y plants, and the epidermal cell area did not significantly differ between TRV-MdARR6-1y and 5y plants (Fig. 10, E and F), indicating that the larger-leaf phenotype of 5y plants possibly stems from the reduced transcription of MdARR6.
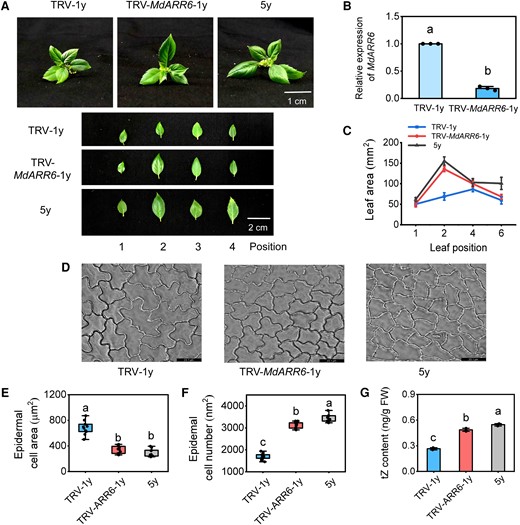
MdARR6 is involved in the CK-dependent regulation of leaf size in apple. A) The phenotype difference between TRV-MdARR6 plants and TRV plants after 30 d. TRV, tobacco rattle virus. Scale bars = 1 cm. B) Relative expression levels of MdARR6 in leaves. C) The leaf area. D) Microscopic observation of adaxial epidermal cells. Scale bar = 23.1 μm. E, F) The area and number of adaxial epidermal cells. Data are means ± Sd (n = 10). G) Endogenous tZ content in leaves. FW, fresh weight. Center line, median; box limits, upper and lower quartiles; whiskers, 1.5× interquartile range; points, outliers. Data are means ± Sd (n = 3). Values with different letters are significantly different according to 1-way ANOVA followed by Tukey's test (P < 0.05).
The tZ level was significantly lower in MdARR6-overexpressing lines than in WT plants (Supplementary Fig. S19G), but significantly higher in TRV-MdARR6-1y plants than in TRV-1y plants (Fig. 10G), indicating that MdARR6 controls the CK level via a negative feedback mechanism. Given that MdARR6 is mainly expressed in the roots and leaves (Supplementary Fig. S17D), we generated MdARR6-overexpressing apple lines using A. rhizogenes-mediated transformation technology to obtain additional evidence that MdARR6 is a negative feedback regulator of the CK signaling pathway in apple. The tZ and iP content was significantly lower in MdARR6-OE apple plants than in WT apple plants (Supplementary Fig. S20, A to D). Overall, these results demonstrated that MdARR6 negatively regulates leaf size in apple by controlling the transition between cell expansion and proliferation.
Discussion
Vegetative phase transition is a complex process that is mainly driven by age in perennial woody plants (Rankenberg et al. 2021). The transitions are characterized by pronounced changes in leaf morphology, photosynthetic traits, and growth strategies (Poethig 2010; Lawrence et al. 2021; Lawrence-Paul et al. 2023). Although these age-dependent changes are strongly associated with the capacity of plants to cope with various environmental stresses, the regulatory mechanisms remain unknown in apple because of limitations of the long juvenile phase. In this study, we provided morphological evidence that vegetative phase change-related traits including leaf size, trichome density, and stomata density in the source tree could be maintained in tissue-cultured apple plants after tissue culture. Tissue culture should be controlled over 2 generations to avoid rejuvenation. We further provided detailed mechanistic evidence that the age-mediated mdm-miR156a–MdSPL14–MdWRKY24 module integrates CK signaling to control changes in leaf size during the juvenile-to-adult phase transition. These findings provide critical insights into the age-dependent changes in tissue-cultured apple plants, which enhance our understanding of the vegetative phase transition in apple and provide important materials for future studies of the effects of biotic stress.
The age-mediated mdm-miR156a–MdSPL14–MdWRKY24 regulatory module is involved in CK homeostasis and signaling responses
The miR156–SPL module is a conserved age-dependent pathway that controls the juvenile-to-adult transition in diverse plants (Wu et al. 2009; Teotia and Tang 2015; Wang and Wang 2015). Recent studies have revealed the importance of crosstalk between the miR156–SPL pathway and hormones in controlling phase transitions (Werner et al. 2021; Guo et al. 2021a; Zhou et al. 2023). For example, the BR biosynthesis gene, DWARF5 (DWF5), is a rate-limiting enzyme in the BR biosynthetic pathway that modulates the juvenile-to-adult phase transition through the SPL9-dependent pathway in Arabidopsis (Zhou et al. 2023). However, the molecular mechanisms underlying the vegetative phase transition that induce age-dependent changes among tissue-cultured apple plants at different age remain unclear. In this study, we showed that MdSPL14 physically interacts with MdWRKY24 to synergistically repress the expression of the downstream target genes MdCKX5 and MdARR6 involved in CK homeostasis and signaling responses (Fig. 11). The transcript level of MdSPL14, which is the target of mdm-miR156a, did not differ among 1y, 3y, and 5y plants when these plants were grown to late stages (Supplementary Fig. S7). This discrepancy might be attributed to the fact that the abundance of MdSPL14 in old tissue-cultured apple plants is greater than the level of MdSPL14 released by mdm-miR156a (Xu et al. 2016). Another possibility is that MdSPL14 expression can be mediated by proteins encoded by other age-targeted genes such as different SPL genes that are functionally redundant with MdSPL14, nuclear factor Y (NF-Y) proteins, or WRKY transcription factors (Teotia and Tang 2015; Ma et al. 2020; Zhou et al. 2023).
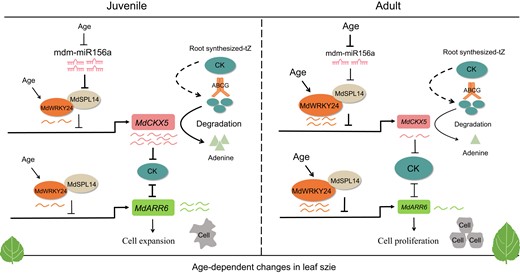
A model showing the molecular mechanism of mdm-miR156a–MdSPL14–MdWRKY24 module integrates CK to regulate the age-dependent changes in leaf size during the vegetative phase change in apple. CK, cytokinin; tZ, trans-zeatin; ABCG, ATP-binding cassette G. The larger the font, the higher the expression level. Black arrows indicate promotion, while black blunts represent inhibition. The thickness of black arrows indicates the strength of the effect of inhibition. Wavy line indicates the expression levels of genes; the more the line, the higher the expression level. The curved arrow represents the degradation of CK by MdCKX5. The dashed arrow represents the transport from the roots to leaves, which remains to be further experimentally confirmed.
In Arabidopsis, WRKY12 and its homolog WRKY13 regulate the GA-mediated flowering pathway by directly interacting with DELLA proteins (Li et al. 2016); WRKY12 and WRKY13 are involved in the age-mediated juvenile-to-adult phase transition by physically interacting with SPL10 and coregulating the expression of the target gene miR172b (Ma et al. 2020). Our study revealed that the age-mediated MdWRKY24 enhances the transcriptional repression activity of MdSPL14 on MdCKX5 and MdARR6, indicating that MdWRKY24 plays a crucial role in mediating age-dependent changes in apple. CKX proteins irreversibly degrade active CKs, thereby maintaining CK homeostasis in plants (Wang et al. 2022a; Geng et al. 2023). The overexpression of MdSPL14 and MdWRKY24 in apple leaves decreased MdCKX5 expression and promoted CK accumulation; cooverexpression of these genes increased CK levels (Fig. 6, E to G), which indicates that the interaction between MdSPL14 and MdWRKY24 reduces the degradation of CK. These results revealed that the MdSPL14–MdWRKY24 interaction is critical for the function of MdSPL14 in the CK-dependent regulation of age-dependent changes in apple.
MdCKX5 is involved in the age-dependent regulation of changes in leaf size by controlling CK levels in apple.
CK has been linked to many aspects of plant development including shoot regeneration, leaf blade development, and root primordium formation (Zhang and Yuan 2014; Wang et al. 2022a; Geng et al. 2023). For example, CK activates the expression of type-B ARR genes to regulate shoot regeneration in Arabidopsis (Meng et al. 2017; Liu et al. 2020). The protein encoded by the CK-responsive gene MdTCP17 interacts with MdWOX11 to repress adventitious root primordium formation by altering CK signaling in apple rootstocks (Mao et al. 2023). OsCKX3 controls lamina joint development and negatively regulates the leaf angle by mediating CK homeostasis in rice (Huang et al. 2023). In this study, we demonstrated that MdCKX5 acts downstream of the mdm-miR156a–MdSPL14–MdWRKY24 module to regulate leaf size by controlling CK levels (Figs. 5 and 7). Previous studies have shown that miR156-targeted SPLs are master regulators of leaf trait development during the vegetative phase change (Poethig 2013; Wang and Wang 2015; Yu et al. 2015), and elevated SPL10 levels are required to promote anisotropic cell growth and prolong the cell proliferation phase during leaf blade morphogenesis in Arabidopsis (Tang et al. 2023). We thus speculate that variation in leaf size during the vegetative phase change was mainly attributable to changes in CK levels due to the degradation of MdCKX5.
This hypothesis was further confirmed by the finding that the overexpression of MdCKX5 in both Arabidopsis and apple enhanced degradation to CK, which resulted in the smaller leaves of MdCKX5-overexpressing plants compared with WT plants, and the silencing of MdCKX5 by RNAi in apple roots resulted in larger leaves due to CK accumulation (Supplementary Fig. S13; Fig. 7). Similarly, the overexpression of NsCKX3 in Medicago truncatula and Nicotiana sylvestris accelerated the degradation of CK and resulted in narrower and shorter leaves with fewer cells (Wang et al. 2022a), indicating that CK controls leaf development by regulating cell proliferation and differentiation (Shani et al. 2010; Meng et al. 2017). Reductions in CK accumulation or signaling contribute to smaller leaves, confirming that CK plays a critical role in determining final leaf size (Nishimura et al. 2004; Muszynski et al. 2020). These results demonstrated that CK levels are mediated by the mdm-miR156a–MdSPL14–MdWRKY24–MdCKX5 pathway and are closely associated with leaf size in apple.
CK signaling-mediated type-A MdARR6 regulates age-dependent change in leaf size in apple
Characterizing how CK signaling affects cell growth zones is important for determining final leaf size in the CK-dependent juvenile-to-adult phase transition in apple. In Arabidopsis, the well-known type-A ARRs are negative feedback regulators of the CK signaling pathway that can be rapidly induced by CK treatment (To et al. 2004; Liu et al. 2020), and they play essential roles in mediating shoot regeneration, leaf senescence, and vegetative phase transitions (Duan et al. 2019; Acheampong et al. 2020). Type-A ARRs were specifically expressed in the expanding leaves, which contributed to the repression of CK responses and cell proliferation (Efroni et al. 2013). The ZmRR3 signal in the margins of early-stage leaf primordia was observed when CK signaling was upregulated in maize, and ZmRR3 normally functions by altering leaf phyllotaxy (Muszynski et al. 2020), confirming that type-A ARRs play critical roles in controlling leaf marginal morphogenetic activity (Shani et al. 2010). These results support the notion that type-A ARRs might act downstream of CK to directly regulate leaf development.
In this study, we showed that overexpression of MdARR6 in Arabidopsis delayed epidermal cell proliferation relative to that in WT plants, which altered leaf development (Supplementary Fig. S19); the phenotype observed is typical under CK deficiency (Guo et al. 2021b). Moreover, the level of tZ was reduced in MdARR6-overexpressing Arabidopsis plants and slightly elevated when the expression of MdARR6 was reduced by VIGS (Supplementary Fig. S19H; Fig. 10G), confirming that MdARR6 negatively regulates CK levels via a feedback loop, thereby weakening the CK response. Consistent with this, VIGS-induced gene silencing of SlWRKY44/76, SlbHLH079, SlIAA, and SlGA20 in tomato alters leaf morphology (Zhu et al. 2023), and this alteration is determined by cell proliferation, differentiation, and homeostasis (Wang et al. 2022a). Our observations of epidermal cells in MdARR6-overexpressing Arabidopsis lines and TRV-MdARR6 apple plants revealed that MdARR6 accelerates leaf expansion and represses cell proliferation (Supplementary Fig. S19; Fig. 10). Molecular evidence demonstrated that the age-mediated mdm-miR156a–MdSPL14–MdWRKY24 module directly represses MdARR6 expression and thus reduces CK responses (Fig. 9). These results demonstrated that the mdm-miR156a–MdSPL14–MdWRKY24 pathway integrates CK signals to regulate age-dependent changes in leaf size during the vegetative phase transition in apple.
Based on these findings, we propose a regulatory model for age-dependent changes in leaf size during the vegetative phase change in apple (Fig. 11). In juvenile stage, the high expression of mdm-miR156a results in a low level of MdSPL14 to alleviate the suppression of MdCKX5 by MdSPL14, which leads to the overaccumulation of MdCKX5 and maintains low CK levels. During the transition from the juvenile to the adult stage, the level of mdm-miR156a decreases, and MdWRKY24 expression increases, which promotes the MdSPL14–MdWRKY24 interaction, strengthens the suppression of MdCKX5 by the MdSPL14–MdWRKY24 module, reduces the degradation of MdCKX5 to CK, and increases CK signaling; however, a high level of CK suppresses MdARR6 expression, thereby promoting cell proliferation. The mdm-miR156a–MdSPL14–MdWRKY24 module also enhances the repression of MdARR6 to precisely regulate CK responses. Overall, these results provide important insights into changes in leaf size during the vegetative phase change in apple.
Materials and methods
Plant materials and growth conditions
In 2015, 224 seeds of the F1 progeny of “Ambrosia (maternal parent/apple [M. domestica]) × Honeycrisp (paternal parent/apple)” were germinated in a nutrient bowl that contained a local 4:1 (v:v) mixture of loess and sand, and the seedlings were transplanted to a breeding base after growing for 4 mo. In mid-March 2018, we selected 16 plants with branches at the first year and planted them into fields at Northwest A&F University, Yangling (34°20′N, 108°24′E), Shaanxi Province, China. In 2020, 1 apple tree with a short juvenile period was selected from among 16 5-yr-old apple trees and named as the source tree. Specifically, the shoot tips of the juvenile, transition, and adult phases were collected from the 1-, 3-, and 5-yr-old parts of the source tree and regenerated via in vitro tissue culture (Fig. 1A). The regeneration method for stem explants was described by Niu et al (2019). As a result, we obtained tissue-cultured apple plants at different ages and named as 1y, 3y, and 5y, respectively (1-, 3-, and 5-yr-old tissue-cultured apple plants, hereafter referred to as 1y, 3y, and 5y). The 1y represents 1-yr-old tissue-cultured apple plants with juvenile traits, and 5y represents 5-yr-old tissue-cultured apple plants with adult traits. Subsequently, the culture-derived apple plants were transferred to plastic pots (8 × 8 cm) filled with a mixture of organic substrate/perlite/vermiculite (3:1:1) 30 d after rooting and maintained in a light incubator (25 °C, 16/8 h light/dark) for 40 d. After 40 d, the plants were moved into larger plastic pots (30 × 18 cm) with the same weight mixture of soil/organic matter (v:v, 5:1) and placed in a greenhouse to grow.
Morphology and microscopy observation
Four-month-old tissue-cultured apple plants were used for measurements of plant height and nodes. Leaf area was measured using a leaf area scanner (Perfection V19, EPSON, Nagano, Japan). Observations of stomatal and trichomes on the abaxial side of leaves were obtained using a JSM-6360LV scanning electron microscope (SEM; JEOLLtd., Tokyo, Japan). Cross-sections of paraffin-embedded leaves were prepared as described by Yao et al. (2007) and were observed using a light microscope (Olympus BX63, Japan). The adaxial epidermis of leaves was analyzed using a confocal laser scanning microscope (LEICA TCS SP8, Germany) with a 40×/1.4 water objective. The excitation wavelength was performed using a 488 nm solid-state laser, fluorescence was detected at 498 to 540 nm, and the intensity and gain were 4.9% and 800, respectively. Images were postprocessed using the Leica LAS X software (Version 3.7.2) and ImageJ 1.8.0. Stomatal and epidermis density were measured by calculating the number of stomata per unit area. Trichome density and leaf anatomy indexes (leaf thickness, palisade tissue thickness, and upper–lower epidermis thickness) were calculated using ImageJ software. Each leaf was dried at 65 °C until a constant mass was achieved to determine the SLA. SLA represents the amount of area per unit of leaf mass.
RNA extraction and RT-qPCR analysis
Total RNA extraction and cDNA synthesis were performed as previously described by Huo et al. (2020). The cDNA of small RNAs was synthesized using a miRNA 1st Strand cDNA Synthesis Kit (by stem–loop; Vazyme, China). RT-qPCR analysis was conducted using a LightCycler 96 system (Roche, Switzerland). Three biological replicates were performed for each sample. The primers used are listed in Supplementary Table S4.
Transcriptome sequencing
Total RNA was extracted from the fully expanded leaves at 5 positions of 1y, 3y, and 5y plants, and eukaryotic mRNA was enriched using oligo(dT) beads. The mapped reads of each sample were assembled by using StringTie v1.3.1, and fragment per kilobase of transcript per million mapped reads (FPKM) values were calculated to quantify their expression abundance and variations using RSEM software. RNA differential expression analysis was performed using DESeq2 software. mRNAs with a fold change ≥ 2 and P < 0.05 were considered differentially expressed.
Small RNA sequencing
Total RNA was extracted from the fully expanded leaves at 5 positions of 1y, 3y, and 5y plants using the TRIzol reagent kit (Invitrogen, Carlsbad, CA, USA). The clean tags were then searched against the miRBase database (Release 22) to identify known miRNAs (i.e. existing miRNAs). Significantly DEMs were identified using the following criteria: fold change ≥ 2 and P < 0.05. The software PatMatch (Version 1.2) was used to predict target genes. All DEGs were mapped to Gene Ontology (GO) terms in the GO database (http://www.geneontology.org/). Pathway enrichment analysis was conducted using the KEGG database (http://www.genome.jp/kegg).
Subcellular localization
The MdCKX5-GFP fusion protein was transiently expressed in N. benthamiana leaves, and RFP-p24-mCherry (Lerich et al. 2011) was used as an ER location marker. The fluorescence was captured using a confocal laser scanning microscope (LEICA TCS SP8 SR, Germany) with a 40×/1.4 water immersion objective. GFP excitation was performed using a 488 nm solid-state laser, fluorescence was detected at 498 to 540 nm, and the intensity and gain were 4.9% and 800, respectively. mCherry excitation was performed using a 552 nm solid-state laser, fluorescence was detected at 590 to 640 nm, and the intensity and gain were 9.1% and 800, respectively. Pinholes were adjusted to 1 Airy unit for each wavelength. Images were postprocessed using the Leica LAS X software (Version 3.7.2) and ImageJ 1.8.0.
Enzymatic assays of the recombinant protein MdCKX5
The MBP-MdCKX5 fusion protein was expressed in E. coli Rosetta (DE3) and purified using MBP beads (Beyotime, Shanghai, China). MdCKX5 activity was measured according to the method of Zhang et al (2021). The substrates were quantified using HPLC-MS (QTRAP5500; AB SCIEX, Washington, DC, USA).
Exogenous CK treatment
To quantify the transcriptional response of MdCKX5 to CK, 50-d-old seedlings were grown in half-strength Hoagland's nutrient solution. A total of 10 μM tZ and iP was added to the hydroponic culture medium after 14 d. The leaves and roots of seedlings were collected at different time points and immediately transferred into liquid nitrogen until qPCR analysis. For the 6-BA treatment, 45-d-old 1y plants were sprayed with 100 μM 6-BA every 2 d. Water-treated trees served as the controls. After 30 d, the leaves were analyzed.
Measurements of the CK contents
Samples (0.2 g) were extracted using 1.5 mL of mixture containing 2:1:0.002 isopropanol/water/0.1 mol/L HCl and incubated for 24 h at −20 °C under light exposure. The extraction was centrifuged at 4,000 × g for 12 min at 4 °C, and the supernatants were transferred to a clean centrifuge tube with 3 mL of dichloromethane. The mixture was filtered through 0.22-µm organic filters after nitrogen blowing, and CK content was measured using HPLC-MS (QTRAP5500; AB SCIEX, Washington, DC, USA).
Y1H assay
For Y1H assays, the promoter fragments of MdCKX5 (1,500 bp) and MdARR6 (1,500 bp) were cloned into the pHIS2 vector, and the ORFs of MdSPL14 and MdWRKY24 were cloned into the pGADT7 vector. The pGADT7-MdSPL14 and pGADT7-MdWRKY24 vectors were transformed into the Y187 yeast strain with pHIS2-proMdCKX5. The yeast cells were cultured on SD/Ura/Leu/aureobasidin A (AbA) medium for 3 d.
Y2H assay
The MdSPL14 full-length CDS was cloned in pGBKT7, and the MdWRKY24 full-length CDS was cloned in pGADT7. The recombinant plasmids were cotransformed into Y2H Gold (Weidi Biotechnology, Shanghai, China). Positive colonies grown on SD/-Leu/-Trp (DDO) medium were transferred onto selective SD/-Leu/-Trp/-His/-Ade (-QDC) medium.
Dual-luciferase reporter assay
The promoter fragments of MdCKX5 (1,500 bp) were cloned into the pGreenII 0800-LUC double-reporter vector, and the ORFs of MdSPL14 and MdWRKY24 were cloned into the pGreenII62-SK vector. The fusion vectors were coinjected into N. benthamiana leaves with specified combinations by using a needleless syringe. After 48 h, the LUC fluorescence was observed using a Lumazone Pylon 2048B imaging system (Princeton, New Jersey, USA), and the activities were measured using a dual-luciferase reporter gene assay kit (Yeasen, Shanghai, China).
EMSA
GST-MdSPL14 and GST-MdWRKY24 were expressed in E. coli Rosetta (DE3) and purified with GST beads (Beyotime, Shanghai, China). EMSA was performed using a Light Shift Chemiluminescent EMSA Kit according to the manufacturer's instructions (Thermo Scientific, Waltham, MA, USA).
BiFC assay
The full-length CDSs of MdSPL14 and MdWRKY24 were cloned into the pSPYCE-35S and pSPYNE-35S vectors, respectively. The recombinant plasmids were transiently coexpressed in N. benthamiana leaves. The YFP fluorescence was observed under a confocal laser scanning microscope after 2 to 3 d.
Luciferase complementation assay
MdSPL14-NLuc and MdWRKY24-CLuc vectors were constructed. Agrobacterium tumefaciens strain GV3101 (Weidi Biotechnology, Shanghai, China) was then transformed with these 2 vectors, which were also coinjected into N. benthamiana. The fluorescence intensity was measured with an in vivo imaging system (Xenogen, Boston, USA). The primers are listed in Supplementary Table S4.
GST pull-down assays
The full-length CDSs of MdSPL14 and MdWRKY24 were cloned into the pET-32a (with a His tag) and pGEX-4T-1 (with a GST tag) vectors, respectively. The recombinant plasmids were transformed into E. coli (Rosetta strain) to express corresponding His- or GST-fused proteins. Pull-down assays with purified proteins were carried out using a Pierce GST Protein Interaction Pull-Down Kit (Thermo Scientific, Waltham, MA, USA). The proteins were immunoblotted using anti-GST (Beyotime) and anti-His antibodies (Yeasen, Shanghai, China).
Transient transformation assays
Agrobacterium tumefaciens EHA105 that harbored pCambia2300-GFP and mdm-miR156/MdSPL14–MdWRKY24 was used to transiently transform the 1y apple leaves. The bacterial solution and leaves were vacuum infiltrated for 20 min, and the leaves were placed on the culture medium. RT-qPCR identification was performed after 2 to 3 d of transformation.
Vector construction and generation of transgenic materials
The 35S: MdCKX5-GFP, 35S: mdm-miR156a-GFP, and 35S: MdSPL14-GFP fusion vectors were constructed. The principles of RNAi were applied to select a suitable fragment of MdCKX5 and connect it to the pK7WIWG2D vector to obtain the RNAi plasmid. These recombinant plasmids were transformed into A. rhizogenes K599 (Weidi Biotechnology, Shanghai, China) and were obtained transgenic roots as described by Meng et al. (2019).
VIGS
Specific partial sequences of MdARR6 (200 bp) were cloned into separate pTRV2 vectors. The recombinant plasmids were transformed into A. tumefaciens strain EHA105 (Weidi Biotechnology, Shanghai, China). Agrobacterium strain EHA105 contained the resulting vector pTRV2-MdARR6, and pTRV1 was cultured to an OD600 of around 1.0. Whole plants were submerged in infiltration buffer and exposed to vacuum conditions for 15 min. Plants infiltrated with the empty psTRV vectors (psTRV1 + psTRV2) were used as the TRV control plants. After 4 wk, new leaves were collected from the treated plants and immediately frozen in liquid nitrogen. The expression of MdARR6 in each plant was detected by performing an RT-qPCR analysis.
Statistical analyses
Statistical analyses were performed using Origin version 2021 software. All data in this study are shown as the means ± Sd. Statistical significance was determined by 1-way ANOVA. A P < 0.05 was considered statistically significant.
Accession numbers
Sequence data from this article can be found in the GenBank/EMBL data libraries under accession numbers MdCKX5 (XM_008389775.3), MdSPL14 (XM_008393866.3), MdWRKY24 (XM_008386494.3), MdARR6 (XM_008376253), and mdm-miR156a (MDC010428.317).
Acknowledgments
We thank Prof. Jing Zhang (Horticulture Science Research Center, Northwest A&F University, Yangling, China) for technical support.
Author contributions
X.J., C.L., and F.M. conceived and designed the experiments. X.J., S.X., C.Y., and Z.Z. performed the research. Y.W., T.G., L.J., and Y.Q. analyzed the data. X.J. and F.M. wrote the paper.
Supplementary data
The following materials are available in the online version of this article.
Supplementary Figure S1. Leaf morphological changes of source tree at different developmental stages.
Supplementary Figure S2. Multiomics analysis for 1y, 3y, and 5y tissue-cultured apple plants.
Supplementary Figure S3. The GO and KEGG pathway enrichment analysis of DEGs in profile 7.
Supplementary Figure S4. GSEA of all genes in the zeatin biosynthesis pathway.
Supplementary Figure S5. Relative expression levels of MdCKX5 for 1y, 3y, and 5y tissue-cultured apple plants.
Supplementary Figure S6. Relative expression levels of pre-mdm-miR156 for 1y, 3y, and 5y tissue-cultured apple plants.
Supplementary Figure S7. Relative expression levels of mdm-miR156a and MdSPLs at different time points (30, 120, and 210 d) of 1y, 3y, and 5y tissue-cultured apple plants.
Supplementary Figure S8. Schematic diagrams of effector and reporter constructs.
Supplementary Figure S9. Expression patterns of MdWRKY24 for 1y, 3y, and 5y tissue-cultured apple plants.
Supplementary Figure S10. A Y2H assay indicates the interaction of MdSPL14 and MdWRKY24.
Supplementary Figure S11. Mutation data of core sequences of MdCKX5 in EMSA.
Supplementary Figure S12. Agroinfiltration overexpression of MdSPL14, MdWRKY24, and MdSPL14 + MdWRKY24 in apple leaves.
Supplementary Figure S13.MdCKX5 overexpression alters leaf size in Arabidopsis.
Supplementary Figure S14. Identification of MdCKX5 transgenic apple roots.
Supplementary Figure S15. Exogenous 6-benzylaminopurine (6-BA) increased the leaf size in apple plants.
Supplementary Figure S16. Identification of mdm-miR156a and MdSPL14 transgenic apple roots.
Supplementary Figure S17. Expression patterns of MdARR6 for 1y, 3y, and 5y tissue-cultured apple plants.
Supplementary Figure S18. Mutation data of core sequences of MdARR6 in EMSA.
Supplementary Figure S19. MdARR6 is involved in the CK-dependent regulation of leaf size in Arabidopsis.
Supplementary Figure S20. Identification of MdARR6 transgenic apple roots.
Supplementary Table S1. Summary of genome-wide mRNA and miRNA levels in the leaves of 1y, 3y, and 5y tissue culture-derived apple plants.
Supplementary Table S2. The expression pattern of DEGs in profile 0 for 1y, 3y, and 5y tissue-cultured apple plants.
Supplementary Table S3. Summary of mdm-miRNA156 family members in profile 0.
Supplementary Table S4. Primers used in this study.
Funding
This work was supported by the Earmarked Fund for the China Agriculture Research System of MOF and MARA under grant number CARS-27, the National Natural Science Foundation of China under grant number 31972389, the Key S&T Special Projects of Shaanxi Province, under grant number 2020zdzx03-01-02, and the Chinese Universities Scientific Fund (2452023067).
Data availability
The authors confirm that all experimental data are available and accessible via the main text and/or the supplemental data.
Dive Curated Terms
The following phenotypic, genotypic, and functional terms are of significance to the work described in this paper:
References
Author notes
Xumei Jia and Shuo Xu contributed equally.
The author responsible for distribution of materials integral to the findings presented in this article in accordance with the policy described in the Instructions for Authors (https://dbpia.nl.go.kr/plphys/pages/General-Instructions) is: Fengwang Ma ([email protected]).
Conflict of interest statement. The authors declare that they have no conflict of interest.