-
PDF
- Split View
-
Views
-
Cite
Cite
Wei Chang, Huina Zhao, Hongqiao Chen, Guixiang Jiao, Jing Yu, Bing Wang, Haiqian Xia, Boyu Meng, Xiaodong Li, Mengna Yu, Shengting Li, Mingchao Qian, Yonghai Fan, Kai Zhang, Bo Lei, Kun Lu, Transcription factor NtNAC56 regulates jasmonic acid-induced leaf senescence in tobacco, Plant Physiology, Volume 195, Issue 3, July 2024, Pages 1925–1940, https://doi.org/10.1093/plphys/kiae116
- Share Icon Share
Abstract
Leaf senescence is a vital aspect of plant physiology and stress responses and is induced by endogenous factors and environmental cues. The plant-specific NAC (NAM, ATAF1/2, CUC2) transcription factor family influences growth, development, and stress responses in Arabidopsis (Arabidopsis thaliana) and other species. However, the roles of NACs in tobacco (Nicotiana tabacum) leaf senescence are still unclear. Here, we report that NtNAC56 regulates leaf senescence in tobacco. Transgenic plants overexpressing NtNAC56 (NtNAC56-OE) showed induction of senescence-related genes and exhibited early senescence and lower chlorophyll content compared to wild-type (WT) plants and the Ntnac56-19 mutant. In addition, root development and seed germination were inhibited in the NtNAC56-OE lines. Transmission electron microscopy observations accompanied by physiological and biochemical assays revealed that NtNAC56 overexpression triggers chloroplast degradation and reactive oxygen species accumulation in tobacco leaves. Transcriptome analysis demonstrated that NtNAC56 activates leaf senescence-related genes and jasmonic acid (JA) biosynthesis pathway genes. In addition, the JA content of NtNAC56-OE plants was higher than in WT plants, and JA treatment induced NtNAC56 expression. We performed DNA affinity purification sequencing to identify direct targets of NtNAC56, among which we focused on LIPOXYGENASE 5 (NtLOX5), a key gene in JA biosynthesis. A dual-luciferase reporter assay and a yeast one-hybrid assay confirmed that NtNAC56 directly binds to the TTTCTT motif in the NtLOX5 promoter. Our results reveal a mechanism whereby NtNAC56 regulates JA-induced leaf senescence in tobacco and provide a strategy for genetically manipulating leaf senescence and plant growth.
Introduction
Leaves are indispensable photosynthetic organs that provide energy and carbohydrates to sustain plant growth. Leaf senescence is the last stage of leaf development, being often accompanied by leaf yellowing and extensive programmed cell death (PCD) (Kim et al. 2016). During senescence, sequential disorganization of intracellular organelles occurs, along with considerable changes in cell structure, metabolism and gene expression, ultimately resulting in cell death (Buchanan-Wollaston et al. 2005; Lim et al. 2015; Woo et al. 2019). Leaf senescence is controlled by plant age and is strongly induced by adverse environmental conditions (Lee et al. 2012) as well as numerous endogenous cues, such as phytohormones (He et al. 2002; Iqbal et al. 2017; Zhang et al. 2020). In general, cytokinins, auxin, and gibberellic acid (GA) delay leaf senescence, whereas jasmonic acid (JA), salicylic acid, ethylene, abscisic acid (ABA), and strigolactones promote leaf senescence (Jiang et al. 2014; Zhao et al. 2016; Wang et al. 2019).
JA is a lipid-derived phytohormone that mediates plant responses to environmental signals and endogenous signaling pathways. JA influences leaf senescence (Zhuo et al. 2020), stress responses (Yan et al. 2016; Balfagón et al. 2019), and growth–defense trade-offs (Campos et al. 2016; Havko et al. 2016). In various plant species, endogenous JA levels increase in senescing leaves, leading to the activation of JA signaling (Ueda and Kato 1980; Yan et al. 2009; Yang et al. 2021). Therefore, JA is considered a key inducer of leaf senescence (He et al. 2002; Zhu et al. 2015; Hu et al. 2017). JA promotes leaf senescence via two main mechanisms: through regulating genes involved in JA biosynthesis, and via the JASMONATE ZIM-DOMAIN (JAZ)- and MYC (Myelocytomatosis) 2-mediated JA signaling pathway (Chini et al. 2007; Sheard et al. 2010; Zhuo et al. 2020).
JAZ proteins, key repressors of JA signaling, interact with the F-box protein CORONATINE INSENSITIVE 1 (COI1), a component of the Skip-Cullin-F-box (SCF) complex SCFCOI1 (Chini et al. 2007). Upon JA perception, JAZs are degraded by the 26S proteasome, thereby alleviating the transcriptional repression of downstream JA-responsive genes imposed by MYC2, MYC3, and MYC4, three functionally redundant basic helix-loop-helix transcription factors (TFs) that activate JA-induced leaf senescence (Qi et al. 2015; Chini et al. 2016). More specifically, MYC2 binds to the promoter of and activates the expression of SENESCENCE-ASSOCIATED GENE 29 (SAG29) to trigger JA-induced leaf senescence (Qi et al. 2015). In addition, MYC2 targets CATALASE 2 (CAT2) to repress CAT2 expression and promote H2O2 accumulation and JA-induced leaf senescence (Zhang et al. 2020). In Arabidopsis (Arabidopsis thaliana), the expression of JA biosynthesis genes, such as LIPOXYGENASE 1 (LOX1), LOX3, LOX4, and ALLENE OXIDE CYCLASE 1 (AOC1), is upregulated in senescing leaves, accompanied by increased endogenous JA content (He et al. 2002). In particular, these LOX genes regulate chloroplast degradation in senescing plants (Springer et al. 2016).
Within the plant, the physiological and biochemical changes that occur during leaf senescence are accompanied by changes in the expression of many SAG and TF genes, including those encoding basic leucine zipper (bZIP), NAC (NO APICAL MERISTEM [NAM]; Arabidopsis TRANSCRIPTION ACTIVATION FACTOR [ATAF]; CUP-SHAPED COTYLEDON [CUC]), WRKY, MYB, and C2H2-type zinc finger TFs (Kim et al. 2018). DELLA proteins suppress the WRKY6-induced expression of SAG13 and STAY-GREEN 1 (SGR1), a chlorophyll catabolic gene, and negatively regulate dark-induced leaf senescence (Zhang et al. 2018a). The C2H2-type zinc finger TF MdZAT10, is homologous to Arabidopsis STZ/ZAT10 (SALT TOLERANCE ZINC FINGER), which is interacts with BTB-BACK-TAZ2 (MdBT2), a negative regulator of JA signaling, to accelerate JA-induced leaf senescence in apple (Malus domestica) (Yang et al. 2021). In tomato (Solanum lycopersicum), SlMYC2 directly binds to the promoter of SlWRKY37, which is responsible for both JA- and dark-induced leaf senescence (Wang et al. 2022). In Chinese flowering cabbage (Brassica rapa L. ssp. chinensis [L.] Mokino var. utilis Tsen et Lee), BrWRKY75 directly binds to the W-box (TTGAC) in the promoter of its target genes, and the expression of its encoding gene is upregulated in senescent leaves (Tan et al. 2016).
The NAC superfamily of plant-specific TFs comprises 106 members in Arabidopsis, 149 in rice (Oryza sativa) and 154 in tobacco (Nicotiana tabacum) (Gong et al. 2004; Nuruzzaman et al. 2010; Li et al. 2018a). NACs are upregulated by leaf senescence, in which they play an important role (Gregersen and Holm 2007). In tobacco, NtNAC028 plays a key regulatory role in leaf senescence and response to multiple abiotic stresses (Wen et al. 2022). SAG expression is regulated by senescence-related NAC TFs. For instance, overexpression of the NAC TF gene NAC-LIKE, ACTIVATED BY APETALA3 and PISTILLATA (NAP) causes precocious senescence in Arabidopsis (Guo and Gan 2006). The trifurcate feed-forward pathway formed by ETHYLENE INSENSITIVE2 (EIN2), microRNA 164 (miR164), and ORESARA1 (ORE1, also reported as ANAC092) plays an important role in plant senescence and cell death in Arabidopsis (Kim et al. 2009). Moreover, ORE1 represses GOLDEN2-LIKE2 (GLK2) expression and directly activates chlorophyll catabolic genes (CCGs) to accelerate leaf senescence (Rauf et al. 2013; Qiu et al. 2015). In rice, OsNAP positively regulates leaf senescence via the JA pathway (Zhou et al. 2013), and ONAC054 directly activates the expression of ABA INSENSITIVE 5 (OsABI5) during leaf senescence (Sakuraba et al. 2020).
Tobacco is an economically important leaf crop grown globally. Therefore, leaf maturity and yellowing dictate the quality of tobacco products. Studies on leaf senescence have mainly focused on the model plants Arabidopsis and rice, whereas tobacco leaf senescence remains understudied. Here, we explored the regulatory connections between NAC TFs, leaf senescence, and the JA pathway in tobacco. From published transcriptome data, we identified NtNAC56, encoding a positive regulator of leaf senescence. To determine the function of NtNAC56, we generated NtNAC56 overexpression and gene-edited mutant lines and analyzed their leaf senescence phenotypes. We established that NtNAC56 activates the expression of the key JA biosynthesis gene NtLOX5, leading to an increase in endogenous JA content and to premature aging of tobacco leaves. In summary, we reveal that NtNAC56 plays an important role in JA-induced leaf senescence in tobacco.
Results
NtNAC56 promotes premature leaf senescence
Based on previous transcriptome deep sequencing (RNA-seq) analysis of tobacco samples regulating leaf senescence in our laboratory and data from The Arabidopsis Information Resource (TAIR, https://www.arabidopsis.org/), we identified the NAC gene NtNAC56 (mRNA_80414), which is similar to ANAC056 from Arabidopsis (Supplementary Fig. S1). In tobacco, there are 154 nonredundant NAC family proteins (Li et al. 2018a), including four ANAC056 homologs (Supplementary Fig. S1). To study the role of NtNAC56 in leaf senescence, we assessed the senescence phenotypes of transgenic lines overexpressing NtNAC56 under the control of the cauliflower mosaic virus 35S promoter (35S:NtNAC56; NtNAC56-OE) and gene-edited mutant lines (Ntnac56) obtained via clustered regularly interspaced short palindromic repeats (CRISPR)/CRISPR-associated nuclease 9 (Cas9)-mediated genome editing. We measured NtNAC56 expression in independent T3 transgenic overexpression lines by reverse transcription quantitative polymerase chain reaction (RT-qPCR). We chose three transgenic overexpression lines (OE4, OE7, and OE10) for analysis (Supplementary Fig. S2A). We determined that the Ntnac56-19 mutant harbors a 1-bp deletion at the target site (Supplementary Fig. S2B), based on sequencing analysis with the Hi-TOM tool (Liu et al. 2019).
We used the leaves of 8-wk-old tobacco plants for phenotypic observations. Compared to the wild type (WT), leaves turned yellow earlier in NtNAC56-OE lines but much later in the Ntnac56-19 mutant (Fig. 1A). As leaf senescence is usually accompanied by chlorophyll (Chl) degradation (Sakuraba et al. 2014), we determined the Chl content of the third and fifth leaves of all genotypes. The Chl content in OE7 (0.18 ± 0.05 mg/g fresh weight [FW]) and OE10 (0.28 ± 0.07 mg/g FW) leaves was about 30% of that in WT leaves (0.67 ± 0.14 mg/g FW). By contrast, the Chl content of Ntnac56 leaves was not significantly different from that of the WT (Fig. 1B). In agreement with the differences in Chl contents among genotypes, the photosynthetic rate of NtNAC56-OE lines was significantly (P < 0.0001) lower than that of WT and Ntnac56 (Fig. 1C). We also measured leaf water conductance, intercellular CO2 concentration, and transpiration rate. Leaf water conductance and transpiration rate were significantly lower in NtNAC56-OE lines than in the WT and Ntnac56; there was no significant difference in intercellular CO2 concentration among the genotypes (Supplementary Fig. S3). Based on the leaf senescence phenotypes, we predicted that the expression of senescence-related genes would be altered in NtNAC56-OE lines. We, therefore, evaluated the transcript levels of NtSAG12, NtSAG18, NtSAG21, NtSAG101, and SENESCENCE 1 (NtSEN1) by RT-qPCR: we indeed observed significant increases in the expression of these genes in the NtNAC56-OE lines compared to the WT (Fig. 1, D to H). Taken together, these results suggest that NtNAC56 positively regulates leaf senescence.
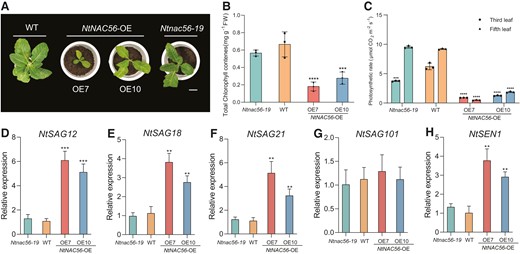
NtNAC56 promotes premature leaf senescence. A) Representative photographs of 8-wk-old WT, Ntnac56-19, and NtNAC56-overexpression (NtNAC56-OE) tobacco plants. Scale bar, 10 cm. FW, fresh weight. Images were digitally extracted for comparison. B) Chlorophyll contents of WT, Ntnac56-19, and NtNAC56-OE tobacco plants. Data are means ± Sd. ***P < 0.001 and ****P < 0.0001, Student's t-test. C) Photosynthetic efficiency from the third and fifth leaves of WT, Ntnac56-19, and NtNAC56-OE tobacco plants. Data are means ± Sd of three replicates. ***P < 0.001 and ****P < 0.0001, Student's t-test. D to H) RT-qPCR analysis of the expression of genes related to senescence in WT, Ntnac56-19 and NtNAC56-OE tobacco plants. Data are means ± Sd of three replicates. **P < 0.01 and ***P < 0.001.
NtNAC56 inhibits root development, seed germination, and plant growth
Leaf senescence is accompanied by restricted growth and development, such as hindered root development and germination rate (Zhuo et al. 2020). Thus, we measured primary root length and plant height in 4-wk-old NtNAC56-OE and WT tobacco plants (Fig. 2A). NtNAC56-OE lines had a shorter stature and shorter primary roots compared to the WT (Fig. 2, B and C), suggesting that plant growth and development are inhibited, which may affect plant biomass accumulation.
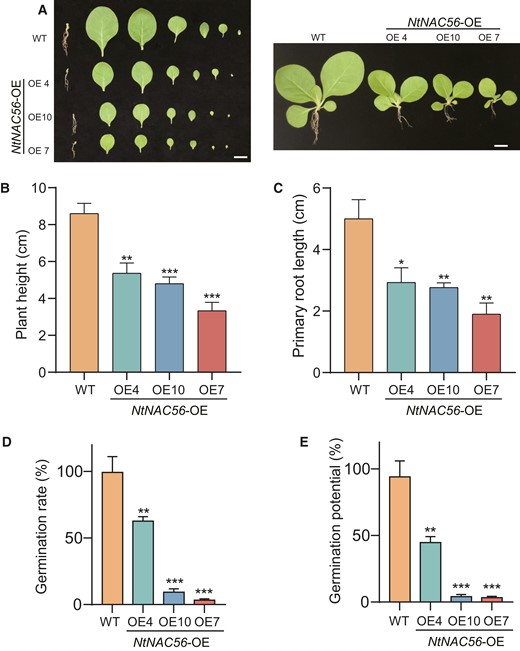
NtNAC56 inhibits root development, seed germination, and plant growth. A) Representative images showing plant height and primary root length of NtNAC56 overexpression lines (NtNAC56-OE) and WT plants. Scale bars, 2 cm. B) Height of the seedlings shown in (A). C) Primary root length. D) Germination rate. E) Germination potential (%). Data are means ± Sd from three replicates in (B) to (E). *P < 0.05; **P < 0.01 and ***P < 0.001, Student's t-test.
Furthermore, the germination rate and germination potential of the NtNAC56-OE lines differed from those in the WT. We recorded the germination potential and germination rate of tobacco seeds over 14 d following initial seed hydration. Compared to the WT, NtNAC56-OE lines exhibited a significantly lower germination rate, and germination potential (P < 0.01 in OE4, P < 0.001 in OE7 and OE10; Fig. 2, D and E). These results suggest that NtNAC56 overexpression impairs plant growth, root length, and seed germination.
NtNAC56 overexpression triggers chloroplast degradation and reactive oxygen species accumulation
Given the lower Chl content and photosynthetic efficiency of NtNAC56-OE lines, we examined chloroplast stability and integrity by transmission electron microscopy (TEM). WT leaf chloroplasts were intact, with highly stacked grana thylakoids. By contrast, many chloroplasts were shrunken in NtNAC56-OE, with blurred granum and lamella, fewer starch granules, and more osmiophilic granules. A few chloroplasts even showed dissolution of the thylakoid membrane (Fig. 3A). These results demonstrate that chloroplasts are degraded more rapidly in NtNAC56-OE than in the WT.
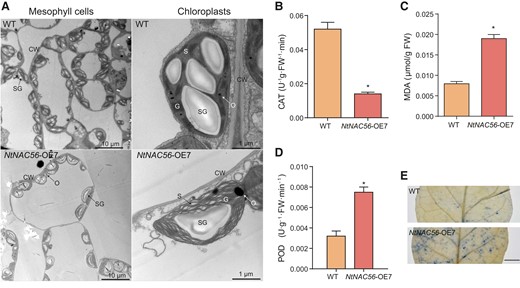
NtNAC56 overexpression triggers chloroplast degradation and ROS accumulation. A) Transmission electron micrographs of leaves from 8-wk-old WT and NtNAC56 overexpression (NtNAC56-OE) plants. Scale bars, 10 μm (left) or 1 μm (right). CW, cell wall; SG, starch granules; G, granum; S, stroma; O, osmiophilic granules. B to D) CAT activity MDA content (C) and POD activity (D) of WT and NtNAC56-OE leaves from 8-wk-old plants. E) TB staining of WT and NtNAC56-OE leaves. Scale bar, 500 μm. For (B) to (D), data are means ± Sd from three replicates. *P < 0.05, Student's t-test. FW, fresh weight.
Reactive oxygen species (ROS) play signaling roles in initiating senescence (Yan et al. 2021). The lipid peroxidation product malondialdehyde (MDA) is an important indicator of oxidative damage. We thus measured catalase (CAT) and peroxidase (POD) activities as well as MDA content (Fig. 3, B to D) and determined that the degree of membrane lipid peroxidation increases in NtNAC56-OE leaf cells, damaging the lipid biofilm structure. Trypan blue (TB) staining showed that NtNAC56-OE leaves contain more dead cells than WT leaves (Fig. 3E). In addition, we investigated the expression of genes encoding ROS-scavenging enzymes, including CAT, glutathione S-transferase (GSTU), glutathione peroxidase (GPX), ascorbate peroxidase (APX), and superoxide dismutase (SOD) in NtNAC56-OE and WT leaves. RT-qPCR analysis showed that NtCAT1, NtGPX2, and NtGSTU8 were induced by NtNAC56 overexpression, while NtAPX1, NtAPX4, and NtSOD2 were repressed (Supplementary Fig. S4). These results suggest that NtNAC56 overexpression causes chloroplast degradation and ROS accumulation in tobacco leaves.
Transcriptome analysis reveals that NtNAC56 activates leaf senescence
To investigate the molecular mechanism underlying NtNAC56-regulated leaf senescence, we performed transcriptome deep-sequencing (RNA-seq) analysis using the leaves of 8-wk-old WT and NtNAC56-OE tobacco plants. We identified 7,917 differentially expressed genes (DEGs), with 4,231 upregulated genes and 3,686 downregulated genes (Fig. 4A). A gene ontology (GO) term enrichment analysis revealed that the upregulated DEGs are involved in “defense response” (GO: 0006952), “response to JA stimulus” (GO: 0009753), “leaf senescence” (GO: 0010150), “immune system process” (GO: 0002376), and “programmed cell death” (GO: 0012501) (Fig. 4B, Supplementary Table S3). Based on these data, we reasoned that the increased JA content in NtNAC56-OE plants might trigger PCD and senescence. The downregulated DEGs were mainly related to “photosynthesis” (GO: 0015979), “carbon fixation” (GO: 0015977), “plastid part” (GO: 0044435), and “metabolic process” (GO: 0008152) (Fig. 4C, Supplementary Table S3).
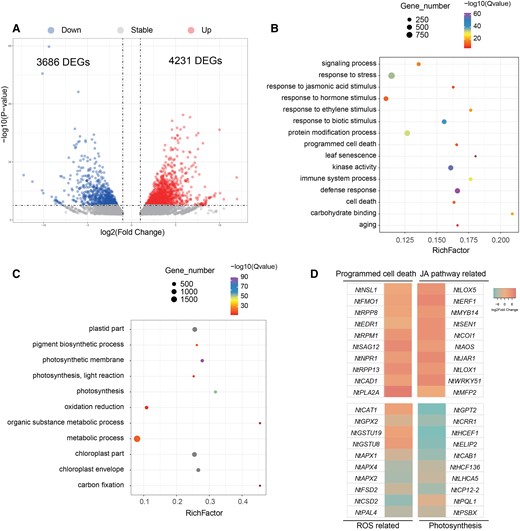
Transcriptome analysis reveals that NtNAC56 activates leaf senescence. A) Volcano plot showing the DEGs in tobacco leaves between WT and NtNAC56-OE lines. P < 0.05 and absolute log2(fold-change) > 1 were used as the significance threshold criteria. B) Top 15 GO terms for biological process, molecular function, and cell component among upregulated genes in NtNAC56-OE lines. C) Top 11 GO terms for biological process, molecular function, and cell component among downregulated genes in NtNAC56-OE lines. D) Heatmap representation of gene expression levels from the RNA-seq data for genes related to PCD, the JA pathway, ROS accumulation, and photosynthesis. The bar in the upper right corner represents log2(fold-change): red indicates high expression and blue indicates low expression.
Consistent with these results, we observed the disintegration of chloroplasts and other organelles in NtNAC56-OE plants, as well as lower photosynthetic capacity and carbon fixation, which also supported the notion that NtNAC56 overexpression triggers senescence. Indeed, we identified many genes related to PCD, JA signaling and biosynthesis pathway, ROS accumulation, and photosynthesis as DEGs in the RNA-seq data (Fig. 4D), several of which showed a consistent pattern with the RT-qPCR results above (Fig. 1, D to H, Supplementary Fig. S4).
JA-inducible NtNAC56 regulates leaf senescence
JA is involved in leaf senescence (Zhuo et al. 2020), and our RNA-seq analysis revealed that upregulated DEGs in NtNAC56-OE lines are associated with response to JA stimulus. To determine whether JA-regulated leaf senescence is mediated by NtNAC56, we treated the WT with 100 μM methyl jasmonate (MeJA) and collected samples at different time points for RT-qPCR analysis. NtNAC56 expression increased upon MeJA treatment (Fig. 5A). Its expression pattern was similar to that of DNA-BINDING WITH ONE FINGER 2.1 (NtDOF2.1) (Fig. 5B), which encodes an enhancer of JA-induced leaf senescence (Zhuo et al. 2020), and opposite that of the photosynthetic marker gene RIBULOSE BISPHOSPHATE CARBOXYLASE SMALL SUBUNIT (NtRBCS) (Fig. 5C).
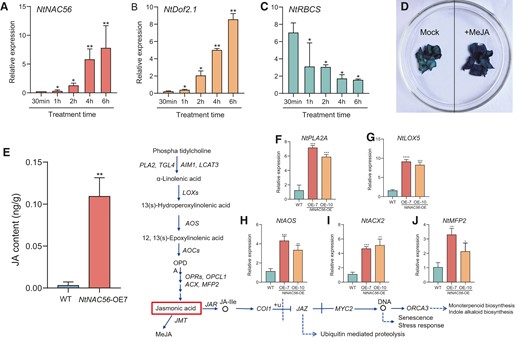
JA-inducible expression of NtNAC56 regulates leaf senescence. A to C) RT-qPCR analysis of NtNAC56 (A), NtDOF2.1 (B), and NtRBCS (C) expression at different time points into MeJA treatment. Data are means ± Sd from three replicates. *P < 0.05 and **P < 0.01, Student's t-test. B) RT-qPCR validation of NtDOF2.1 expression after MeJA treatment at different time points. *P < 0.05 and **P < 0.01, Student's t-test. C) RT-qPCR validation of NtRBCS expression after MeJA treatment at different time points. *P < 0.05, Student's t-test. D) Histochemical analysis of β-GLUCURONIDASE (GUS) activity in NtNAC56 overexpression lines (NtNAC56-OE) tobacco plants harboring the NtNAC56pro:GUS reporter construct. Mock-treated NtNAC56-OE tobacco plants (left) and NtNAC56-OE tobacco plants treated with 100 μM MeJA (right) are shown. E) JA contents in WT and NtNAC56-OE leaves. Data are means ± Sd from three replicates. **P < 0.01, Student's t-test. F to J) RT-qPCR analysis of JA marker gene expression. Data are means ± Sd of three replicates. *P < 0.05; **P < 0.01; ***P < 0.001; ****P < 0.0001, Student's t-test.
To investigate the JA-inducible expression of NtNAC56, we generated transgenic tobacco plants expressing the β-GLUCURONIDASE (GUS) reporter gene under the control of the NtNAC56 promoter (NtNAC56pro:GUS). According to GUS staining assays, MeJA treatment increased GUS activity in NtNAC56pro:GUS leaves compared to mock-treated leaves (Fig. 5D). Moreover, we predicted the presence of multiple elements in the NtNAC56 upstream promoter region by bioinformatic methods (Supplementary Fig. S5A), among which we noticed the presence of MeJA-responsive elements (TGACG-motif and CGTCA-motif, Supplementary Fig. S5B). These results indicate that JA-induced NtNAC56 regulates leaf senescence.
NtNAC56 regulates the expression of JA biosynthesis genes to trigger JA accumulation
To test whether NtNAC56 affects endogenous JA production, we examined JA levels in the leaves of 8-wk-old WT and NtNAC56-OE tobacco plants. The JA content in NtNAC56-OE was 0.11 ± 0.02 mg/g FW, which was significantly (P < 0.01) higher than that of WT leaves (Fig. 5E). Combined with the above data, we hypothesized that JA biosynthesis genes may be targets of NtNAC56. To test this hypothesis, we conducted RT-qPCR analysis for key JA biosynthesis genes. The expression of PHOSPHOLIPASE A 2A (NtPLA2A) (Fig. 5F), NtLOX5 (Fig. 5G), ALLENE OXIDE SYNTHASE (NtAOS) (Fig. 5H), ACYL-CoA OXIDASE 2 (NtACX2) (Fig. 5I), and MULTIFUNCTIONAL PROTEIN 2 (NtMFP2) (Fig. 5J) was induced in NtNAC56-OE lines relative to the WT, consistent with the RNA-seq data and our above hypothesis (Fig. 4D). Thus, NtNAC56 enhances JA accumulation by upregulating JA biosynthesis genes.
NtNAC56 directly binds the promoter of the JA biosynthesis-related gene NtLOX5
Bioinformatics analysis predicted that NtNAC56 contains a nuclear localization signal (Supplementary Fig. S6), suggesting that it may be targeted to the nucleus. We, therefore, constructed the 35S:NtNAC56-YFP (encoding a fusion between NtNAC56 and yellow fluorescent protein) construct and infiltrated it into Nicotiana benthamiana leaf epidermal cells using Agrobacterium (Agrobacterium tumefaciens)-mediated infiltration. Indeed, the NtNAC56-YFP fusion protein localized to the nucleus (Fig. 6A). As NAC TFs often possess transcriptional activity (Sakuraba et al. 2020), we cloned the full-length NtNAC56 coding sequence into the yeast expression vector pGBKT7. Yeast self-activation assays showed that NtNAC56 indeed possesses transactivation activity (Fig. 6B). These results indicate that NtNAC56 functions as a TF.
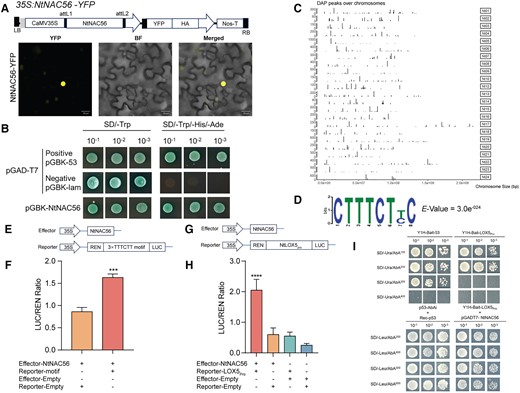
NtNAC56 directly binds to the promoter of a JA biosynthesis-related gene. A) Subcellular localization of NtNAC56. The 35S:NtNAC56-YFP construct was infiltrated into the leaves of N. benthamiana plants. Scale bars, 20 μm. LB, left border. RB, right border. YFP, yellow fluorescent protein. HA, hemagglutinin tag. B) NtNAC56 possesses transcriptional activity in a yeast self-activation assay. SD, synthetic defined medium. C) Positions of DAP peaks over tobacco chromosomes, as determined by DAP-seq analysis. Peak height indicates relative peak density. D) Logo DNA of the core binding motif for NtNAC56. E, G) Diagrams of the constructs used for the dual-luciferase (LUC) system. F) Dual-LUC assay demonstrating that NtNAC56 binds to the 3×TTTCTT motif. Data are means ± Sd of three replicates. ***P < 0.001, Student's t-test. H) Dual-LUC assay showing that NtNAC56 binds to the NtLOX5 promoter. Data are means ± Sd of three replicates. ****P < 0.0001, Student's t-test. I) Y1H assay showing that NtNAC56 binds to the NtLOX5 promoter in yeast cells. AbA, aureobasidin A.
We wished to investigate whether NtNAC56 directly targets downstream genes to regulate tobacco senescence. To this end, we performed DNA affinity purification sequencing (DAP-seq) to predict the target genes of and the motifs targeted by NtNAC56 (Fig. 6C). The average number of total clean sequencing reads was 9,669,500, representing 1,320,930,258 bp, with 94.2% of reads mapping to the reference genome (Supplementary Table S4). The average width of the NtNAC56 binding site was approximately 331.5 bp. We identified 1,300 NtNAC56-binding sites, most of which were centered on the transcription start sites (TSS) of their target genes (Supplementary Fig. S7A). Most of the binding sites were enriched in intergenic regions (c. 75.1%) followed by promoter regions (c. 14.8%), as defined by the sequence up to 2.0 kb upstream from the TSS. In addition, some sites were enriched at intronic (c. 5.08%), transcription termination sites (TTS) (c. 3.46%), and exonic (c. 1.62%) regions (Supplementary Fig. S7B).
Among these NtNAC56 binding sites, we identified six substantially enriched motifs, including RAYTTTCMCCTCACAMGRYTMSWCA (E-value = 3.0e−24), TAGGMA (E-value = 1.5e−02), RRMKACGTATRSRCGAGGTGACG (E-value = 7.7e−013), CTTTCTYC (E-value = 9.2e−06), CAACAAB (E-value = 4.8e−02), and AGTCTGATGAAYTTTCTCCTAGGAT (E-value = 3.1e−36) (Supplementary Fig. S8). Importantly, we noticed the presence of the specific core binding motif, TTTCTY, in all six motifs mentioned above for NtNAC56 binding (Fig. 6D), which is similar to a motif known as the NTM1-LIKE 8 (NTL8)/ANAC040-binding site (NTLBS; TTNCTT) (Lindemose et al. 2014, Yan et al. 2021). To verify that NtNAC56 does bind to TTTCTY, we synthetized a synthetic promoter containing three tandem repeats of the TTTCTT sequence (3× TTTCTT) and placed it upstream of the firefly luciferase (LUC) reporter gene, then tested NtNAC56 binding to this motif in a dual-luciferase reporter (DLR) assay (Fig. 6E). Indeed, we measured higher relative LUC activity when we co-infiltrated the synthetic reporter construct into N. benthamiana leaves together with a NtNAC56 effector construct, in the pGreenII 62-SK backbone, indicating that NtNAC56 directly binds to the 3× TTTCTT motif to activate transcription (Fig. 6F).
To analyze the regulatory relationship among NtNAC56, JA, and leaf senescence, we looked for DEGs in RNA-seq data which containing the TTTCTT motif in their promoters. We identified NtLOX5, encoding a lipoxygenase involved in JA biosynthesis, as one such gene. To test the binding of NtNAC56 to the NtLOX5 promoter, we cloned a 1500-bp NtLOX5 upstream promoter fragment into the LUC reporter vector pGreenII 0800-LUC. We detected high relative LUC activity when the 35S:NtNAC56 effector construct was co-infiltrated with the NtLOX5pro:LUC reporter construct (Fig. 6G). We also performed a yeast one-hybrid (Y1H) assay to validate the NtNAC56–NtLOX5 promoter interaction, which revealed that NtNAC56 can bind to the NtLOX5 promoter (Fig. 6H). These results suggest that NtNAC56 regulates downstream target genes via the TTTCTT cis-regulatory motif and may promote tobacco leaf senescence by binding to the promoter of JA biosynthesis genes and affecting their expression.
Discussion
Senescence is the final stage of development, ending with cell collapse and death. In many plants, senescence begins in the leaves. Leaf senescence research has been the focus of much work combining the analysis of underlying mechanisms and mining of regulatory networks, largely based on the integration of morphological, physiological, and biochemical data (Woo et al. 2019).
The molecular mechanisms associated with leaf senescence have been revealed by identifying SAGs and studying age-related mutants in Arabidopsis (Kim et al. 2018; Zhang et al. 2018a). In NtNAC56-OE lines, SAGs were generally upregulated (Fig. 1, D to H), and the photosynthetic efficiency and physiological and biochemical parameters (Fig. 1, B and C; Supplementary Fig. S3) indicated that NtNAC56 overexpression induces premature senescence. Other aspects of plant growth and development were also affected, such as repressed root development, seed germination, and plant growth (Fig. 2). We plan to investigate the effects of NtNAC56 on other traits in future work, with the aim of elucidating the NtNAC56 regulatory network in tobacco plant growth and development.
Gene expression in plants is tightly controlled by TFs, which often function as molecular switches (Guo and Gan 2006; Woo et al. 2019). Studies have demonstrated that NAC TFs are the core regulators of plant leaf senescence (Guo and Gan 2006; Kim et al. 2009; Lee et al. 2012; Zhou et al. 2013; Zhu et al. 2015; Ma et al. 2018; Yan et al. 2018; Li et al. 2018a; Sakuraba et al. 2020; Yan et al. 2021; Wen et al. 2022). For instance, the NAC TF ATAF1 promotes leaf senescence by upregulating GLK1 and ORE1, which are involved in chlorophyll development and maintenance in Arabidopsis (Garapati et al. 2015). Ma et al. (2018) reported that the ABA-activated NAC TF SlNAP2 regulates leaf senescence and fruit yield in tomato. SlNAP2 directly controls the expression of the senescence-related gene SlSAG113 and the chlorophyll degradation-related genes SlSGR1 and PHEOPHORBIDE A OXYGENASE (SlPAO) to establish ABA homeostasis. In addition to promoting leaf senescence, several NAC family members inhibit leaf senescence, such as VND-INTERACTING 2 (VNI2, also reported as ANAC083) (Yang et al. 2011) and JUNGBRUNNEN 1 (JUB1, also reported as ANAC042) (Wu et al. 2012). Li et al. (2018b) systematically analyzed genetic redundancy among senescence-related NAC TFs and found that most NACs mainly belong to two clusters based on protein sequence similarity. The authors confirmed the genetic redundancy between repetitive NAC TF genes and its minor contribution to leaf senescence regulation. Higher-order knockout mutants need to be generated to verify the roles of these NAC TFs, and also to analyze the leaf senescence phenotypes caused by their interaction with the external environment through some inducible methods.
Although JA has been characterized as a senescence-promoting phytohormone, the mechanisms underlying JA-mediated senescence are not well understood. WRKY57, part of the POWERDRESS (PWR)–HISTONE DEACETYLASE 9 (HDA9)–WRKY53 complex, physically interacts with JAZ4 and JAZ8 and directly represses the expression of SAGs (Jiang et al. 2014). EARLY FLOWERING 3 (ELF3), ELF4, and LUX ARRHYTHMO (LUX) form the Arabidopsis circadian evening complex (EC), which represses diverse SAGs in JA-induced leaf senescence (Zhang et al. 2018a). In our study, the JA content was higher in NtNAC56-OE plants than in the WT (Fig. 5E). JA treatment also induced NtNAC56 expression and participated in leaf senescence (Fig. 5). Moreover, NtNAC56 directly bound to the TTTCTT motif and the NtLOX5 promoter (Fig. 6).
LOXs initiate JA biosynthesis and are widely involved in abiotic stress tolerance (Lim et al. 2015), wounding (Yuan et al. 2023), growth and development (Keereetaweep et al. 2015), and biotic stress responses. In maize (Zea mays), lox5 knockout mutants displayed diminished wound-induced accumulation of multiple oxylipins and defense metabolites, including benzoxazinoids, ABA, and JA-isoleucine (JA-Ile). The lox5 mutant also accumulated lower wound-induced Ca2+ levels (Yuan et al. 2023), which may explain its lower wound-induced JA content. ZmLOX5 contributes to plant defense against insect herbivory by modulating ZmLOX10-mediated signaling via green leaf volatiles (Yuan et al. 2023). In rose (Rosa hybrida), the LOX gene RhLox1 was dramatically induced during petal senescence (Fukuchi-Mizutani et al. 2000). In terms of leaf senescence, 13-lipoxygenase (13-LOX) promotes chloroplast degradation in senescing barley (Hordeum vulgare) plants, which requires its N-terminal chloroplast transit peptide. 13-LOX accumulates in the plastid envelope and catalyzes the dioxygenation of unsaturated membrane fatty acids, leading to selective destruction of the chloroplast and releasing stromal constituents (Springer et al. 2016). Together with our results, we propose that LOX genes play important regulatory roles in plant senescence (Fig. 7).
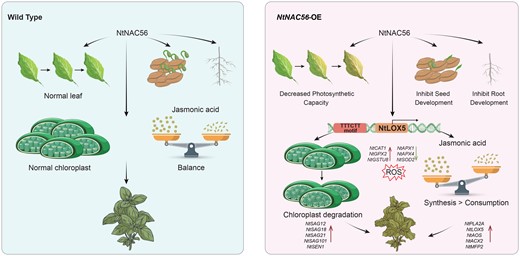
Proposed molecular model of tobacco leaf senescence regulated by NtNAC56. In WT and NtNAC56-overexpression (NtNAC56-OE) tobacco plants, NtNAC56 directly binds to the promoter of the JA biosynthesis-related gene NtLOX5, which promotes chloroplast degradation and JA content increased in transgenic plants. At the same time, the more accumulation of ROS in the NtNAC56-OE lines also promotes leaf senescence.
Carbohydrates can act as signaling molecules and have distinct physiological functions at different stages of plant development. High carbohydrate levels lead to lower photosynthetic activity and can cause leaf senescence when threshold levels are exceeded (Quirino et al. 2000), triggering the expression of some SAGs. Consistent with our transcriptome data, the upregulated genes detected in NtNAC56-OE leaves were associated with carbohydrate binding (Fig. 4B). For most crops, the timing of leaf senescence is critical for proper formation and accumulation of biological yield. Indeed, leaf senescence leads to lower photosynthesis, which greatly limits crop production potential, resulting in lower yields in many crops. In tobacco, a limited number of leaf senescence regulators have been identified. Therefore, it is of great importance for agricultural production to identify the mechanisms and factors influencing leaf senescence.
Conclusion
Here, we identified NtNAC56 as a regulator of leaf senescence in tobacco based on a previous RNA-seq phenotypic analysis showed that NtNAC56 overexpression promotes the premature senescence of tobacco leaves and inhibits plant growth and seed germination. Furthermore, photosynthetic efficiency decreased in NtNAC56-OE lines, and TEM revealed chloroplast degradation in NtNAC56-OE lines while the endogenous JA content increased compared to the WT. Moreover, JA treatment induced NtNAC56 expression. Transcriptome analysis, DAP-seq, and biochemical experiments revealed that NtNAC56 directly targets NtLOX5 to control leaf senescence. Overall, we established that NtNAC56 plays an important role in JA-induced tobacco leaf senescence, and identified a regulatory mechanism that broadens our understanding of senescence in plants.
Materials and methods
Plant materials and growth conditions
The WT tobacco (N. tabacum) cultivar K326 and all transgenic lines, as well as N. benthamiana plants, were grown in a greenhouse at 25 °C under a 16 h light/8 h dark photoperiod (light intensity 100 μmol m−2 s−1) in a peat soil/vermiculite/perlite substrate (3:1:1 by volume). Tobacco (both N. tabacum and N. benthamiana) seeds were surface-sterilized and sown on half-strength Murashige and Skoog (MS) medium (0.8% [w/v] agar, 0.5% [w/v] sucrose, 3 mM MES-KOH, pH 5.8). The seeds were kept at 4 °C for 2 d and then moved to a growth chamber under a 16 h light (100 μmol m−2 s−1)/8 h dark photoperiod at a temperature of 25 °C. The relative humidity was 60% to 70%. Ten-d-old seedlings were planted in soil/vermiculite/perlite (3:1:1 by volume) substrate. Experiments were carried out using 7 to 8-wk-old plants.
The seed germination rates and germination potential experiments were performed with NtNAC56-OE and WT seeds and recorded over 14 d. Seeds of the same size were selected and treated with 70% (v/v) ethanol and then 2.5% (w/v) NaClO, then washed with sterile water, and placed in petri dishes with two layers of sterilized filter paper. One hundred seeds were sown per dish with three technical replicates. Seeds were incubated in the greenhouse at 25 °C under a 16 h light/8 h dark photoperiod (light intensity 100 μmol m−2 s−1). The germination rate was calculated as the ratio of the number of germinated seeds to the total number of tested seeds sown. The germination potential of tobacco seeds was recorded over 7 d and calculated as the percentage of the number of germinated seeds times the total number of seeds sown.
Vector construction and tobacco transformation
To generate transgenic tobacco plants, the full-length NtNAC56 coding sequence was amplified from cDNA and recombined into the Gateway entry vector pENTR/D-TOPO (Thermo Fisher, Massachusetts, USA). The resulting clone was subjected to Sanger sequencing, followed by recombination into the pEarlyGate101 vector by Gateway technology to generate pEarlyGate101-NtNAC56 (35S:NtNAC56-YFP) (Earley et al. 2006). The 1,800-bp promoter region of NtNAC56 was cloned into pCAMBIA1305.1, generating NtNAC56pro:GUS. The CRISPR/Cas9 vector was constructed as described previously (Ma et al. 2015). Specific NtNAC56 genomic target sites were selected and evaluated using targetDesign (http://skl.scau.edu.cn/targetdesign/). The corresponding single guide RNAs (sgRNAs) were then cloned into the pYLCRISPR/Cas9Pubi-N vector. Transgenic plants were obtained via Agrobacterium (A. tumefaciens) (strain GV3101)-mediated transformation (Cardoza and Stewart 2003). Homozygous transgenic lines were used for analyses. Gene-specific primer sequences used for vector construction are listed in Supplementary Table S1.
Photosynthetic parameters
Three plants of each tobacco genotype (WT, NtNAC56-OE, Ntnac56-19) with normal growth were randomly marked to measure photosynthetic indices at 8 wk after germination. The third and fifth fully expanded leaves of tobacco plants were examined. The transpiration rate, photosynthetic rate, intercellular CO2 concentration, and water conductance were measured on a sunny morning with a Li-6800 Portable Photosynthesis System (LI-COR, USA). During measurement process, the light intensity was kept constant (1200 μmol m −2 s−1), and the temperature was 23 °C with ambient CO2 and humidity levels.
Quantification of antioxidant enzyme activity and malondialdehyde and chlorophyll contents
WT, NtNAC56-OE, and Ntnac56 leaves were collected from eight-week-old plants and used to measure MDA content, antioxidant enzyme (POD and CAT) activity, and chlorophyll content.
First, a mixture of 0.6% (w/v) thiobarbituric acid (TBA) and 10% (w/v) trichloroacetic acid (TCA) was prepared, then 0.5 g of ground fresh tobacco leaves (with three replicates) was added to the solution and mixed thoroughly. The solution was heated at 95 °C for 1 h. Then, the sample was allowed to cool to room temperature and centrifuge for 10 min with 10,000×g. And the supernatant was collected. The absorbance of each sample at 532 and 600 nm was recorded; the 0.6% TBA and 10% TCA solution was used as a blank control. MDA content was calculated using the difference between blank absorbance and each sample (Yan et al. 2018). POD and CAT activity measurement kits (A084-3-1 for POD and A007-1-1 for CAT; Nanjing Jiancheng Biological Engineering, Nanjing, China) were used to determine POD and CAT activities. To measure chlorophyll content, three samples were collected from each genotype, placed in a 10 mL centrifuge tube, and mixed with 80% (v/v) acetone to 10 mL. The centrifuge tubes were kept in the dark for 24 h with shaking every 8 to 10 h to completely dissolve the photosynthetic pigments. Using 80% (v/v) acetone as a blank control, the absorbance values were measured at 440, 645, and 663 nm, and the chlorophyll content was calculated as described in Gitelson et al. (2003).
Trypan blue staining
Eight-week-old tobacco leaves of WT and NtNAC56-OE were soaked in the TB (0.67 mg/mL) solution and boiled. The solution was then incubated at room temperature for 3 d, with gentle shaking every 5 h to evenly soak the tobacco leaves. After staining, the leaves were transferred to clearing solution (methanol:glacial acetic acid, 3:1, v/v) and incubated for about 5 d until translucent.
Transmission electron microscopy
Leaves of 8-wk-old WT, NtNAC56-OE, and Ntnac56 tobacco plants were collected and used for TEM observation to check for organelle damage. Samples were fixed in 2.5% (w/v) glutaraldehyde solution at 4 °C overnight, and then rinsed at least three times with phosphate buffer (0.1 M, pH 7.0) to clean them. Before observation, the samples were fixed in 1% (w/v) osmic acid buffer (pH 7.0), and dehydrated through a graded ethanol series, then embedded in EPON resin for TEM observation. Ultrathin sections were generated and imaged using a JEM-1230 TEM (JEOL, Japan).
RNA extraction and RT-qPCR
Total RNA was extracted from tobacco plants using an EZ-10 DNAaway RNA Mini-prep Kit (Tiangen, China) according to the manufacturer's instructions. First-strand cDNA was synthesized using 1 μg of total RNA, SuperScript II reverse transcriptase, and oligo (dT)15 primer (Invitrogen, Carlsbad, CA). qPCR was conducted using a SYBR FAST qPCR Kit according to the manufacturer's instructions (TaRaKa, China). Elongation Factor 1-α (NtEF-1α) and NtActin were used as reference genes to normalize the qPCR data (Schmidt and Delaney 2010). the qPCR primers were designed using from the qPrimerDB database (https://biodb.swu.edu.cn/qprimerdb) (Lu et al. 2018) and are listed in Supplementary Table S2. Relative expression levels were calculated using the 2−ΔΔCt method (Livak and Schmittgen 2001). RNA-seq data were also queried to validate expression data.
RNA-seq and transcriptome analysis
Leaves from WT and NtNAC56-OE tobacco plants were sent to Shanghai Meiji Biomedical Technology for RNA-seq library construction. Paired-end sequencing was used to sequence the libraries, with 100-bp read length. Paired-end reads were generated using an Illumina HiSeq 2500 instrument. Post-trimming, clean reads were aligned to the Nitabv4.5 reference genome (https://solgenomics.net/jbrowse_solgenomics/? data=data/json) using Salmon (v. 0.8.2) (Hahn et al. 2013) with default parameters. Sequence count data inferred from Salmon gene abundance were imported into R and DEGs were identified using DESeq2 (Love et al. 2014) based on the following criteria: false discovery rate (FDR, Benjamini–Hochberg multiple test correction) < 0.05 and Log2(fold-change) > 1.
The functions associated with DEGs were characterized using BiNGO in Cytoscape v3.1.0 (Smoot et al. 2011), and significantly enriched GO terms (FDR < 0.05) were displayed using the R package ggplot2.
MeJA treatment and GUS staining
To investigate the expression patterns of NtNAC56 in leaves in response to JA treatment, 4-wk-old seedlings grown in soil were used. WT tobacco seedlings were treated with 100 μM MeJA, a bioactive JA derivative. Samples were taken at 30 min, 1, 2, 4 and 6 h, and immediately stored in liquid nitrogen. Total RNA was extracted and the expression levels of related genes were detected by RT-qPCR as described above.
WT and transgenic tobacco seedlings harboring the NtNAC56pro:GUS construct were treated with 100 μM MeJA, for 24 h. Samples were fixed in 90% (v/v) acetone and incubated in X-Gluc (5-bromo-4-chloro-3-indolyl-β-D-glucuronide) solution (0.5% [w/v] X-Gluc, 0.5 mM potassium ferricyanide, 0.5 mM potassium ferrocyanide, 0.1 M sodium phosphate, pH 7.4) for 1 h at room temperature. The tobacco seedlings were cleared three times using 70% (v/v) ethanol for visual observation of GUS staining using an Olympus SZ61(Japan). Images were captured using control software ACT-1C for DXM1200C. The software image J (v. 1.53k) was used for image integration and analysis.
Quantification of JA levels
JA was extracted and analyzed as described by Zhang et al. (2018b), with some modifications. Three biological replicates for each genotype (WT and NtNAC56-OE) were used to quantify JA levels. An HPLC 1290 system (Agilent, USA) with tandem mass spectrometry (MS/MS) on a SCIEX-6500Qtrap (Thermo Fisher Scientific, USA) was used to determine JA levels.
Subcellular localization
The 35S:NtNAC56-YFP construct was transformed into Agrobacterium strain GV3101 cells. Leaves of 28-d-old N. benthamiana plants were infiltrated and the YFP signal was detected 2 d later using a confocal microscope (LSM 510 META; Carl Zeiss) with 514 nm for excitation, 570 nm for emission and 519 to 620 nm for detection.
Transcriptional activation and Y1H assays
For self-activation assays in yeast (Saccharomyces cerevisiae), the full-length NtNAC56 coding sequence was amplified from cDNA using PrimeSTAR Max DNA Polymerase (Takara) and subcloned into the EcoRI and BamHI sites of pGBKT7. All primers used for cloning are listed in Supplementary Table S1. pGBKT7-NtNAC56 and the empty pGBKT7 vector were transformed into yeast using the polyethylene glycol (PEG)/lithium acetate (LiAc) method, and yeast cells were plated on synthetic defined (SD)/–His medium for selection and on SD/–His/–Trp/–Leu medium for stringent screening of activation.
For Y1H assays, the full-length NtNAC56 coding sequence was amplified using PrimeSTAR Max DNA Polymerase (Takara, Japan) and subcloned into the pGADT7vector by EcoRI and BamHI sitesto generate the prey vector. To generate the bait vector, a 1,500-bp promoter fragment from the target gene (LIPOXYGENASE 5, NtLOX5) was amplified by PCR and cloned into the pAbAi vector through SacI and XhoI sites. All primers used for cloning are listed in Supplementary Table S1. Bait and prey vectors, as well as empty vectors, were transformed into yeast strain Y1HGold using the PEG/LiAc method. Yeast transformants were selected on SD/–Ura/–Leu medium; yeast cultures of positive transformants were spotted onto SD/–Ura/–Leu + Aureobasidin A (AbA) medium for stringent screening of the possible interactions, according to the protocol of the Y1HGold-pAbAi Yeast One Hybrid kit (Coolaber).
Dual-luciferase reporter assay
The promoter fragments of putative downstream binding genes or binding motifs were cloned into the pGreenII 0800-LUC vector using the primers listed in Supplementary Table S1. The full-length NtNAC56 coding sequence was subcloned into the pGreenII 62-SK vector with BamHI and EcoRI sites to generate the effector construct. The Renilla luciferase gene (REN) present in the vector under the control of the 35S promoter (35S:REN) was used as an endogenous control. The dual-luciferase assay was performed as described previously (Hellens et al. 2005). LUC and REN activities were measured using a Dual Luciferase Reporter Assay System (Promega, Madison, WI) on a GloMax 20/20 Luminometer (Promega, Madison, WI) according to the manufacturer's instructions. Three independent measurements were acquired for each construct combination.
DNA affinity purification sequencing (DAP-seq) and analysis of NtNAC56 binding sites
DAP-seq analysis was conducted according to the method of Liu et al. (2023) with modifications. To construct DAP-seq libraries, 5 μg of tobacco genomic DNA (gDNA) was fragmented to an average of 200 bp. The NtNAC56 coding sequence was cloned into the halo tag vector to generate the as Halo-NtNAC56 fusion protein and translated using a TNT SP6 Coupled Wheat Germ Extract System (Promega, Madison, WI, USA) at room temperature for 2 h. Halo tag protein-bound beads were incubated with adaptor-ligated gDNA fragments and shaken gently at for 1 h at room temperature. After the bound gDNA fragments were released and the protein was denatured, eluted gDNA fragments were sequenced on an Illumina NovaSeq system. Reads were mapped to the Nitabv4.5 reference genome using HISAT2 (Kim et al. 2015). MACS2 software coupled with the IDR pipeline was used to analyze the DAP-seq peaks (Zhang et al. 2008), and MEME-Chip suite v5.0.5 was used for the binding motifs discovery (Machanick and Bailey 2011).
Statistical analysis
Statistical analysis and data visualization were performed in software GraphPad Prism (v. 8.0.2) (https://www.graphpad.com/) and image J (v. 1.53k). All experiments were performed at least in triplicate. Data are presented as means ± standard deviation (Sd). Student's t-test was used to identify significant differences: *P < 0.05; **P < 0.01, ***P < 0.001, ****P < 0.0001.
Accession numbers
The raw transcriptome sequencing data and the DAP-seq data for tobacco leaves from WT and NtNAC56-OE plants have been deposited into the Genome Sequence Archive (GSA) database in BIG Data Center (https://ngdc.cncb.ac.cn/gsa) under accession numbers PRJCA018772 and PRJCA022355. Tobacco sequence data used in this article is available under accession numbers: mRNA-80414 (LOC107805064), mRNA_70561 (LOC107762818), mRNA_32336 (LOC107789644), mRNA_125220 (LOC107789325). Arabidopsis sequence data used in this article are form The Arabidopsis Information Resource website (https://www.arabidopsis.org/index.jsp) under accession number: At3g15510 (ANAC056).
Acknowledgments
The authors are grateful to Biyue Ding and Yan Zhou from the Instrument and Equipment Sharing Platform of LargeScale Instruments & Equipments in Academy of Agricultural Science, Southwest University, China. The authors are grateful to professional editors from the Plant Editors for critical, clear, and concise reading and improving of the manuscript.
Author contributions
K.L. and B.L.: Conceptualization, Methodology. W.C., H.Z., H.C., G.J., and H.X.: Performed the sampling and experiments, Writing—Original draft preparation. B.M., X.L., M.Y., S.L., and M.Q.: Collected the phenotypes and performed the data analysis. Y.F.: Data curation. X.L. and B.L.: Software. K.Z., J.Y., and B.W.: Validation. W.C., B.L., and K.L.: Visualization, Writing—reviewing and editing.
Supplementary data
The following materials are available in the online version of this article.
Supplementary Figure S1. Protein domain and sequence analysis of NtNAC56 homologs in tobacco and Arabidopsis.
Supplementary Figure S2. Identification of transgenic overexpression lines and gene-edited knockout mutants.
Supplementary Figure S3. Photosynthesis indicators in WT, Ntnac56-19, and NtNAC56-OE tobacco plants.
Supplementary Figure S4. RT-qPCR analysis of the expression of genes related to ROS in WT and NtNAC56-OE tobacco plants.
Supplementary Figure S5. Bioinformatics prediction of the cis-regulatory elements in the upstream 1,800 bp promoter of NtNAC56.
Supplementary Figure S6. Bioinformatics analysis predicting the nuclear localization signal of NtNAC56.
Supplementary Figure S7. Genome-wide distribution of NtNAC56-binding sites in tobacco.
Supplementary Figure S8. Enriched DNA motifs identified in NtNAC56-binding sites.
Supplementary Table S1. A list of primers used for vector construction.
Supplementary Table S2. A list of primers used for RT-qPCR.
Supplementary Table S3. GO analysis of DEGs between WT and NtNAC56-OE leaves.
Supplementary Table S4. DAP-seq data mapping information.
Funding
This work was supported by the National Natural Science Foundation of China (32260528), Special fund for youth team of the Southwest Universities (SWU-XJPY202306), Chongqing Postgraduate Research and Innovation Projects (CYB23129 and CYS22225), the Program of Guizhou Branch of China National Tobacco Corporation (2021XM03).
Data availability
The data underlying this article are available in the article and in its online supplementary material.
Dive Curated Terms
The following phenotypic, genotypic, and functional terms are of significance to the work described in this paper:
References
Author notes
Conflict of interest statement. None declared.