-
PDF
- Split View
-
Views
-
Cite
Cite
Chao Li, Shaofeng Luo, Lu Feng, Qianqian Wang, Jiasen Cheng, Jiatao Xie, Yang Lin, Yanping Fu, Daohong Jiang, Tao Chen, Protist ubiquitin ligase effector PbE3-2 targets cysteine protease RD21A to impede plant immunity, Plant Physiology, Volume 194, Issue 3, March 2024, Pages 1764–1778, https://doi.org/10.1093/plphys/kiad603
- Share Icon Share
Abstract
Clubroot, caused by the soil-borne protist pathogen Plasmodiophora brassicae, is one of the most devastating diseases of Brassica oil and vegetable crops worldwide. Understanding the pathogen infection strategy is crucial for the development of disease control. However, because of its obligate biotrophic nature, the molecular mechanism by which this pathogen promotes infection remains largely unknown. P. brassicae E3 ubiquitin ligase 2 (PbE3-2) is a Really Interesting New Gene (RING)-type E3 ubiquitin ligase in P. brassicae with E3 ligase activity in vitro. Yeast (Saccharomyces cerevisiae) invertase assay and apoplast washing fluid extraction showed that PbE3-2 harbors a functional signal peptide. Overexpression of PbE3-2 in Arabidopsis (Arabidopsis thaliana) resulted in higher susceptibility to P. brassicae and decreases in chitin-triggered reactive oxygen species burst and expression of marker genes in salicylic acid signaling. PbE3-2 interacted with and ubiquitinated host cysteine protease RESPONSIVE TO DEHYDRATION 21A (RD21A) in vitro and in vivo. Mutant plants deficient in RD21A exhibited similar susceptibility and compromised immune responses as in PbE3-2 overexpression plants. We show that PbE3-2, which targets RD21A, is an important virulence factor for P. brassicae. Two other secretory RING-type E3 ubiquitin ligases in P. brassicae performed the same function as PbE3-2 and ubiquitinated RD21A. This study reveals a substantial virulence functional role of protist E3 ubiquitin ligases and demonstrates a mechanism by which protist E3 ubiquitin ligases degrade host immune-associated cysteine proteases to impede host immunity.
Introduction
Plasmodiophora brassicae, causing clubroot disease worldwide, is an obligate biotrophic pathogen. It produces club-shaped galls by parasitizing the roots of cruciferous plants. The above-ground part of the plant tends to show the symptoms of wilting, stunting, yellowing, premature senescence, or death (Hwang et al. 2012). A global loss of 10% to 15% in yield and 2% to 6% in oil content is attributed to clubroot disease. Clubroot pathogen and disease have been reported in more than 80 countries in the world (Javed et al. 2023). Breeding and cultivation of resistant varieties is the most effective approach to mitigate the threats of this disease (Guo et al. 2022). However, new virulent pathotypes of P. brassicae have been constantly emerging (Galindo-González et al. 2020). Understanding the mechanism for successful infection of P. brassicae on the host plants will facilitate the breeding of new resistant cultivars and disease management.
P. brassicae is an obligate biotrophic protist appertaining to a subgroup of Rhizaria, one of the most poorly understood subsets of eukaryotes. The obligate lifestyle largely hinders investigation of the molecular mechanisms for pathogenic infection. Delivering effectors into host cells during infection to manipulate plant defenses and facilitate parasitic colonization is an advanced strategy evolved by many eukaryotic biotrophic pathogens (Dodds and Rathjen 2010). Identification of effectors of P. brassicae is critical for understanding how the pathogen manipulates the immune response of plants and regulates clubroot resistance. The availability of the genome and transcriptome of P. brassicae pathotypes provides an opportunity for identifying the putative effectors (Schwelm et al. 2015; Bi et al. 2016; Rolfe et al. 2016). The first effector identified and characterized in P. brassicae is a methyltransferase (PbBSMT), which may methylate salicylic acid (SA) in host cells to manipulate plant immunity (Ludwig-Müller et al. 2015). P. brassicae effector SSPbP53 suppresses plant immunity by inhibiting cruciferous papain-like cysteine proteases (PLCPs) (Pérez-López et al. 2021). P. brassicae RxLR effector that contains zinc finger Myeloid-Nervy-DEAF1 (PBZF1) interacts with and inhibits the biological function of SnRK1.1. Heterologous expression of PBZF1 in Arabidopsis (Arabidopsis thaliana) increases the susceptibility of plants to P. brassicae (Chen et al. 2021). To better understand the pathogenic mechanism of P. brassicae, it is necessary to further study the roles and molecular mechanisms of P. brassicae effector candidates.
Ubiquitination, an important posttranslational modification of proteins unique to eukaryotes (Hershko and Ciechanover 1998), serves as a degradation signal or changes the property of the target protein (Smalle and Vierstra 2004). Usually, ubiquitin ligase E3 can specifically recognize target proteins and is therefore particularly important and extensively studied. Ubiquitination is involved in various mechanisms including cell cycle, apoptosis, protein degradation, and immune regulation and is therefore one of the most important mechanisms regulating various life activities (Hershko and Ciechanover 1998; Smalle and Vierstra 2004; Kim et al. 2010). To date, 139 putative E3 ubiquitin ligases have been predicted in the P. brassicae e3 genome, among which PbRING1 contains a functional signal peptide and has E3 ligase activity in vitro (Yu et al 2019). However, the biological functions of E3 ubiquitin ligases in P. brassicae remain largely unknown.
Many studies have revealed that ubiquitination is extensively involved in the regulation of plant immunity (Zhou and Zeng 2017). A. thaliana E3 ubiquitin ligases PUB12/PUB13 and PUB22 ubiquitinate the pattern recognition receptor FLS2 and the exocyst complex subunit Exo70B2, respectively, to promote their degradation to attenuate PAMP-triggered immune (PTI) signaling (Lu et al. 2011; Stegmann et al. 2012). Besides, PUB12/PUB13 also mediates the degradation of abscisic acid (ABA) coreceptor ABI1 to regulate ABA signaling (Kong et al. 2015). In addition, pathogens secrete effectors to influence plant immunity by regulating the host ubiquitination system. Rice (Oryza sativa) E3 ligases APIP6 and APIP10 are targeted by Magnaporthe oryzae effector AvrPiz-t, resulting in weakened PTI (Park et al. 2012, 2016). AvrptoB, a type III effector with E3 ligase activity, is important for the pathogenicity of Pseudomonas syringae (Abramovitch et al. 2006).
This study aims to investigate the biological functions of secreted E3 ubiquitin ligases in P. brassicae and understand the mechanisms for ubiquitination in clubroot development. Our findings indicate that the protist pathogen E3 ubiquitin ligases ubiquitinate the plant cysteine protease RD21, thereby suppressing plant immunity for successful infection.
Results
PbE3-2 is a RING-type E3 ubiquitin ligase
By using Blast2GO to systemically investigate the genome of P. brassicae ZJ-1 (Bi et al. 2019), 60 genes were predicted to encode putative E3 ubiquitin ligases (Supplemental Table S1). We hypothesized that some of these E3 ubiquitin ligases are secreted into the host cell and contribute to successful infection. Five out of the 60 predicted E3 ubiquitin ligases possessed signal peptides, which were designated as PbE3-1, PbE3-2, PbE3-3, PbE3-4, and PbE3-5, respectively (Supplemental Table S1 and Fig. S1A). PbE3-1 and PbE3-5 were predicted to have transmembrane domain, while the remaining 3 PbE3s had no such domain (Supplemental Fig. S1A). Reverse transcription quantitative PCR (RT-qPCR) analysis indicated that these 5 PbE3s have differential expression patterns at different life stages of P. brassicae, and PbE3-2, PbE3-3, and PbE3-4 were highly expressed during cortex infection (secondary infection) (Supplemental Fig. S1B). PbE3-2 harbored a Really Interesting New Gene (RING) finger domain (Supplemental Table S1) and is conserved among P. brassicae pathotypes (Supplemental Fig. S1C). The RING finger domain of PbE3-2 contained 8 conserved Cys/His amino acid residues and thus belonged to the C3H2C3-type family (Fang and Weissman 2004) and could coordinate 2 Zn2+ ions (Fig. 1A). The 3D model of the RING finger domain in PbE3-2 was similar to that of the canonical RING from maize (Zea mays) (Fig. 1B). We first tested whether PbE3-2 has an enzymatic activity for self-ubiquitination. AtUBA1-S (E1 activating enzyme), AtUBC8-S (E2 conjugating enzyme), PbE3-2-Myc, and His-FLAG-UBQ10 (ubiquitin) were expressed in Escherichia coli DE3 strains, and self-ubiquitination of PbE3-2 was tested in the presence or absence of E1, E2, and ubiquitin. PbE3-2 was converted into a mixture of high-Mr ubiquitinated protein products (Fig. 1C), indicating the ability of PbE3-2 to self-ubiquitinate. The PbE3-2 (C423S) mutant was generated by replacing the cysteine residue in the RING structure at Position 423 with a serine. As a result, PbE3-2 (C423S) showed no self-ubiquitination (Fig. 1C). Therefore, PbE3-2 is an E3 ubiquitin ligase.
![PbE3-2 is a secreted E3 ubiquitin ligase and suppressed BAX-induced cell death. A) Protein alignment of PbE3-2 RING and its homologs (Symbiodinium microadriaticum OLP82957.1, Theobroma cacao EOY12354.1, Camelina sativa XP_010413448.1, Gossypium arboretum KHG04456.1, Toxoplasma gondii VEG CEL71473.1, Vigna radiata var. radiata XP_014517466.1, and Monoraphidium neglectum XP_013905359.1). B) Structures of PbE3-2 RING and RING finger protein 165 (Z. mays). C) Self-ubiquitination of PbE3-2. Ub, E1, E2, and PbE3-2 are all present in the reconstituted systems. PbE3-2 can undergo self-ubiquitination. The system lack of any component is a negative control. The PbE3-2 (C423S) mutant is also unable to undergo autoubiquitination. D) PbE3-2-GFP and PbE3-2ΔSPGFP infiltrated to N. benthamiana leaves for 2 d, apoplast washing fluid was extracted, and western blotting with GFP antibody indicated that PbE3-2-GFP but not PbE3-2ΔSPGFP was detected in the apoplast washing fluid. E) The signal peptide of PbE3-2 has a secretory function. Clones carrying the pSUC2 plasmid can be grown in CMD-W medium (0.67% yeast N base without amino acids, Trp dropout supplement, 2% [w/v] sucrose, 0.1% [w/v] glucose, and 2% [w/v] agar). Yeast secreted invertase degrades cottonseed on YPRAA medium (1% [w/v] yeast extract, 2% [w/v] peptone, 2% [w/v] raffinose, 2-μg/L antimycin A, and 2% [w/v] agar) for growth. TTC is reduced by invertase to red insoluble formazan. pSUC2-avr1b is used as a positive control and pSUC2 empty vector as a negative control. F) PbE3-2 and PbE3-2ΔSP suppressed BAX-induced cell death. PbE3-2 and BAX coinfiltrated to N. benthamiana leaves, and BAX and PVX-GFP coinfiltrated as a negative control. Left picture was taken at 5 d after infiltration under visible light, middle picture was taken under UV light, and right picture was decolorization with 75% (v/v) acetic acid and 25% (v/v) alcohol buffer. Experiments were independently performed 3 times with similar results.](https://oup.silverchair-cdn.com/oup/backfile/Content_public/Journal/plphys/194/3/10.1093_plphys_kiad603/1/m_kiad603f1.jpeg?Expires=1747928326&Signature=BQQ5uxaIiQidME62AZ1sUZCnQmOnTaXR8d8lxHM4-hLqjmdaJnm07Jw4KKUSdvGUEALkU-zwce6ornnSRKqmz7PkwH3Szn4SoidLhkcuEfGQlVH0dkBGjKDR5UNsZAhnYQogYLnlF0rDAvwohlvK6~LduOU~dZyysSfJGG9i-WN5POse5XEUHFopM1UGuJV6xYcHds1LZc4LTbgfd9zGpljHZAMYMUQqucifhMpYKecvRHd4M0g4uqmk7c9XHkiKbh0rBXQgleoKCc8o4ht5qKn9IIVBFIbVt6TCVlO0j5mFjpTExErb3MdkI7pq77QJqWjPKR1YWbxpPWZfPfwijw__&Key-Pair-Id=APKAIE5G5CRDK6RD3PGA)
PbE3-2 is a secreted E3 ubiquitin ligase and suppressed BAX-induced cell death. A) Protein alignment of PbE3-2 RING and its homologs (Symbiodinium microadriaticum OLP82957.1, Theobroma cacao EOY12354.1, Camelina sativa XP_010413448.1, Gossypium arboretum KHG04456.1, Toxoplasma gondii VEG CEL71473.1, Vigna radiata var. radiata XP_014517466.1, and Monoraphidium neglectum XP_013905359.1). B) Structures of PbE3-2 RING and RING finger protein 165 (Z. mays). C) Self-ubiquitination of PbE3-2. Ub, E1, E2, and PbE3-2 are all present in the reconstituted systems. PbE3-2 can undergo self-ubiquitination. The system lack of any component is a negative control. The PbE3-2 (C423S) mutant is also unable to undergo autoubiquitination. D) PbE3-2-GFP and PbE3-2ΔSPGFP infiltrated to N. benthamiana leaves for 2 d, apoplast washing fluid was extracted, and western blotting with GFP antibody indicated that PbE3-2-GFP but not PbE3-2ΔSPGFP was detected in the apoplast washing fluid. E) The signal peptide of PbE3-2 has a secretory function. Clones carrying the pSUC2 plasmid can be grown in CMD-W medium (0.67% yeast N base without amino acids, Trp dropout supplement, 2% [w/v] sucrose, 0.1% [w/v] glucose, and 2% [w/v] agar). Yeast secreted invertase degrades cottonseed on YPRAA medium (1% [w/v] yeast extract, 2% [w/v] peptone, 2% [w/v] raffinose, 2-μg/L antimycin A, and 2% [w/v] agar) for growth. TTC is reduced by invertase to red insoluble formazan. pSUC2-avr1b is used as a positive control and pSUC2 empty vector as a negative control. F) PbE3-2 and PbE3-2ΔSP suppressed BAX-induced cell death. PbE3-2 and BAX coinfiltrated to N. benthamiana leaves, and BAX and PVX-GFP coinfiltrated as a negative control. Left picture was taken at 5 d after infiltration under visible light, middle picture was taken under UV light, and right picture was decolorization with 75% (v/v) acetic acid and 25% (v/v) alcohol buffer. Experiments were independently performed 3 times with similar results.
PbE3-2 suppresses BAX-induced cell death
Secretory proteins, as candidate effectors, are important for the interaction between P. brassicae and its hosts (Pérez-López et al. 2018). To determine whether PbE3-2 is secretory, we constructed 2 plasmids expressing PbE3-2-GFP fusion proteins with (PbE3-2-GFP) and without signal peptide (PbE3-2ΔSP-GFP) and delivered them into Nicotiana benthamiana epidermal cells via Agrobacterium tumefaciens. Apoplast washing fluid was extracted, and western blotting indicated that PbE3-2 but not PbE3-2ΔSP was detected in the apoplast washing fluid (Fig. 1D), suggesting that PbE3-2 is most likely a secretory protein. The secretory nature of PbE3-2 was also confirmed by yeast invertase assay (Fig. 1E). Transient expression assays in the N. benthamiana model system have been performed to identify effectors in bacteria, oomycetes, fungi, nematodes, and protist P. brassicae (Zhan et al. 2022). Many predicted candidate effectors of P. brassicae were assessed by inducing cell death or suppressing plant immunity triggered by BAX in N. benthamiana (Chen et al. 2019; Zhan et al. 2022). Thus, PVX plasmid constructs of PbE3-2 or GFP were coexpressed with Bax in N. benthamiana leaves (Fig. 1F). PbE3-2 and PbE3-2ΔSP but not GFP suppressed BAX-induced cell death in infiltrated leaves. These results indicated that PbE3-2 is a candidate effector that suppresses BAX-induced cell death.
PbE3-2 promotes the development of clubroot
To characterize the biological functions of PbE3-2, we generated stable transgenic A. thaliana lines constitutively expressing the PbE3-2 coding sequence. Independent homozygous A. thaliana lines (OX2, OX5, and OX6) were identified by using western blotting analysis (Supplemental Fig. S2, A and B). The transgenic plants displayed similar growth phenotypes to Col-0 plants (Supplemental Fig. S2A). To determine whether PbE3-2 contributes to clubroot disease, transgenic lines and Col-0 were challenged with P. brassicae, and the phenotype was investigated at 21 d postinoculation (dpi) (Fig. 2A; Supplemental Fig. S2C). The roots of wild-type plants showed the formation of typical galls and correspondingly fewer rootlets, and severe galls (disease classes 5) accounted for 12.8% of the total (Fig. 2, A and B); besides, the infected plants exhibited purple leaves (Supplemental Fig. S2C). The PbE3-2 transgenic lines showed more severe symptoms than Col-0, with severe galls (disease classes 5) on the roots accounting for 50% to 60%, and leaves were dark purple and yellow (Fig. 2, A and B; Supplemental Fig. S2C). The disease index of Col-0 was 64.1, while that of transgenic lines was 90.3 to 91.6 (Fig. 2B). Quantification of P. brassicae biomass showed that PbE3-2 transgenic plants had a more than 10-fold higher amount of P. brassicae than Col-0 (Fig. 2, C and D). These results indicated that PbE3-2 transgenic plants are more susceptible to P. brassicae than Col-0. Although P. brassica is a biotrophic pathogen, its effector PbE3-2 may interfere with the overall immune level of the plant, thereby affecting the resistance to necrotrophic pathogens. Hence, we assessed the susceptibility of PbE3-2 transgenic lines to the necrotrophic fungi Botrytis cinerea and Sclerotinia sclerotiorum. As a result, the PbE3-2 transgenic lines exhibited more significant symptoms of fungal infection than Col-0 (Fig. 2, E to H), indicating that PbE3-2 also modulates the susceptibility of A. thaliana to necrotrophic pathogens.
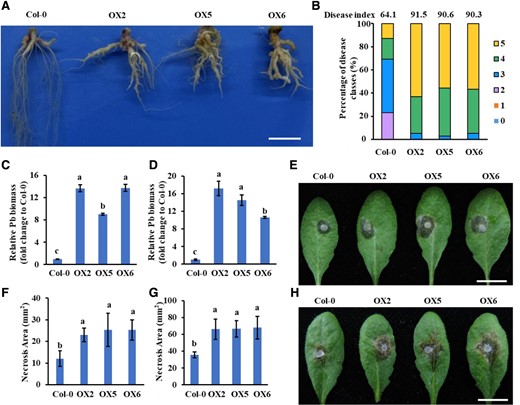
PbE3-2 overexpression plants are more susceptible to pathogens. A, B) Col-0 and PbE3-2 overexpression plants inoculated with P. brassicae for 21 d. Root phenotype of Col-0 and PbE3-2 overexpression plants inoculated with P. brassicaeA). Bar: 1 cm. Disease index of Col-0 and PbE3-2 overexpression plants inoculated with P. brassicaeB). Percentages of plants in the individual disease classes are shown. For transgenic lines and Col-0 plants, 35 to 40 plants were analyzed. The disease index for each sample is shown as a number above the respective histograms. The experiment was conducted with 3 independent replications with similar results. C, D) Quantification of P. brassicae content in diseased roots of Arabidopsis by qPCR, and the vertical coordinates indicate the multiplicity of Pb content in different lines relative to Col-0. The relative biomass of P. brassicae is represented by the content of the P. brassicae ACTIN gene relative to that of the Arabidopsis ACTIN2 gene C) and the content of the P. brassicae DC1 gene relative to that of the Arabidopsis GAPDH gene D). Relative Pb biomass is presented as the means ± Sdn = 3 biological replicates. Bar: 1 cm. Experiments were repeated 3 times with similar results. E, F) Col-0 and PbE3-2 overexpression plants were inoculated with B. cinerea; pictures were taken at 24 h postinoculation (hpi) E), and disease spot area data F) are presented as the means ± Sd (n = 10 biological replicates). Bar: 1 cm. Experiments were repeated twice with similar results. G, H) Col-0 and PbE3-2 overexpression plants were challenged with S. sclerotiorum, 30 hpi H) and disease spot area after inoculation of S. sclerotiorum in Col-0 and PbE3-2 overexpression plants G). Data are presented as the means ± Sd (n = 10 biological replicates). Bar: 1 cm. Experiments were repeated twice with similar results. Statistical tests used in C), D), F), and G) were 1-way ANOVA with Kruskal–Wallis test (significance set at P ≤ 0.05). Different letters (a, b, and c) indicate significant differences.
PbE3-2 suppresses plant immune response
To complete its life cycle, P. brassicae needs to evade from or downregulate PAMP-triggered immunity (Pérez-López et al. 2021). PTI plays a prominent role in curtailing pathogen invasion (Melotto et al. 2006) and leads to a number of overlapping downstream outputs, such as MAP kinase (MAPK) cascades, calcium flux, reactive oxygen species (ROS) burst, and transcriptional reprogramming (Devendrakumar et al. 2018; Peng et al. 2018). We tested whether PbE3-2 suppresses typical immune responses such as ROS burst, MAPK activation, and immune gene induction in response to PAMP. Chitin-triggered ROS burst was reduced by about 50% in PbE3-2 transgenic plants relative to Col-0 (Fig. 3A). Moreover, the induced expression of RBOHD was also remarkably suppressed in PbE3-2 transgenic plants (Supplemental Fig. S3A). However, a comparative analysis of MAPK activation showed no substantial difference between these treatments (Supplemental Fig. S3B). The SA signaling pathway is a central pathway for plant defense against P. brassicae (Vlot et al. 2009; Ludwig-Müller et al. 2015; Chen et al. 2016). We compared the induction levels of marker genes of the SA signaling pathway to chitin treatment in PbE3-2 transgenic lines and Col-0 plants. As expected, the induced expression of these marker genes was suppressed in the PbE3-2 transgenic lines (Fig. 3, B and C). Under the induction of chitin, SA accumulation in PbE3-2 transgenic lines was significantly decreased compared with Col-0 (Fig. 3D). These results indicated that PbE3-2 may suppress PTI responses by manipulating host ROS burst or SA signaling pathway consistently with our observation that PbE3-2 promotes clubroot disease.
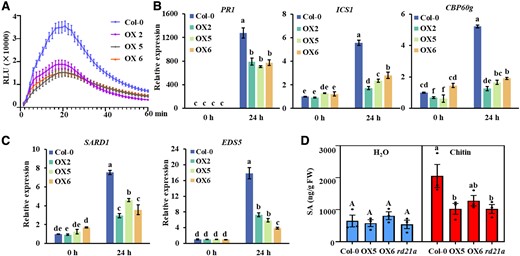
PbE3-2 suppresses plant immune response. A) Chitin-induced ROS burst is suppressed in PbE3-2 overexpression lines. The values represent means ± Se (n = 16 biological replicates). Experiments were repeated 3 times with similar results. B, C) Induction of the salicylate pathway genes was compromised in PbE3-2 overexpression lines. Leaves of 4-wk-old plants were infiltrated with 20-μg/mL chitin for 24 h. Data were normalized to ACTIN2 expression in qPCR analysis. Statistical analysis in B) and C) were performed by 1-way ANOVA with Kruskal–Wallis test (significance set at P ≤ 0.05). Different letters (a, b, c, d, and e) are significantly different. n = 3 biological replicates; data are shown as mean ± Sd. Experiments were repeated 3 times with similar results. D) Salicylic acid metabolite quantification of 24 h after hand infiltration with 20 μg/mL of chitin or H2O of 4-wk-old Arabidopsis leaves. Statistical analysis was performed by 1-way ANOVA with Kruskal–Wallis test (significance set at P ≤ 0.05). Different letters (a and b) indicate significant differences. n = 3 biological replicates; data are shown as mean ± Se.
PbE3-2 interacts with the plant cysteine protease RD21A
To identify the host target of PbE3-2, 35S:PbE3-2-GFP transgenic A. thaliana lines infected with P. brassicae were prepared. Immunoprecipitation (IP) and liquid chromatography–MS (LC-MS) identified 268 potential interacting proteins of PbE3-2 (Supplemental Table S2). We next screened A. thaliana cysteine protease RESPONSIVE TO DEHYDRATION 21A (RD21A) in the yeast 2-hybrid (Y2H) system and in yeast PbE3-2 interacted with RD21A (Fig. 4A). The interaction was validated with a split-luciferase (LUC) complementation assay (Fig. 4B; Supplemental Fig. S5C) and further examined by Co-IP assay by tagging PbE3-2 with GFP whereas tagging RD21A with FLAG, which were coexpressed in N. benthamiana leaves. As shown in Fig. 4C, FLAG-tagged RD21A could be coimmunoprecipitated with GFP-tagged PbE3-2. These results indicated that PbE3-2 interacts with RD21A in planta. We further verified the interaction between PbE3-2 and RD21A using a pull-down assay in vitro. PbE3-2 was fused with His tag and RD21A was fused with glutathione S-transferase (GST), which were purified, incubated, and then immobilized to glutathione sepharose beads. Immunoblotting with α-His antibody detected that PbE3-2 was pulled down by RD21A-GST but not GST (Fig. 4D). We coexpressed PbE3-2 and RD21A in plant cells as recombinant proteins fused with split yellow fluorescent protein tags. Poor fluorescent signals were detected in the plasma membrane and cytoplasm (Supplemental Fig. S4A). Interestingly, when PbE3-2 (C423S) and RD21A were coexpressed in plant cells with split red fluorescent protein tags, strong red fluorescent signals were observed in the plasma membrane and the cytoplasm of leaf cells (Fig. 4E), suggesting that PbE3-2 (C423S) and RD21A interact with each other in plant cells. Subcellular colocalization revealed that PbE3-2-GFP and RD21A-mCherry fluorescence signals were overlapped in the plasma membrane and cytoplasm (Supplemental Fig. S4C). This result is consistent with the localization of PbE3-2 (Supplemental Fig. S4B) and similar to the finding of Liu et al. (2020) that mature RD21A (mRD21A) interacts with the E3 ligase SINAT4 in the cytosol and plasma membrane. Taken together, these results suggest that PbE3-2 directly interacts with RD21A in vivo and in vitro.
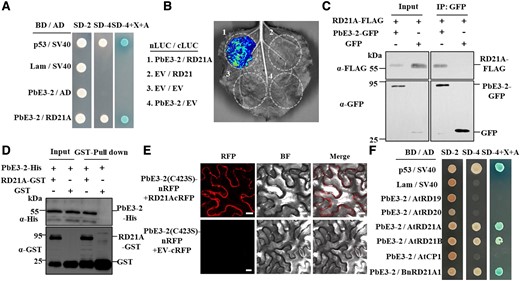
PbE3-2 interacts with A. thaliana cysteine protease RD21A. A) Y2H assays show that PbE3-2 interacts with RD21A. BD indicated pGBKT7 vector. BD indicates pGBKT7 vector. AD indicates pGADT7 vector. p53 + SV40 is a positive control, Lam + SV40 is a negative control. SD–Leu–Trp medium (SD-2) was used for screening clones harboring both plasmids and SD–Leu–Trp–His–Ade medium (SD-4) with/without X-a-Gal (X) and aureobasidin A (A) were used for verifying the interactions. B) Interaction between PbE3-2 and RD21A in split-LUC reconstitution assay. The constructs of N-terminal luciferase (nLUC) and C-terminal luciferase (cLUC) were coexpressed in N. benthamiana leaves. The combination containing the empty vector was used as a negative control. C) Co-IP confirmed that PbE3-2 interacts with RD21A in vivo. IP was performed with α-GFP agarose beads, followed by the detection of RD21 with α-FLAG antibody. Input and IP proteins were immunoblotted with α-FLAG and α-GFP antibodies. D) The direct interaction between PbE3-2 and RD21A was demonstrated by pull-down. Mixtures of PbE3-2-6 × His with GST-RD21A or GST were incubated with glutathione agarose beads for 3 h, and then the agarose beads were collected and washed 3 times and eluted. Immunoblotting of input and pull-down proteins was performed using α-His and α-GST antibodies. E) BiFC confirmed the interaction of PbE3-2 (C423S) and RD21A. PbE3-2 (C423S) is fused to the N-terminus of RFP; full-length RD21A is fused to the C-terminus of RFP. The corresponding GV3101 strains carrying the target plasmid are transiently expressed in the N. benthamiana and observed with a confocal laser-scanning microscope. Bars: 20 μm. Experiments were repeated twice with similar results. F) Y2H assays verified the specificity of PbE3-2 interaction with RD21A. PbE3-2 interacted with the Arabidopsis cysteine proteases AtRD21A (AT1G47128) and AtRD21B (AT5G43060) but not with the Arabidopsis cysteine proteases RESPONSIVE TO DEHYDRATION 19 (AtRD19) (AT4G39090) and AtCP1 (AT4G11310). PbE3-2 also did not interact with AtRD20 (AT2G33380). PbE3-2 interacted with RD21 homologs in B. napus (BnRD21A1 and BnaA08g04080D). Experiments in A), B), C), D), and F) were repeated 3 times with similar results.
Several RD21A-related genes were also cloned to examine the interaction with PbE3-2, including the cysteine proteases AtRD19, AtRD21B, and cysteine protease 1 (AtCP1), RESPONSIVE TO DESSICATION 20 (AtRD20) from A. thaliana, and BnRD21A1 from Brassica napus, an ortholog of A. thaliana AtRD21A. The results demonstrated that PbE3-2 interacts with AtRD21A, AtRD21B, and BnRD21A1 but not with AtRD19, AtRD20, and AtCP1 in yeast cells (Fig. 4F; Supplemental Fig. S6E). These results suggested that PbE3-2 interacts explicitly with cysteine proteases in the RD21 clade. Three orthologs of AtRD21A in rapeseed that can interact with PbE3-2 were examined by split-LUC complementation assays (Supplemental Fig. S5). The results demonstrated that the interactions between PbE3-2 and RD21 orthologs are conserved.
Full-length AtRD21A contains a signal peptide, an autoinhibitory precursor protein, a protease domain, a proline-rich region, and a granule protein domain (Yamada et al. 2001; Gu et al. 2012; Pogorelko et al. 2019). According to available information, this precursor protein has undergone extensive posttranslational processing, resulting in the formation of intermediate RD21A (iRD21A, removal of signal peptide and autoinhibitory prodomain) and mature/active RD21A (mRD21A, consisting of only the protease domain). To identify the structural requirements for the interaction between RD21A and PbE3-2, constructs of RD21A and its 4 truncated derivatives were used to analyze the potential interactions with PbE3-2 (Supplemental Fig. S6). PbE3-2 interacted with RD21A, iRD21A, mRD21A (Supplemental Fig. S6, C and E). Among the 4 constructs, the one containing the protease domain exhibited a stronger interaction with PbE3-2, suggesting that the protease domain of RD21A is responsible for its interaction with PbE3-2. Besides, the granule protein domain had a much weaker interaction with PbE3-2 in the colony growth assay (Supplemental Fig. S6D) and thus is not essential to the interaction with PbE3-2. Split-LUC complementation imaging assays were performed to further verify the interactions between RD21A constructs and PbE3-2. All 3 forms of RD21A exhibited fluorescence (Supplemental Figs. S6B and S5C), indicating their interactions with PbE3-2 in vivo.
PbE3-2 ligase ubiquitinates RD21A
As PbE3-2 is an E3 ubiquitin ligase and interacts with RD21A, we investigated whether RD21A is a substrate of PbE3-2. We incubated recombinant AtUBA1-S (E1), PbE3-2-Myc, RD21A-HA, AtUBC8-S (E2), and His-FLAG-UBQ10 (ubiquitin) and tested for ubiquitination. The results revealed that RD21A was ubiquitinated by PbE3-2 with a laddering pattern and could not be ubiquitinated in the absence of any component of ubiquitin, E1, E2, or PbE3-2 (Fig. 5A). Besides, the PbE3-2 (C423S) mutant showed no self-ubiquitination and could not ubiquitinate RD21A (Fig. 5A), indicating that the cysteine residue at position 423 is essential for RD21A ubiquitination. Interestingly, the interaction of PbE3-2 and RD21A was not affected by the mutation (C423S) in the Y2H assay (Fig. 5B; Supplemental Fig. S6E), suggesting that the ubiquitin ligase activity of PbE3-2 is not required for the physical interaction between the 2 proteins. We then determined whether the other 2 forms of RD21A can be ubiquitinated by PbE3-2. The results showed that all 3 forms of RD21A, including full-length RD21A, iRD21A, and mRD21A, were ubiquitinated by PbE3-2 (Fig. 5C). Next, we investigated whether PbE3-2 facilitates RD21A degradation in plants. RD21A-FLAG was transiently coexpressed with PbE3-2-GFP or PbE3-2 (C423S)-GFP by Agrobacterium infiltration of N. benthamiana, and the protein level of RD21A-FLAG was assayed to assess whether it was degraded. RD21A showed increased protein levels when coexpressed with PbE3-2 (C423S) compared to that with PbE3-2, and the degradation of RD21A was inhibited by infiltration of the 26S proteasome degradation inhibitor MG132 (Fig. 5D). These results suggested that PbE3-2 directly ubiquitinates RD21A and results in its degradation via the 26S proteasome pathway.
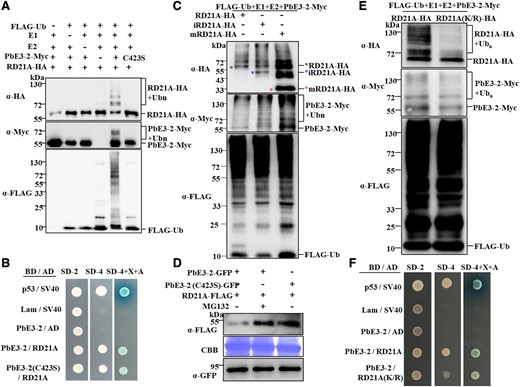
RD21A is a ubiquitinated substrate of PbE3-2 and is mediated by degradation through the 26S proteasome. A) PbE3-2 ubiquitinates RD21A whereas PbE3-2 (C423S), an enzyme active site mutant of PbE3-2, fails to ubiquitinate RD21A in the reconstituted system. B) Y2H assay shows that PbE3-2 (C423S), an enzyme active site mutant of PbE3-2, interacts with RD21A. C) RD21A, iRD21A, and mRD21A are ubiquitinated by PbE3-2 in the reconstituted system. D) PbE3-2 promotes the degradation of RD21A. Agrobacterium harboring RD21A-FLAG and PbE3-2-GFP or PbE3-2 (C423S)-GFP constructs were coinfiltrated in N. benthamiana leaves. Immunoblotting was performed with α-FLAG and α-GFP antibodies against RD21A and PbE3-2-GFP or PbE3-2 (C423S)-GFP, respectively. Protein loading was shown by Coomassie blue staining of Rubisco. E) Ubiquitination of RD21A (K/R) (13 Lys residues that identified as potential ubiquitination sites were mutated to Arg) by PbE3-2 was significantly reduced compared with wild-type RD21A. F) Y2H assay showing that RD21A(K/R), a ubiquitination site mutant of RD21A, interacts with PbE3-2. All experiments in Fig. 5 were repeated 3 times with similar results.
RD21A ubiquitination sites
Since RD21A is ubiquitinated by PbE3-2, we predicted and identified the ubiquitination sites in RD21A protein sequences. We purified the ubiquitin-conjugated RD21A protein and identified the ubiquitination sites by MS analysis and identified 13 lysine sites (Supplemental Table S3). We then generated a dominant-negative ubiquitin mutant RD21A (K/R) with the substitution of the 13 predicted Lys (K) residues by Arg (R). As a result, the ubiquitination of RD21A (K/R) by PbE3-2 was substantially reduced compared with wild-type RD21A (Fig. 5E). To check whether some potential ubiquitination sites but not all of them are important for RD21A ubiquitination, we generated 4 single-site mutations, 1 double-site mutation, and 2 triple-site mutations of RD21A at K69, K260, K261, and K321, respectively. As shown in Supplemental Fig. S7, these mutations did not substantially reduce RD21A ubiquitination by PbE3-2 compared with wild-type RD21A, suggesting that RD21A may be polyubiquitinated by PbE3-2 at multiple Lys sites. We suspect that some of the potential ubiquitination sites are more important, but it is difficult to confirm at present. However, the interaction between PbE3-2 and RD21A (K/R) in yeast cells was not affected (Fig. 5F; Supplemental Fig. S6E). These results suggested that the 13 Lys residues in RD21A play an essential role in ubiquitination.
RD21A mediates plant disease resistance
To test whether RD21A contributes to resistance against P. brassicae, Col-0 and rd21a mutant plants were inoculated with P. brassicae for 21 d. The noninoculated rd21a mutant showed no morphological difference compared to Col-0 (Supplemental Fig. S2A). However, the inoculated rd21a mutant exhibited much more severe symptoms than Col-0 with yellow leaves and severe galling, while the control plants showed galls with lateral roots (Fig. 6A; Supplemental Fig. S2D). The percentage of severe galls (disease levels of 4 and 5) was 53% in Col-0 and 91% in rd21a. The disease index was 67.2 for Col-0 while 87.0 for rd21a (Fig. 6B). Quantification of P. brassicae biomass showed a significantly higher amount of P. brassicae in rd21a mutants than in Col-0 (Fig. 6, C and D). These results indicated that rd21a has reduced resistance to P. brassicae compared to Col-0. Besides, rd21a was more susceptible to B. cinerea than Col-0 (Supplemental Fig. S2, E and F). Since it is already known that PbE3-2 suppresses host defense response (Fig. 3), we tested whether RD21A mediates the defense response by comparing the ROS production, transcript levels of defense marker genes, and SA accumulation (Figs. 6, E and F, and 3D). Comparative analysis of chitin-triggered ROS burst, SA signaling pathway marker gene expression and SA accumulation revealed that they were substantially reduced in the rd21a mutant than in Col-0. However, there was no discernible difference between Col-0 and rd21a in the chitin-triggered MAPK activation (Supplemental Fig. S3B). Taken together, the PbE3-2 transgenic plants and rd21a mutant had consistent disease susceptible phenotype and suppression of defense response, suggesting that PbE3-2 alters host defense responses by degrading plant RD21A protein through the 26S proteasome pathway, thereby promoting pathogen infection.
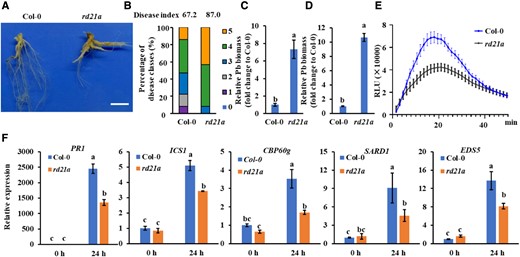
Rd21a mutants exhibit susceptibility to pathogens and reduced levels of immunity. A) Phenotype of Col-0 and rd21a at 21 d after P. brassicae inoculation. Bar: 1 cm. B) Disease index of Col-0 and rd21a. The percentages of plants in the individual disease classes are shown; Col-0 and rd21a used 35 to 40 plants for analysis. C, D) Quantification of P. brassicae content in diseased roots of Arabidopsis by qPCR, and the vertical coordinates indicate the multiplicity of P. brassicae content in different lines relative to Col-0. The relative biomass of P. brassicae is represented by the content of the P. brassicae ACTIN gene relative to that of the Arabidopsis ACTIN2 gene C) and the content of the P. brassicae DC1 gene relative to that of the Arabidopsis GAPDH gene D). n = 3 biological replicates. A significant difference was determined by Student's t-test (2-tailed). Different letters (a and b) indicate significant differences. Data are shown as mean ± Sd. E) Chitin-induced ROS burst is suppressed in rd21a mutant. The values represent means ± Se. n = 16 biological replicates. F) Induction of salicylate pathway genes was compromised in rd21a mutant. Leaves of 4-wk-old plants were infiltrated with 20-μg/mL chitin for 24 h. Data were normalized to ACTIN2 expression in qPCR analysis. Statistical analysis was performed by 1-way ANOVA with Kruskal–Wallis test (significance set at P ≤ 0.05). Different letters (a, b, and c) indicate significant differences. n = 3 biological replicates; data are shown as mean ± Sd. Experiments in Fig. 6 were repeated 3 times with similar results.
Role of PbE3s in P. brassicae pathogenesis
We next tested whether PbE3-3 and PbE3-4 perform the same function as PbE3-2. As a result, both of them were capable of self-ubiquitination (Supplemental Fig. S8A). In addition, PbE3-3 and PbE3-4 can suppress cell death induced by BAX in N. benthamiana (Supplemental Fig. S8B), suggesting that PbE3-3 and PbE3-4 can suppress plant immunity during the infection of host plants. In addition, PbE3-3 and PbE3-4 associate with RD21A in a Y2H assay (Supplemental Figs. S8D and S6E). Interestingly, in the reconstituted ubiquitination system in E. coli cells, PbE3-3 and PbE3-4 also ubiquitinated RD21A (Supplemental Fig. S8C). RD21B is a homolog of RD21A with a 78.9% similarity at the amino acid level (Supplemental Fig. S9A) and also interacts with PbE3-2 (Fig. 4F; Supplemental Fig. S6E); therefore, they may be functionally redundant. To confirm this possibility, we determined whether RD21B is the substrate of these E3 ubiquitin ligases. As expected, we observed the ubiquitination of RD21B by PbE3-2 (Supplemental Fig. S9B).
Discussion
Plants have evolved complex innate immune systems to defend against various types of pathogens (Zhou and Zhang 2020). During infection, many eukaryotic biotrophic plant pathogens have evolved secretory proteins that in some cases avoid the immune perception and/or suppress immune responses to ensure successful infection (Toruno et al. 2016). P. brassicae is an obligate intracellular plant pathogen and has developed numerous effectors to promote infection (Pérez-López et al. 2018, 2020). Overexpression of P. brassicae effector PbBSMT in Arabidopsis promoted methyl-SA production and caused susceptibility to pathogens (Bulman et al. 2019). Overexpression of P. brassicae effector PBZF1 disrupted the expression of Arabidopsis SnRK1.1 regulation gene and enhanced P. brassicae virulence (Chen et al. 2021). Ubiquitination is involved in regulation of almost all cellular processes in eukaryotes. Many bacterial pathogens exploit host ubiquitin systems by delivering E3 ubiquitin ligase effector proteins or mimicking the E3 ubiquitin ligase to achieve successful infection (Ashida and Sasakawa 2017). An oomycete effector Avr1b interacts with soybean E3 ubiquitin ligase GmPUB1 to modulate host immunity (Li et al. 2021). A rice blast fungus effector avrpiz-t targets the RING-type E3 ubiquitin ligase APIP6 to suppress pathogen-associated molecular pattern-triggered immunity (Park et al. 2012). PbRING1 was predicted to be a putative effector and display E3 ligase activity in vitro of P. brassicae in a previous study (Schwelm et al. 2015; Yu et al. 2019). In this study, PbE3-2, PbE3-3, and PbE3-4 were identified as secretory proteins, which are highly expressed in secondary infection and suppress cell death induced by BAX in N. benthamiana. These characteristics are consistent with the features of previously reported effectors (Chen et al. 2019; Pérez-López et al. 2020; Zhan et al. 2022). Hence, we suspect that they are candidate effectors. PbE3-2, PbE3-3, and PbE3-4 all harbor the RING domain at their C-terminus, which was found to have E3 ligase activity in vitro. Besides, PbE3-2, PbE3-3, and PbE3-4 can ubiquitinate RD21A and therefore may have the same biological functions. Bimolecular fluorescence complementation (BiFC) assay and colocalization assay indicated PbE3-2 interacts with RD21A in the plasma membrane and cytoplasm (Fig. 4F; Supplemental Fig. S4). Ubiquitination of RD21A by PbE3-2 may lead to poor fluorescence signals were detected in the BiFC assay (wild-type PbE3-2 and RD21A) and subcellular colocalization assay. Overall, our results suggested that P. brassicae secretes E3 ubiquitin ligases, taking the cruciferous cysteine protease RD21 as a virulence target and mediating its degradation to suppress plant immunity. Therefore, we propose a working model to summarize E3 ubiquitin ligases and RD21A-mediated immunity (Fig. 7). Here, we report on the functions of E3 ubiquitin ligase effectors in the pathogenesis of the mysterious protist P. brassicae.
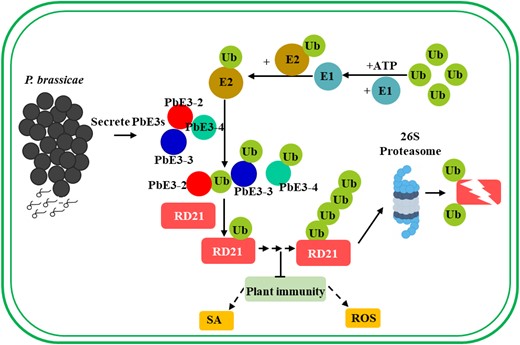
Working model of the mechanism for PbE3 to regulate plant immunity. E3 ubiquitin ligases secreted by P. brassicae directly target host cysteine protease RD21, leading to its degradation via the ubiquitin–proteasome pathway. Degradation of RD21, a positive regulator of plant immunity, leads to a reduction in the level of plant immunity and promotes infestation of P. brassicae. ROS, reactive oxygen species; SA, salicylic acid; Ub, ubiquitin.
RD21A was initially recognized as a drought-inducible gene (Koizumi et al. 1993). With the progress of relevant research, RD21A was found to play important roles in many biochemical processes, including plant immunity (Davies et al. 2015; Rustgi et al. 2017). RD21A is also involved in disease resistance of plants, including resistance to bacteria, fungi, and nematodes, and its mutation increases plant susceptibility (Shabab et al. 2008; Lozano-Torres et al. 2012; Shindo et al. 2012; Pogorelko et al. 2019; Liu et al. 2020). Proper RD21A homeostasis is critical as excessive accumulation of RD21A can lead to autoimmunity, while insufficient accumulation of RD21A may result in susceptibility to pathogens (Lampl et al. 2013; Boex-Fontvieille et al. 2015). Therefore, the level of RD21A in plant cells is tightly regulated by various biochemical reactions. In this study, we confirmed that knocking out rd21a resulted in higher susceptibility to P. brassicae (Fig. 6). ROS production and transcript levels of defense marker genes in the chitin-triggered immune responses substantially decreased in the rd21a plants than that in Col-0 (Fig. 6). Many biotrophic and necrotrophic plant pathogens regulate PTI by manipulating plant SA metabolism (Han and Kahmann 2019; Chen et al. 2013). Application of exogenous SA suppresses clubroot disease in host, and SA-deficient mutants are more susceptible to the disease than the wild type (Chen et al. 2016). To counteract SA-related defenses, P. brassicae produces a methyltransferase effector PbBSMT to reduce SA content in the host cell and facilitate colonization and lead to clubroot disease (Ludwig-Müller et al. 2015; Djavaheri et al. 2019). In PbE3-2 transgenic plants, the marker genes of the SA signaling pathway were suppressed and SA accumulation decreased under chitin treatment (Fig. 3). We suspect that results in susceptibility to P. brassicae. Although S. sclerotiorum is a necrotrophic fungal pathogen, at the early stages of infection, it grows in the apoplast without crossing the plant cell wall and the host cells are still alive at that stage (Kabbage et al. 2015). The defense against S. sclerotiorum in Arabidopsis and oilseed rape is also associated with SA signaling, and SA signaling pathway possibly acts as a positive regulator in plant immunity at the early stage of S. sclerotiorum infection (Yang et al. 2018; Tang et al. 2020). We speculate that the level of SA may be one of the reasons for the susceptibility of PbE3-2 transgenic plants to S. sclerotiorum. Besides, RD21A played a crucial role in disease immunity, including resistance to fungi, nematodes, and bacteria. However, the mechanism by which RD21A regulates plant disease resistance is not well understood. In addition to SA, there may be ROS or other phytohormone, etc. In this study, PbE3-2 ubiquitinated and degraded RD21A, and the rd21 mutant substantially decreased SA accumulation, which might contribute to the higher susceptibility of PbE3-2 transgenic plants to the necrotrophic fungal pathogens than Col-0. It is necessary to clarify how RD21A manipulates plant SA metabolism will improve the understanding of the interaction between P. brassicae and the host. In addition, we still did not address whether PbE3-2-mediated immune responses defect dependent on RD21A. Because of the parasitic nature of the P. brassicae, it is currently almost impossible to obtain a knockout mutant of PbE3-2.
RD21A can be ubiquitinated by PbE3-2, PbE3-3, and PbE3-4 in vitro (Fig. 5; Supplemental Fig. S8). RD21B, a homolog of RD21A, can be ubiquitinated by PbE3-2 (Supplemental Fig. S9). All these results suggest the ubiquitination of RD21 by secreted E3 ubiquitin ligase in P. brassicae. In previous reports, RD21A level was also regulated by the plant ubiquitination system (Kim and Kim 2013). RD21A can interact with the ubiquitin E3 ligase SINAT4 to participate in drought-induced immunity (Liu et al. 2020). Different from previous reports, we identified that pathogen secreted E3 ligase to regulate the RD21A level. We also identified 13 potential ubiquitination sites on RD21A and generated the RD21A ubiquitination site mutant RD21AK/R. The ubiquitination of RD21AK/R by PbE3-2 was much less substantial than that of wild-type RD21A. However, it is interesting to identify the real ubiquitination sites. Unfortunately, the 7 generated mutations resulted in no substantial reduction in the ubiquitination of RD21A compared with RD21AK/R. It is possible that some of them are important ubiquitination sites, but they are difficult to be identified as there are many combinations. It remains to be determined whether substituting arginine for the 13 Lys residues of RD21A would perturb its degradation in vivo in future work. The PLCPs have important, complex and not fully understood functions for the regulation of plant immunity. PLCPs contribute to plant resistance to widespread pathogens. Tomato immune proteases RCR3 and PIP1 are involved in resistance to fungal and oomycete pathogens (Rooney et al. 2005; Kaschani et al. 2010; Paulus et al. 2020). Maize immune signaling peptide 1 (Zip1) is released by PLCP and can strongly trigger the accumulation of SA in leaves (Ziemann et al. 2018). OsXCP2, a PLCP in rice, mediates resistance against Xanthomonas oryzae by elevating SA levels and ROS accumulation (Niño et al. 2020). In addition, protease could split microbial peptides to trigger immune responses (Wang et al. 2021). Since PLCP plays an important role in plant immunity, many pathogens secrete effector to target PLCP. Huanglongbing-associated pathogen secreting SDE1 directly interacts with citrus PLCP and inhibits its protease activity (Clark et al. 2018). P. syringae secretes CIP1 to inhibit tomato immune-associated protease thereby promoting virulence (Shindo et al. 2016). The cyst nematode effector Hs4E02 targets plant defense protease and achieves virulence by altering its subcellular localization (Pogorelko et al. 2019). In the present study, we identified P. brassicae directly targets the plant defense-associated cysteine protease RD21A to promote virulence, which increases our understanding of the pathogenesis of protist. Therefore, future studies of how RD21A regulates plant resistance to P. brassicae are of relevance. In the future, RD21A may be genetically edited to help plants breed against clubroot disease.
In summary, our results suggest that the cysteine protease RD21 is a positive regulator of plant immunity and that P. brassicae secretes E3 ubiquitin ligases to directly target RD21 for degradation, thereby weakening plant immunity for successful infection. This study may help the generation of germplasm with higher clubroot resistance in the future.
Materials and methods
P. brassicae inoculation
P. brassicae strain ZJ-1 was isolated by Chen et al. (2016). P. brassicae resting spores were collected from the galls and kept at 4 °C. One milliliter of 1.0 × 107 spores/mL resting spore suspension was inoculated onto 14-d-old Arabidopsis (A. thaliana) seedlings through the soil around the roots, followed by 21 dpi analysis. A modified 0 to 5 scoring system from previous studies was used to assess disease severity (Siemens et al. 2012): 0, no disease; 1, extremely little galls primarily on lateral roots, with negligible harm to main roots; 2, the main roots and a few lateral roots were covered in tiny galls; 3, main root with medium to large globules; 4, severe galls on lateral roots, main roots or rosettes, with total fine root damage on lateral roots; and 5, completely expansion and rotting of roots.
Plants and growth conditions
The Arabidopsis and N. benthamiana plants were grown in greenhouse at 12-h day, 12-h night, 22 °C, and 60% relative humidity. The rd21a mutant was obtained using the CRISPR/Cas9 method in a Col-0 background (Liu et al. 2020). PbE3-2 transgenic A. thaliana line was generated by using Agrobacterium (A. tumefaciens)-mediated transformation by floral dipping (Clough and Bent 1998).
Yeast invertase secretion assay
PbE3-2-SP was cloned into the vector pSUC2. The pSUC2, pSUC2-avr1b-SP (positive control), and pSUC2-PbE3-2-SP fusion vectors were transformed into yeast (S. cerevisiae) strain YTK12. The transformants were grown on CMD-W (0.67% [w/v] yeast N base without amino acids, Trp dropout supplement, 2% [w/v] sucrose, 0.1% [w/v] glucose, and 2% [w/v] agar) or YPRAA medium (1% [w/v] yeast extract, 2% [w/v] peptone, 2% [w/v] raffinose, 2-μg/L antimycin A, and 2% [w/v] agar) for invertase secretion assays. Invertase enzymatic activity was detected by the reduction of 2,3,5-triphenyltetrazolium chloride (TTC) to red-colored insoluble triphenylformazan.
Apoplastic fluid assay
Extraction of plasmatic extracellular fluid was conducted according to a previous protocol (O'Leary et al. 2014). Sterile water was infiltrated by a vacuum pump into the leaves of N. benthamiana with transient expression of PbE3-2 or PbE3-2ΔSP protein. The apoplast washing fluid was recovered by centrifugation and concentrated for western blotting to verify that proteins were secreted into the apoplast.
Confocal microscopy assay
For subcellular localization or BiFC assay, A. tumefaciens strain GV3101 carrying the desired constructs was infiltrated into N. benthamiana leaves; GFP, YFP, or RFP fluorescence signals were examined at 2 to 3 dpi with confocal microscopy (Nikon, A1HD25). GFP or YFP was detected after excitation with a 488-nm wavelength laser, and emissions were collected between 500 and 550 nm. RFP was detected after excitation with a 561-nm wavelength laser, and emissions were collected between 570 and 620 nm. Laser intensity is in the range of 2.0 to 3.0, and gain is in the range of 50 to 80.
Ubiquitination assay
Ubiquitination assay was performed by reconstituted ubiquitination reactions in E. coli (Han et al. 2017). Arabidopsis ubiquitin UBQ10 was constructed into the prokaryotic expression vector pET28a. AtUBA1 and RD21A were constructed into the compatible dual expression vector pCDFDuet, and AtUBC8 and PbE3-2 were constructed into the compatible dual expression vector pACYDuet. Strains lacking any of these components were used as negative controls. Self-ubiquitination reactions were performed with strains lacking the substrate. Western blot of purified proteins from crushed organisms was performed to determine the occurrence of ubiquitination according to the previous method.
Measurement of the amount of P. brassicae DNA in diseased roots
Extraction of DNA from infected roots was performed using the cetyltrimethylammonium bromide method (Allen et al. 2006). qPCR was performed as described by Chen et al. (2016). The relative biomass of P. brassicae is represented by the content of the P. brassicae ACTIN gene (AY452179.1) relative to that of the Arabidopsis ACTIN2 gene (AT3G18780), or the content of P. brassicae is represented by the content of the P. brassicae DC1 gene from a partial 18S rRNA gene sequence (AF231027) relative to that of the Arabidopsis GAPDH gene (AT1G13440) (Rennie et al. 2011). The qPCR primers used are listed in Supplemental Table S4.
ROS assay and MAPK assay
ROS assay and MAPK assay were conducted as previously described (Qi et al. 2022). Arabidopsis leaf discs from 4-wk-old plants after overnight incubation with ddH2O were treated with 100-μL solution containing 5 μm L-012 (Wako, 120-04891, Japan), 10-μg/mL horseradish peroxidase (Sigma, P6782, USA), and 20-μg/mL chitin. ROS production was measured using a Multimode Reader Platform (Tecan Austria GmbH, SPARK 10M) with a reading interval of 1.5 and 50 min per treatment. Five leaf discs were transferred to 6-well plates with H2O overnight and then treated with 20-μg/mL chitin for 5, 15, or 30 min, respectively. Used α-pErk1/2 antibody (Cell Signaling Technology, 9101, USA) to detect the level of pMPK3 and pMPK6.
IP and LC-MS analysis
The IP experiment was performed as previously described (Yang et al. 2018), using IP buffer (Beyotime, P0013, China) to extract the total protein from PbE3-2-GFP transgenic plants. About 50 mL of protein extract was incubated with 100 μL of α-GFP agarose beads (ChromoTek, gta-20, Germany) for 12 h at 4 °C. Beads were collected by centrifugation at 1,000 × g for 1 min at 4 °C, washed with 1 mL of IP buffer 3 times, and boiled for 5 min to elute the bound proteins from the beads. The proteins were sent to HOOGEN BIOTECH Company (Shanghai, China) for LC-MS analysis (Q Exactive, Thermo Fisher).
Y2H system
The cDNA encoding the PbE3-2 without signal peptide was cloned into pGBKT7 vector, and the candidate proteins identified from LC-MS were constructed into pGADT7. Both plasmids were transformed into Y2H golden yeast cells using polyethylene glycol/LiAc-mediated yeast transformation. The transformation mix was spread on SD medium–Leu–Trp (SD-2, selects for both plasmids), and positive clones were further confirmed on SD medium–Trp–Leu–His–Ade (SD-4) containing X-α-Gal and aureobasidin A.
Co-IP assay
Transient expression of RD21A-FLAG with PbE3-2-GFP or GFP was performed in N. benthamiana by Agrobacterium infiltration. Extraction of total proteins with IP buffer was followed by IP with α-GFP agarose beads (ChromoTek, gta-20, Germany) at 4 °C for 1 h. Beads were collected, washed 3 times with IP buffer, and immunoblotted with α-FLAG antibody.
Split-LUC complementation assay
The split-LUC reconstitution assay was carried out as previously reported (Chen et al. 2008). Different N-terminal luciferase (nLUC) or C-terminal luciferase (cLUC) constructs were introduced into A. tumefaciens strain GV3101. Agrobacterium suspension (OD600 = 1.5) carrying the specified nLUC and cLUC plasmids was coinfiltrated into 4-wk-old N. benthamiana leaves. About 1 mm luciferin (YEASEN, 40901ES01, China) was infiltrated 48 h after infiltration, and luminescence was imaged within 5 min using a charge-coupled device imaging apparatus.
Western blots
Western blot was carried out as previously described (Chen et al. 2012). Proteins were separated by 10% SDS–PAGE and transferred to 0.2-μm PVDF membranes for 1 h. In each experiment, a parallel gel was stained with Coomassie brilliant blue as a loading control. The following antibodies were used: mouse anti-FLAG (Sigma Aldrich) at a 1:2,000 dilution, mouse anti-GFP (ABclonal) at a 1:2,000 dilution, rabbit anti-HA (ABclonal) at a 1:2,000 dilution, mouse anti-GST (ABclonal) at a 1:2,000 dilution, mouse anti-His (ABclonal) at a 1:2,000 dilution, mouse anti-Myc (Genscript) at a 1:2,000 dilution, HRP-conjugated anti-rabbit IgG secondary antibodies (ABclonal) at a 1:10,000 dilution, and HRP-conjugated anti-mouse IgG secondary antibodies (ABclonal) at a 1:10,000 dilution.
SA extraction and quantification
SA was extracted and quantified as previously described with some modifications (Huot et al. 2017). Four-week-old Arabidopsis leaves were infiltrated with 20 μg/mL of chitin or water, and 24 h after infiltration, leaf tissue between 50 and 100 mg (fresh weight) was flash-frozen in liquid nitrogen, ground, and extracted at 4 °C with extraction buffer. Filtered extracts were quantified using an ultrafast LC-electrospray ionization tandem MS as previously described (Liu et al. 2012).
Accession numbers
Sequence data from this article can be found in the GenBank/EMBL data libraries under accession numbers: PbE3-1, ON394060; PbE3-2, ON394061; PbE3-3, ON394062; PbE3-4, ON394063; PbE3-5, ON394064; RD21A, NP_564497.1; RD21B, NP_568620.1; RD19, NP_568052.1; RD20, NP_180896.1; CP1, NP_567376.1; BnRD21A1, CDY17044.1; BnRD21A2, CDY17093.1; and BnRD21A3, CDY06760.1.
Acknowledgments
We thank Dr. Yi Liu from the Chinese Academy of Sciences for the seeds of rd21a mutant. We thank Dr. Dongping Lu from the Institute of Genetics and Developmental Biology for sharing the in vitro ubiquitination assay system. We thank Dr. Yangrong Cao from Huazhong Agricultural University for sharing the split-LUC vectors. We thank Dr. Shuai Fang and Hongbo Liu for providing technical support on SA quantification. We thank Dr. Kenichi Tsuda, Shengyang He, and Kabin Xie for their critical reading of the manuscript and constructive suggestions. We thank research associates at the Center for Protein Research (CPR), Huazhong Agricultural University, for the technical support. We would like to thank the State Key Laboratory of Agricultural Microbiology Core Facility for the assistance in confocal microscopy.
Author contributions
C.L. and T.C. conceived the project and designed experiments. C.L. performed most of the experiments. C.L., S. L., L.F., and Q. W. performed experiments and analyzed the data. J.C., J.X., Y.L., Y.F., and D.J. analyzed the data and provided advice that helped shape the research. C.L. and T.C. wrote the manuscript with input from all coauthors.
Supplemental data
The following materials are available in the online version of this article.
Supplemental Figure S1. Analysis of secreted PbE3s.
Supplemental Figure S2. Growth phenotype of Col-0, PbE3-2 overexpression plants, and rd21a mutant.
Supplemental Figure S3. Chitin triggered RBOHD gene expression and MAPK activation in PbE3-2 overexpression lines.
Supplemental Figure S4. BiFC on the interaction between PbE3-2 with RD21A in planta, subcellular localization of PbE3-2, and subcellular colocalization of PbE3-2 and RD21A.
Supplemental Figure S5. PbE3-2 interacts with B. napus BnRD21A.
Supplemental Figure S6. PbE3-2 interacts with truncated RD21A.
Supplemental Figure S7. Detection the ubiquitination sites of RD21A by PbE3-2.
Supplemental Figure S8. PbE3-2, PbE3-3, and PbE3-4 interacted with RD21, respectively.
Supplemental Figure S9. PbE3-2 ubiquitinate RD21A and RD21B, respectively.
Supplemental Table S1. The information of E3 ubiquitin ligases in the genome of P. brassicae ZJ-1.
Supplemental Table S2. List of potential PbE3-2 interacting partners identified by IP-MS/MS analysis.
Supplemental Table S3. Ubiquitination sites by LC-MS.
Supplemental Table S4. Primers used in the study.
Funding
This research was financially supported by the National Natural Science Foundation of China (32172371), the Fundamental Research Funds for the Central Universities (2662023PY006), the earmarked fund of China Agriculture Research System (CARS-12), and the collaborative fund of Huazhong Agricultural University and Agricultural Genomics Institute at Shenzhen (SZYJY2021007).
Data availability
The data are available on request from the corresponding author.
Dive Curated Terms
The following phenotypic, genotypic, and functional terms are of significance to the work described in this paper:
References
Author notes
The author responsible for distribution of materials integral to the findings presented in this article in accordance with the policy described in the Instructions for Authors (https://dbpia.nl.go.kr/plphys/pages/General-Instructions) is Tao Chen.
Conflict of interest statement. None declared.