-
PDF
- Split View
-
Views
-
Cite
Cite
Lydia Pui Ying Lam, Yuki Tobimatsu, Shiro Suzuki, Takuto Tanaka, Senri Yamamoto, Yuri Takeda-Kimura, Yuriko Osakabe, Keishi Osakabe, John Ralph, Laura E Bartley, Toshiaki Umezawa, Disruption of p-coumaroyl-CoA:monolignol transferases in rice drastically alters lignin composition, Plant Physiology, Volume 194, Issue 2, February 2024, Pages 832–848, https://doi.org/10.1093/plphys/kiad549
- Share Icon Share
Abstract
Grasses are abundant feedstocks that can supply lignocellulosic biomass for production of cell-wall-derived chemicals. In grass cell walls, lignin is acylated with p-coumarate. These p-coumarate decorations arise from the incorporation of monolignol p-coumarate conjugates during lignification. A previous biochemical study identified a rice (Oryza sativa) BAHD acyltransferase (AT) with p-coumaroyl-CoA:monolignol transferase (PMT) activity in vitro. In this study, we determined that that enzyme, which we name OsPMT1 (also known as OsAT4), and the closely related OsPMT2 (OsAT3) harbor similar catalytic activity toward monolignols. We generated rice mutants deficient in either or both OsPMT1 and OsPMT2 by CRISPR/Cas9-mediated mutagenesis and subjected the mutants’ cell walls to analysis using chemical and nuclear magnetic resonance methods. Our results demonstrated that OsPMT1 and OsPMT2 both function in lignin p-coumaroylation in the major vegetative tissues of rice. Notably, lignin-bound p-coumarate units were undetectable in the ospmt1 ospmt2-2 double-knockout mutant. Further, in-depth structural analysis of purified lignins from the ospmt1 ospmt2-2 mutant compared with control lignins from wild-type rice revealed stark changes in polymer structures, including alterations in syringyl/guaiacyl aromatic unit ratios and inter-monomeric linkage patterns, and increased molecular weights. Our results provide insights into lignin polymerization in grasses that will be useful for the optimization of bioengineering approaches for the effective use of biomass in biorefineries.
Introduction
Plant biomass is a sustainable and renewable resource for production of chemicals and materials through biorefining. Among the currently available biomass feedstocks, grasses are attracting attention because of their superior characteristics, compared with other feedstocks, in terms of the productivity and utility of lignocellulosic biomass (Umezawa 2018; Umezawa et al. 2020). For example, large-sized grass bioenergy crops, such as sugarcane (Saccharum sp. and Erianthus sp.), sorghum (Sorghum bicolor), switchgrass (Panicum virgatum), and Miscanthus feature particularly high lignocellulose productivity (Mullet et al. 2014; Tye et al. 2016; Umezawa et al. 2020). Large amounts of lignocellulose are produced globally in agricultural wastes from grain crops, such as rice (Oryza sativa), maize (Zea mays), sorghum, wheat (Triticum aestivum), and barley (Hordeum vulgare), which could also be used as lignocellulosic sources for industrial purposes (Lal 2005). Furthermore, chemical delignification of grass lignocellulose is typically easier than for woody biomass (Davis et al. 2013; Umezawa et al. 2020). To facilitate the utilization of grass lignocellulose, we need to deepen our understanding of the biosynthesis, properties, and structure of grass lignocellulose.
Lignins are complex phenylpropanoid polymers that are deposited in secondary cell walls and comprise a large portion (5% to 20%) of lignocellulose, together with cellulose and hemicelluloses. In general, lignin forms by the polymerization of p-hydroxycinnamyl alcohols (monolignols), mainly sinapyl alcohol (S-OH) and coniferyl alcohol (G-OH), and also p-coumaryl alcohol (H-OH) to a lesser extent (Freudenberg 1965; Sarkanen and Ludwig 1971; Ralph et al. 2004, 2019). These monolignols are synthesized via the cinnamate/monolignol pathway through a series of enzymatic reactions (Umezawa et al. 2020; Dixon and Barros 2019) (Fig. 1). In cell walls, monolignols are oxidized by laccases and/or peroxidases and polymerize via radical coupling to generate the syringyl (S), guaiacyl (G), and p-hydroxyphenyl (H) polymer units from S-OH, G-OH, and H-OH, respectively (Ralph et al. 2019; Tobimatsu and Schuetz 2019).
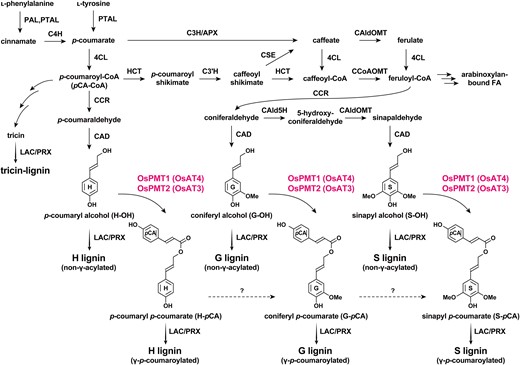
Proposed lignin biosynthetic pathways in rice. Solid arrows indicate discreet enzymatic reactions. Dashed arrows indicate hypothesized reactions. The two rice PMTs (OsPMT1 and OsPMT2) investigated in this study are highlighted. PTAL, ʟ-phenylalanine/ʟ-tyrosine ammonia-lyase; PAL, ʟ-phenylalanine ammonia-lyase, C4H, cinnamate 4-hydroxylase; 4CL, 4-hydroxycinnamate:CoA ligase; C3H, p-coumarate 3-hydroxylase; APX, ascorbate peroxidase; HCT, p-hydroxycinnamoyl-CoA:quinate/shikimate hydroxycinnamoyl transferase; C3′H, p-coumaroyl ester 3-hydroxylase; CSE, caffeoyl shikimate esterase; CCoAOMT, caffeoyl-CoA O-methyltransferase; CAld5H, coniferaldehyde 5-hydroxylase; CAldOMT, 5-hydroxyconiferaldehyde O-methyltransferase; CCR, cinnamoyl-CoA reductase; CAD, cinnamyl alcohol dehydrogenase; PMT, p-coumaroyl-CoA:monolignol transferase; AT, acyltransferase; LAC, laccase; PRX, peroxidase.
Unlike lignin in other major vascular plant groups (e.g. eudicots and gymnosperms), grasses incorporate diverse lignin monomers in addition to the common monolignols. For example, grasses incorporate tricin, a flavonoid, as a monomer for lignification. Tricin undergoes oxidative radical coupling with monolignols and their derivatives, resulting in tricin–lignin units in lignin polymers (del Río et al. 2012; Lan et al. 2015, 2016a; Lam et al. 2021) (Fig. 1).
Another structural feature of grass lignin is its acylation by phenolic acids, mainly p-coumarate (pCA) (Smith 1955; Higuchi et al. 1967; Ralph et al. 1994; Lu and Ralph 1999; Hatfield et al. 2009; Ralph 2010) and also ferulate (FA), albeit at much lower levels (Karlen et al. 2016; Ralph et al. 2019; Chandrakanth et al. 2023). pCA and FA decorations occur exclusively on the γ-hydroxyl groups of phenylpropanoid units and are usually more abundant on S units than G units (Grabber et al. 1996; Morrison et al. 1998; Lu and Ralph 1999; Ralph 2010). Lignin p-coumaroylation takes place at the monomer stage (Ralph 2010). Monolignols (S-OH and G-OH) may be first γ-acylated via p-coumaroyl-CoA (pCA-CoA), generating monolignol-pCA conjugates, i.e. sinapyl p-coumarate (S-pCA) and coniferyl p-coumarate (G-pCA), respectively (Withers et al. 2012). The occurrence of the H-type monolignol-pCA conjugate, i.e. p-coumaryl p-coumarate (H-pCA) from H-OH, in lignification has also been described in some plant species, albeit at very low levels (Karlen et al. 2018; Takeda et al. 2018). Monolignol-pCA conjugates can diffuse or be transported to the cell walls and participate in radical coupling with monolignols and tricin to form p-coumaroylated lignin (Ralph et al. 1994; Grabber et al. 1996; Lu and Ralph 1999; Ralph 2010; Lan et al. 2016b) (Fig. 1). In addition to ubiquitous occurrence in grasses, p-coumaroylated lignin is distributed in other families of commelinid monocots (Karlen et al. 2018) and some eudicots, e.g. Morus (mulberry) (Hellinger et al. 2023) and kenaf (Hibiscus cannabinus) (Mottiar et al. 2023).
In grasses, so-called “BAHD” CoA-acyltransferases (ATs) catalyze the acylation of monolignols and the matrix polysaccharide arabinoxylan via pCA-CoA and feruloyl-CoA (Withers et al. 2012; Marita et al. 2014; Petrik et al. 2014; Chandrakanth et al. 2023) (Fig. 1). In rice, an important crop and model grass, a BAHD p-coumaroyl-CoA:monolignol transferase (PMT), OsPMT1 (OsAT4 in the nomenclature of Bartley et al. 2013) has been shown to harbor PMT catalytic ability in vitro (Withers et al. 2012; Smith et al. 2022b). In addition, heterologous expression of OsPMT1 in the eudicots Arabidopsis (Arabidopsis thaliana) and poplar (Populus sp.), which do not naturally biosynthesize monolignol-pCA conjugates, resulted in formation of p-coumaroylated lignin (Smith et al. 2015; 2022a). Other genetic and biochemical experiments in rice and other grasses have provided evidence that other BAHD ATs esterify monolignols with FA (OsFMT or OsAT5; Karlen et al. 2016) or arabinoxylan with pCA (OsAT10; Bartley et al. 2013; Möller et al. 2022). Still, the in planta roles of OsPMT1 in rice lignin p-coumaroylation have not been conclusively demonstrated through analysis of transgenic or mutant rice plants.
Here, we demonstrate that OsPMT1 and the closely related homolog, OsPMT2 (OsAT3), cooperatively act as the major PMTs involved in lignin p-coumaroylation in rice. We demonstrated that, similarly to OsPMT1, OsPMT2 catalyzes the formation of monolignol-pCA conjugates in vitro. We then investigated the in planta functions of OsPMT1 and OsPMT2 through cell wall structural analysis of genome-edited rice mutants deficient in OsPMT1 and OsPMT2. Lignin-bound pCA units were indetectable in the stem/culm of double mutants that also had substantial changes in lignin polymer structure and molecular weight.
Results
OsPMT1 and OsPMT2 as putative PMTs in rice
The in planta function of BdPMT1 as the predominant PMT involved in lignin p-coumaroylation in B. distachyon has previously been demonstrated by cell wall analysis of the bdpmt1 mutant (Petrik et al. 2014), and by biochemical analysis of recombinant BdPMT1 in vitro (Smith et al. 2022b). Among the rice BAHD ATs implicated in cell wall modification (Mitchell et al. 2007; Bartley et al. 2013), OsPMT1 (OsAT4, encoded by LOC_Os01g18744) and OsPMT2 (OsAT3, encoded by LOC_Os05g04584) possessed the highest identities to BdPMT1 with 61.6% and 74.7% protein sequence identities, respectively. Phylogenetic analysis of related grass BAHD AT proteins showed that OsPMT2 clusters with BdPMT1, and also with a maize p-coumaroyl CoA:hydroxycinnamyl alcohol transferase, ZmpCAT, sorghum SbPMT, and switchgrass PvPMT (Fig. 2A), which have also been implicated in lignin p-coumaroylation (Marita et al. 2014; Smith et al. 2022b). In contrast, putative orthologs of OsPMT1 appear to be absent in B. distachyon and maize but are present in other related grass species including switchgrass. The OsPMT1 and OsPMT2 clades are relatively distant from the other distinct clades that contain different rice BAHD ATs, such as OsFMT/OsAT5, which has been characterized as a feruloyl-CoA:monolignol transferase (Karlen et al. 2016; Smith et al. 2022b), OsAT8 with a yet unknown function but homologous to BdPMT2 (an enzyme that possesses PMT activity when heterologously expressed in Arabidopsis) (Sibout et al. 2016), OsAT9, which is homologous to arabinoxylan feruloyl transferases (feruloyl-CoA:arabinofuranose transferases, FATs) (de Souza et al. 2018), and OsAT10, which has been characterized as an arabinoxylan p-coumaroyl transferase (p-coumaroyl-CoA:arabinofuranose transferase, PAT) (Bartley et al. 2013) (Fig. 2A).
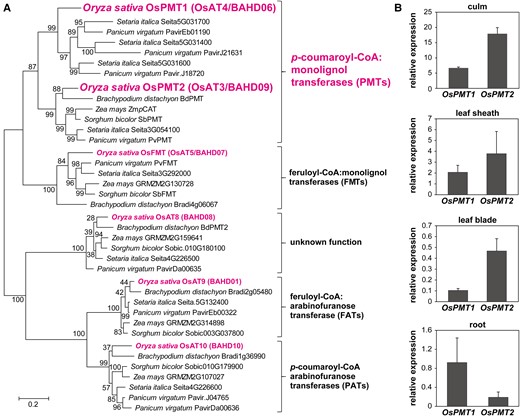
Phylogenetic and gene expression analyses of OsPMT1 and OsPMT2. A) phylogenetic reconstruction of grass BAHD acyltransferase proteins in grasses. The consensus unrooted phylogenetic tree was developed with the maximum likelihood method with 1,000 bootstrap replicates. Scale bar denotes 0.2 substitution per site. OsPMT1 and OsPMT2, and other associated rice BAHD CoA-acyltransferases (ATs) are highlighted. B) RT-qPCR-based gene expression analysis of OsPMT1 and OsPMT2 relative to OsUBQ5 in culm, leaf sheath and leaf blade at the heading stage and root at 2-month-old wild-type (cv. Nipponbare) rice. The values are means ± standard deviation from individual plants (n = 3).
In silico analysis of spatial and developmental gene expression patterns of OsPMT1 and OsPMT2 reveals that both genes are expressed in lignifying tissues. Gene atlas data retrieved from the public database (Sato et al. 2012) shows that OsPMT1 is strongly expressed in culm (i.e. stem) and root during the vegetative and reproductive stages (Supplemental Fig. S1) in which lignification typically occurs and abundant accumulation of p-coumaroylated lignin has been reported. OsPMT2 expression is the highest in culm. These PMT genes are also concurrently expressed with other known monolignol biosynthetic genes, including p-coumaroyl ester 3-hydroxylase (OsC3′H1) (Takeda et al. 2018), coniferaldehyde 5-hydroxylase (OsCAld5H1) (Takeda et al. 2017), 5-hydroxyconiferaldehyde O-methyltransferase (OsCAldOMT1) (Koshiba et al. 2013a; Lam et al. 2019), and cinnamyl alcohol dehydrogenase (OsCAD2) (Koshiba et al. 2013b).
We further analyzed the expression of OsPMT1 and OsPMT2 in the lignifying vegetative organs, including the culm, leaf sheath, leaf blade, and root, by reverse transcription quantitative PCR (RT-qPCR). The total RNA samples used for the analysis were collected from developing rice plants at the heading (culm, leaf sheath, and leaf blade) and 2-month-old growing (root) stages. The results indicated that both OsPMT1 and OsPMT2 were expressed in all these lignifying tissues. With the primer pairs used, the relative expression of OsPMT1 appeared higher than that of OsPMT2 in the root; whereas expression of OsPMT2 was higher than that of OsPMT1 in aerial vegetative organs (culm, leaf sheath, and leaf blade; Fig. 2B). According to these results, in addition to OsPMT1, we selected OsPMT2 for further investigation as a potential PMT involved in lignin p-coumaroylation.
Enzymatic assays of recombinant OsPMT1 and OsPMT2
OsPMT1 was previously shown in vitro to possess PMT activity to γ-acylate monolignols via pCA-CoA to form the corresponding monolignol-pCA conjugates (Withers et al. 2012; Smith et al. 2022b). Here, we tested the PMT catalytic activity of OsPMT2, along with OsPMT1 as a positive control (Fig. 3). Recombinant OsPMT1 and OsPMT2 proteins were expressed in Escherichia coli cells. Because several attempts to purify His6-tagged recombinant proteins retaining enzyme activity failed, we used the crude lysates of E. coli cells expressing OsPMT1 and OsPMT2 for the enzymatic assay. The reaction products from the OsPMT1 and OsPMT2 lysates with the three monolignol substrates and pCA-CoA were subjected to liquid chromatography-mass spectrometry (LC-MS). Lysates containing recombinant OsPMT1 or OsPMT2 produced the anticipated monolignol-pCA conjugates, H-pCA, G-pCA, and S-pCA, from H-OH, G-OH, and S-OH, respectively, whereas monolignol-pCA conjugates were not detected in the control reaction mixtures with heat-treated lysates (Fig. 3, B and C). Thus, similarly to OsPMT1, OsPMT2 possesses PMT activity capable of producing monolignol-pCA conjugates in vitro.
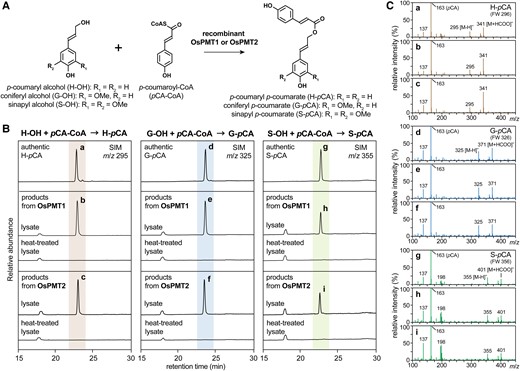
Enzymatic assays for recombinant OsPMT1 and OsPMT2 proteins. A) Enzymatic reaction for acylation of monolignols (H-OH, G-OH, and S-OH) with p-coumaroyl-CoA (pCA-CoA) into monolignol p-coumarate conjugates (H-pCA, G-pCA, and S-pCA). B and C) Detections of monolignol p-coumarate conjugates in the reaction products of the recombinant OsPMT1 and OsPMT2 lysates (without and with the heat treatment) by using liquid chromatography-mass spectrometry (LC-MS). Selected ion monitoring (SIM) chromatograms (m/z 295, 325 and 355 for H-pCA, G-pCA, and S-pCA, respectively) (B) and mass spectra (C) of authentic monolignol p-coumarate conjugate standards and reaction products from the OsPMT1 and OsPMT2 lysates are shown. The mass spectra a–i shown in (C) respectively correspond to the peaks a–i in (B). FW, formula weight.
Generation of OsPMT1- and OsPMT2-deficient rice mutants
To investigate the in planta functions of OsPMT1 and OsPMT2, rice mutants with knockout mutations of either or both OsPMT1 and OsPMT2 were generated by CRISPR/Cas9-mediated mutagenesis. To generate OsPMT1 and OsPMT2 single-knockout mutant lines, CRISPR/Cas9 binary vectors (Mikami et al. 2015) that contained single-strand guide RNAs targeting the first exon of OsPMT1 or OsPMT2 were transformed into rice calli, and regenerated plants were genotyped by a direct sequencing approach (see Supplemental Methods for details). We successfully isolated T1 individuals for an OsPMT1 single-knockout mutant line (ospmt1) harboring a 1-bp deletion in the target OsPMT1 site, and an OsPMT2 single-knockout mutant line (ospmt2-1) harboring a 1-bp insertion in the target OsPMT2 site (Supplemental Fig. S2). To generate OsPMT1 and OsPMT2 double-knockout mutant lines, we first isolated another ospmt2 homozygous mutant line harboring a 1-bp deletion in the target OsPMT2 site (ospmt2-2) but without the CRISPR/Cas9 cassette and the hygromycin-resistance gene. This nonhygromycin-resistant ospmt2-2 mutant line was further genome-edited by transforming the CRISPR/Cas9 vector targeting OsPMT1. Consequently, we isolated T1 individuals for an OsPMT1 and OsPMT2 double-knockout mutant line (ospmt1 ospmt2-2) harboring 1-bp deletions in both the target OsPMT1 and OsPMT2 sites (Supplemental Fig. S2). The genotype and zygosity of the mutant plants were confirmed by direct sequencing of PCR products and also by sequencing at least 12 E. coli colonies with constructs containing the PCR products used for direct sequencing (see Supplemental Methods for details). All the indels resulted in frameshift mutations and the formation of premature stop codons, resulting in the loss of function of OsPMT1 (Supplemental Fig. S3) and/or OsPMT2 (Supplemental Fig. S4). We also determined that there were no off-target mutations in the five top-ranked potential off-target sites in all the mutant plants used for further analysis (Supplemental Table S1).
Phenotypes of OsPMT1- and OsPMT2-deficient rice mutants
The fully genotyped ospmt1, ospmt2-1, and ospmt1 ospmt2-2 mutants and wild-type control (WT) plants were assessed for the growth performance (Supplemental Table S2). Compared with WT, ospmt1 and ospmt2-1 displayed approximately 20% and 21% reductions, respectively, in culm length, whereas ospmt1 ospmt2-2 displayed a greater culm length reduction of approximately 32%. Further, ospmt1 ospmt2-2 also displayed a significant reduction in panicle length, whereas ospmt1 and ospmt2-1 did not. There were no significant differences in the other growth parameters investigated (plant height, tiller number, and panicle number) between the mutants and WT.
Cell-wall-bound hydroxycinnamate contents in different vegetative tissues of OsPMT1- and OsPMT2-deficient rice mutants
To investigate the impact of the disruption of OsPMT1 and OsPMT2 on lignification in different rice organs, cell wall residues (CWRs) were prepared from culm, leaf sheath, leaf blade, and root of the ospmt1, ospmt2-1, and ospmt1 ospmt2-2 mutants and WT, and the cell-wall-bound hydroxycinnamate (pCA and FA) and lignin contents were measured.
Total cell-wall-bound pCA and FA levels were examined by quantifying the pCA and FA released by the mild alkaline hydrolysis of CWRs. Compared with WT, ospmt1 displayed significant reductions in the cell-wall-bound pCA content in leaf sheath (approximately 21%) and root (approximately 44%) but no significant changes in culm and leaf blade (Fig. 4A). For ospmt2-1, significant reductions in the cell-wall-bound pCA content in all the examined organs were observed, except for leaf blade, by approximately 73%, 53%, and 53% in culm, leaf sheath and root, respectively. Strikingly, ospmt1 ospmt2-2 displayed drastic reductions in the cell-wall-bound pCA content in all the examined organs, to an undetectable level in culm, and by approximately 91%, 55%, and 97% in leaf sheath, leaf blade, and root, respectively (Fig. 4A). For the cell-wall-bound FA, which is linked mainly to hemicellulosic arabinoxylan and at much lower levels to lignin (Karlen et al. 2016), we observed significant reductions in ospmt1 ospmt2-2 culms (by approximately 43%) and the ospmt2-1 mutant roots (by approximately 54%) compared with WT, but no significant alterations in the cell-wall-bound FA content in other examined contexts (Fig. 4B).
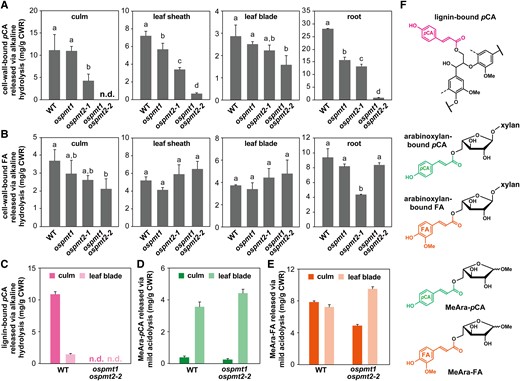
Cell-wall-bound p-coumarate (pCA) and ferulate (FA) contents of OsPMT1- and OsPMT2-deficient rice mutants. A and B) Total cell-wall-bound pCA (A) and FA (B) contents in rice culm, leaf sheath, leaf blade, and root as determined by mild alkaline hydrolysis of cell wall residue (CWR) samples. C) Lignin-bound pCA content in rice culm and leaf blade as determined by alkaline hydrolysis of CWR samples after removal of hemicelluloses via trifluoroacetic acid treatment. D and E) Methyl 5-O-p-coumaroyl-ʟ-arabinoside (MeAra-pCA) and methyl 5-O-feruroyl-ʟ-arabinoside (MeAra-FA) released from rice culm and leaf blade via mild acidolysis of CWR samples. F) Structures of lignin-bound pCA units, arabinoxylan-bound pCA and FA units, MeAra-pCA and MeAra-FA. The values in (A–C) are means ± standard deviation from individually analyzed plants (n = 3). Different letters indicate significant differences (ANOVA with post hoc Tukey–Kramer test, p < 0.05). The mild acidolysis to quantify MeAra-pCA and MeAra-FA was performed on pooled culm and leaf blade CWR samples prepared from three plants for each line. The values in (D and E) are means ± standard deviation from three independent analytical runs (n = 3). n.d., not detected; WT, wild-type control line; ospmt1, OsPMT1 single-knockout line; ospmt2-1, OsPMT2 single-knockout line; ospmt1 ospmt2-2, OsPMT1 and OsPMT2 double-knockout line.
Given that cell-wall-bound pCA can be linked to either lignin or hemicelluloses, especially arabinoxylan (Ralph 2010; Bartley et al. 2013), we suspected that the relatively high amount (approximately 45% of the wild-type level) of the residual pCA detected in the leaf blades of ospmt1 ospmt2-2 was not from lignin-bound pCA but from arabinoxylan-bound pCA (Fig. 4F). To test this hypothesis, we estimated the lignin- and arabinoxylan-bound pCA levels in the culm and leaf blade cell walls of ospmt1 ospmt2-2 and WT. Lignin-bound pCA levels were estimated by quantifying pCA released via the mild alkaline hydrolysis of CWRs after removing hemicelluloses by treatment with dilute trifluoroacetic acid (Saulnier et al. 1995; Bartley et al. 2013). In a more direct approach, we also estimated arabinoxylan-bound pCA and FA by quantifying p-coumaroylated and feruloylated arabinose (methyl 5-O-p-coumaroyl-ʟ-arabinoside, MeAra-pCA; and methyl 5-O-feruloyl-ʟ-arabinoside, MeAra-FA, respectively) (Fig. 4F) released via the mild acidolysis of CWRs (Lapierre et al. 2018). In ospmt1 ospmt2-2, lignin-bound pCA content was reduced to undetectable levels in both culms and leaf blades (Fig. 4C). In contrast, the levels of arabinoxylan-bound pCA (MeAra-pCA) remained comparable to those observed in WT (Fig. 4D). These results are in accordance with our hypothesis that the remaining pCA detected in the leaf blades of ospmt1 ospmt2-2 primarily originated from arabinoxylan-bound pCA. Arabinoxylan-bound FA (MeAra-FA) amounts were comparable between the culms of WT and ospmt1 ospmt2-2 but exhibited an increase in the leaf blades of ospmt1 ospmt2-2 compared to WT (Fig. 4E).
Overall, these results strongly support the notion that OsPMT1 and OsPMT2 function as the major PMTs contributing to lignin p-coumaroylation in mature rice vegetative organs. The results also indicated that cell-wall-bound pCA is predominantly linked to lignin in culms, whereas it is linked to both lignin and arabinoxylan in leaf blades in rice (Fig. 4).
Lignin content and p-coumaroylation status in culm cell walls of OsPMT1- and OsPMT2-deficient rice mutants
To further investigate the altered lignin biosynthesis in the ospmt1, ospmt2-1, and ospmt1 ospmt2-2 mutants, we analyzed the lignin content and composition of the rice CWR samples with chemical methods.
First, the lignin content of CWRs from culm, leaf sheath, leaf blade, and root were quantified with the thioglycolic acid lignin assay. Relative to WT, ospmt2-1 and ospmt1 ospmt2-2 displayed significantly less lignin in the culm and root (approximately 41% and 23% to 27% reductions, respectively) but no change in the leaf sheath or leaf blade; whereas, ospmt1 and WT had similar lignin content in all organs examined (Fig. 5A).
![Lignin content and compositional analysis of OsPMT1- and OsPMT2-deficient rice mutant cell walls. A) Lignin content in rice culm, leaf sheath, leaf blade, and root as determined by a thioglycolic acid lignin assay. B–H) Lignin compositional analysis of culm tissues by derivatization followed by reductive cleavage (DFRC). DFRC-derived non-γ-acylated (HOH, GOH, and SOH) and γ-p-coumaroylated (GpCA and SpCA) monomeric products released via cleavage of the β–O–4 linkages (B). Yield (C) and composition (D) of DFRC-derived monomeric products. Total DFRC-derived product yield (HOH + GOH + SOH + GpCA + SpCA) (E). S and G product ratios for total [(SOH + SpCA)/(GOH + GpCA)] (F), non-γ-acylated (SOH/GOH) (G), and γ-p-coumaroylated (SpCA/GpCA) (H) monomeric products. The values are means ± standard deviation from individually analyzed plants (n = 3). Different letters indicate significant differences (ANOVA with post hoc Tukey–Kramer test, p < 0.05). n.d., not detected; WT, wild-type control line; ospmt1, OsPMT1 single-knockout line; ospmt2-1, OsPMT2 single-knockout line. ospmt1 ospmt2-2, OsPMT1 and OsPMT2 double-knockout line.](https://oup.silverchair-cdn.com/oup/backfile/Content_public/Journal/plphys/194/2/10.1093_plphys_kiad549/1/m_kiad549f5.jpeg?Expires=1747898539&Signature=3PxN8Khjq3exmWfjQjCujqECcLBi9yvfaUTTXyK~uXYONvw90yw7Bn2k1MU3AQLmpRshFeVOTwp8NDhDaQ9zlz6KoMyIxQspi9qxBCz-5BDPFQSaEA~tnDpgKlou~NHNsMvJhKrGyWlLxmGRNdw3VrBniZq4vIgfo7nXWfkBydylEAaX~PXSdOi80YkG8yTQYbzH00pRU9QLO-QYIB~LhL6xbhplEHGo071g24p5tp-WwzZOTmEf87S-8ijuvETim8i~e0Xr3AKihHBlxqe6HKPSjEUm0uC77hGCoIoWccihVYwv3ZiAO74pC~2UillYxw8qR7expyvA1FGHaPir1Q__&Key-Pair-Id=APKAIE5G5CRDK6RD3PGA)
Lignin content and compositional analysis of OsPMT1- and OsPMT2-deficient rice mutant cell walls. A) Lignin content in rice culm, leaf sheath, leaf blade, and root as determined by a thioglycolic acid lignin assay. B–H) Lignin compositional analysis of culm tissues by derivatization followed by reductive cleavage (DFRC). DFRC-derived non-γ-acylated (HOH, GOH, and SOH) and γ-p-coumaroylated (GpCA and SpCA) monomeric products released via cleavage of the β–O–4 linkages (B). Yield (C) and composition (D) of DFRC-derived monomeric products. Total DFRC-derived product yield (HOH + GOH + SOH + GpCA + SpCA) (E). S and G product ratios for total [(SOH + SpCA)/(GOH + GpCA)] (F), non-γ-acylated (SOH/GOH) (G), and γ-p-coumaroylated (SpCA/GpCA) (H) monomeric products. The values are means ± standard deviation from individually analyzed plants (n = 3). Different letters indicate significant differences (ANOVA with post hoc Tukey–Kramer test, p < 0.05). n.d., not detected; WT, wild-type control line; ospmt1, OsPMT1 single-knockout line; ospmt2-1, OsPMT2 single-knockout line. ospmt1 ospmt2-2, OsPMT1 and OsPMT2 double-knockout line.
Next, given the prominent reductions in cell-wall-bound pCA in mutant culm cell walls (Fig. 4), we further analyzed lignin p-coumaroylation status by derivatization followed by reductive cleavage (DFRC), a method orthogonal to the above mild acidolysis method for surveying the acylation of arabinoxylans. DFRC reactions cleave the major β–O–4-ether linkages in lignin polymers while leaving the γ-ester linkages intact, thereby releasing γ-p-coumaroylated monomeric products (GpCA and SpCA) together with non-γ-acylated monomeric products (HOH, GOH, and SOH), and providing useful information regarding the lignin p-coumaroylation status in grass cell walls (Lu and Ralph 1997, 1999; Karlen et al. 2018) (Fig. 5B).
Our DFRC analyses indicated that the amounts of the γ-p-coumaroylated monomeric products (GpCA and SpCA) were significantly reduced in the ospmt1, ospmt2-1, and ospmt1 ospmt2-2 cell walls compared with those released from the WT control cell walls (Fig. 5, C and D). In line with the data for cell-wall-bound pCA (Fig. 4), GpCA and SpCA were undetectable in the double ospmt1 ospmt2-2 knockout mutants; whereas, substantial, but decreased, amounts were detected in the DFRC products from the single ospmt1 or ospmt2-1 lines (Fig. 5, C and D). In contrast, levels of the non-γ-p-coumaroylated monomeric products (HOH, GOH, and SOH) released from the cell walls of the mutants were comparable to those released from the WT control cell walls. Along with depletion of lignin-bound pCA units, the OsPMT1- and OsPMT2-deficient rice mutants displayed considerably altered lignin aromatic unit compositions. The total S/G product ratio [(SOH + SpCA)/(GOH + GpCA)] was slightly reduced in ospmt1 and greatly reduced in ospmt1 ospmt2-2, compared with WT (Fig. 5F). Analysis of different S/G product ratios suggests that the shift in total S/G in ospmt1 ospmt2-2 is mainly due to depletion in the γ-p-coumaroylated lignin monomers (GpCA and SpCA), particularly SpCA which we detect at much greater abundance than GpCA in the WT cell walls: the SpCA/GpCA ratio was approximately 6.7 (Fig. 5H), whereas the SOH/GOH ratio was approximately 0.3 in the WT cell walls (Fig. 5G). Indeed, despite the reduction in the total S/G ratio (Fig. 5F), the nonacylated SOH/GOH ratio may increase slightly relative to that of WT in ospmt1 ospmt2-2 (Fig. 5G).
Two-dimensional NMR analysis of culm cell walls of OsPMT1- and OsPMT2-deficient rice mutants
To further investigate the impact of the OsPMT1 and OsPMT2 mutations on lignin p-coumaroylation, we conducted solution-state 2D 1H–13C heteronuclear single-quantum coherence (HSQC) NMR analysis of the culm cell walls from the ospmt1, ospmt2-1, and ospmt1 ospmt2-2 mutants. We used lignin-enriched cell wall (enzyme lignin) fractions prepared from the rice culm CWR samples by the enzymatic removal of the cell wall polysaccharides (Kim and Ralph 2010; Tobimatsu et al. 2019) to avoid interference from arabinoxylan-bound pCA.
The aromatic subregion in the NMR spectrum of the WT enzyme lignin fraction displayed typical signals from S, G, and H lignin units (S, G, and H) along with those from lignin-bound tricin (T) and pCA (pCA), and lignin- or residual-polysaccharide-bound FA (FA) (Fig. 6). To investigate the lignin compositional changes in the ospmt1, ospmt2-1, and ospmt1 ospmt2-2 cell walls, we performed volume integration of the well-resolved major aromatic signals (½S2/6, G2, ½T2′/6′, and ½pCA2/6, normalized using ½S2/6 + G2). Although pCA units, being mobile end-groups on the lignin, have longer relaxation times and are therefore considerably over-quantified relative to the backbone lignin units in these spectra, comparative level data are valid (Mansfield et al. 2012). The normalized pCA signal intensity was notably reduced from 91% in WT to 81% and 34% in the ospmt1 and ospmt2-1 mutants, respectively, consistent with the lignin-bound-pCA being considerably depleted in the single-knockout mutants. Furthermore, the resolved pCA signals (pCA2/6, pCA7, and pCA8) were depleted to undetectable levels in the spectrum of the ospmt1 ospmt2-2 mutant (Fig. 6), indicating that, in line with the above chemical analysis data, culms of this mutant were practically devoid of lignin-bound pCA.
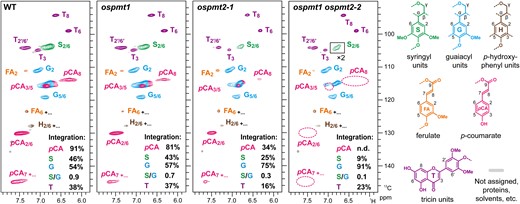
Two-dimensional NMR analysis of culm enzyme lignin fractions prepared from wild-type and OsPMT1- and OsPMT2-deficient rice culms. Aromatic subregions of 1H–13C short-range correlation (HSQC) NMR spectra are shown. Box labeled ×2 indicates region with scale vertically enlarged 2-fold. Volume integration data of the major aromatic units (½S2/6, G2, ½T2′/6′, and ½pCA2/6) are expressed as percentages on a ½S2/6 + G2 = 100 basis. Contour coloration matches that of lignin substructures shown. n.d., not detected; WT, wild-type control line; ospmt1, OsPMT1 single-knockout line; ospmt2-1, OsPMT2 single-knockout line. ospmt1 ospmt2-2, OsPMT1 and OsPMT2 double-knockout line.
Apart from the depletion in pCA, the NMR analysis also detected alterations in the aromatic unit composition of the mutant lignin. In qualitative agreement with the DFRC data, the S/G signal ratios (½S2/6/G2) were notably reduced from that of WT (S/G signal ratio = 0.9) in ospmt1 (S/G signal ratio = 0.7) and even more reduced in ospmt2-1 (S/G signal ratio = 0.3) and ospmt1 ospmt2-2 (S/G signal ratio = 0.1). The normalized intensity of the tricin signals (½T2′/6′) was also lowered compared with the WT (38%) to ospmt2-1 (16%) and ospmt1 ospmt2-2 (23%); whereas ospmt1 showed little reduction in the tricin signals (37%).
These NMR data further corroborated our contention that both OsPMT1 and OsPMT2 are functional and partially redundant PMTs involved in lignin p-coumaroylation in rice culm cell walls. Collectively with the DFRC data (Fig. 5), these NMR data also indicate that the PMT mutations affected not only lignin p-coumaroylation but also lignin monomer composition in rice culms.
Comparison of isolated lignins from WT and ospmt1 ospmt2-2
To further elucidate the lignin structural changes upon elimination of the lignin p-coumaroylation, dioxane-water-soluble lignins were further purified from the culm enzyme lignin fractions and then subjected to additional lignin structural analysis via 2D HSQC NMR and gel-permeation chromatography (GPC).
In agreement with the results presented above, intense pCA signals were detected in the WT control lignin spectrum but were below the detection limit in the lignin spectrum of the ospmt1 ospmt2-2 mutant (Fig. 7A). Furthermore, the volume integration data indicated a large reduction in the S/G unit ratio, as well as a notable reduction in the tricin units in ospmt1 ospmt2-2. The normalized ½T2′/6′ signal intensity was depleted by 25% in the ospmt1 ospmt2-2 mutant lignin spectrum compared with that in WT.
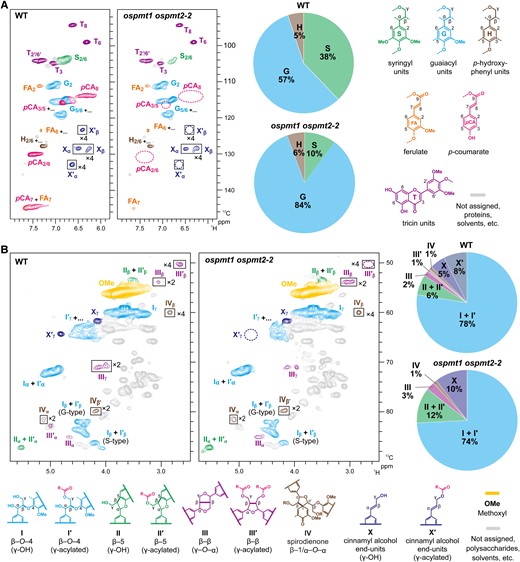
Two-dimensional NMR analysis of dioxane-water-soluble lignin isolated from wild-type and OsPMT1- and OsPMT2-deficient rice culms. Aromatic (A) and oxygenated aliphatic subregions of 1H–13C short-range correlation (HSQC) NMR spectra are shown. Contour coloration matches that of lignin substructures shown in each panel. Boxes labeled ×2 and ×4 indicate regions with scale vertically enlarged 2- and 4-fold. Pie charts illustrate volume integration data of the major aromatic units expressed as percentages on a ½S2/6 + G2 + ½H2/6 = 100 basis in (A) and the major lignin inter-monomeric linkages and end-units expressed as percentages on a Iα + I′α + IIα + II′α + ½IIIα + ½III′β + IVα = 100 basis in (B). NMR analysis was conducted for lignin samples prepared from culm cell wall residue (CWR) samples pooled from three biologically independent plants. n.d., not detected; WT, wild-type control line; ospmt1 ospmt2-2, OsPMT1 and OsPMT2 double-knockout line.
The oxygenated aliphatic subregions of the HSQC spectra indicated changes in lignin substructures, i.e. distribution of the lignin units characterized by their various inter-monomeric linkages (Fig. 7B). In the WT control lignin spectrum, typical signals from the major lignin inter-monomeric linkages, such as β–O–4 (I and Iʹ), β–5 (II and IIʹ), resinol- (III) and tetrahydrofuran-type β–β (IIIʹ), and spirodienone (IV) units, as well as those from non-γ-acylated (X) and γ-acylated cinnamyl alcohol end-units (Xʹ), could be clearly seen. Volume integration analysis of these major lignin substructure signals indicated changes in the ospmt1 ospmt2-2 lignins (see Supplemental Methods for details). β–O–4 units were slightly reduced in the ospmt1 ospmt2-2 mutant compared with those in WT (normalized Iα + I′α signal intensity was reduced to 74% from 78% in WT). The β–5 units were about doubled in the ospmt1 ospmt2-2 mutant (normalized IIα + II′α signal intensity was increased to 12%, from 6% in WT). The signals from the tetrahydrofuran-type β–β (IIIʹ) units originating from the incorporation of γ-acylated monolignols (Lu and Ralph 2002) were completely depleted to undetectable levels in the ospmt1 ospmt2-2 mutant lignin spectrum. The signals from resinol-type β–β (III) units from non-γ-acylated monolignols were proportionally increased and, consequently, the total proportion of the β–β unit signals in ospmt1 ospmt2-2 remained comparable to those in WT (normalized IIIα + III′α signal intensities, approximately 3% in both ospmt1 ospmt2-2 and WT spectra). Similarly, the γ-acylated cinnamyl alcohol end-unit signals (Xʹ) totally disappeared, and non-γ-acylated cinnamyl alcohol end-unit signals (X) increased in the ospmt1 ospmt2-2 spectrum (normalized ½Xγ signal intensity increased to 10%, from 5% in WT). The total volume of the end-unit signals was reduced in the ospmt1 ospmt2-2 spectrum (normalized ½Xγ + ½X′γ signal intensity reduced to 10%, from 13% in the WT control spectrum). The spirodienone signals (IV) remained similar in ospmt1 ospmt2-2 compared with WT (normalized IVɑ signals approximately 1% in both ospmt1 ospmt2-2 and WT).
Lastly, the molecular weight distributions of acetylated lignin samples of the ospmt1 ospmt2-2 mutant and WT were determined by GPC. Both WT and ospmt1 ospmt2-2 mutant lignins had number- and weight-averaged molecular weights (Mn and Mw, respectively) and polydispersity indexes (Mw/Mn) within the range of the literature values reported for plant lignins isolated and analyzed under similar conditions (Tobimatsu et al. 2013; Eloy et al. 2017) (Table 1). Nevertheless, the averaged molecular weights of the ospmt1 ospmt2-2 mutant lignins were higher (by approximately 22% and 24% for Mn and Mw, respectively) than those of the WT lignin samples, whereas the Mw/Mn values remained similar (Table 1).
Molecular weight distribution data of dioxane-water-soluble lignin isolated from wild-type and OsPMT1- and OsPMT2-deficient rice culms. Determined by gel-permeation chromatography after acetylation using polystyrene standards for molecular weight calibration
Genotype . | Mn . | Mw . | Mw/Mn . |
---|---|---|---|
WT | 2300 | 6600 | 2.9 |
ospmt1 ospmt2-2 | 2800 | 8200 | 2.9 |
Genotype . | Mn . | Mw . | Mw/Mn . |
---|---|---|---|
WT | 2300 | 6600 | 2.9 |
ospmt1 ospmt2-2 | 2800 | 8200 | 2.9 |
Mn, number average molecular weight; Mw, weight average molecular weight; WT, wild-type control line; ospmt1 ospmt2-2, OsPMT1 and OsPMT2 double-knockout line.
Molecular weight distribution data of dioxane-water-soluble lignin isolated from wild-type and OsPMT1- and OsPMT2-deficient rice culms. Determined by gel-permeation chromatography after acetylation using polystyrene standards for molecular weight calibration
Genotype . | Mn . | Mw . | Mw/Mn . |
---|---|---|---|
WT | 2300 | 6600 | 2.9 |
ospmt1 ospmt2-2 | 2800 | 8200 | 2.9 |
Genotype . | Mn . | Mw . | Mw/Mn . |
---|---|---|---|
WT | 2300 | 6600 | 2.9 |
ospmt1 ospmt2-2 | 2800 | 8200 | 2.9 |
Mn, number average molecular weight; Mw, weight average molecular weight; WT, wild-type control line; ospmt1 ospmt2-2, OsPMT1 and OsPMT2 double-knockout line.
Discussion
OsPMT1 and OsPMT2 function cooperatively for lignin p-coumaroylation in rice
In the present study, we have shown that OsPMT1 (OsAT4) and OsPMT2 (OsAT3) both function as PMTs for the generation of lignin-bound pCA units in rice cell walls. Recombinant OsPMT1 has previously been reported to catalyze the p-coumaroylation of three monolignol substrates (Withers et al. 2012; Smith et al. 2022b). Here, we further showed that recombinant OsPMT2 possessed similar PMT activity to OsPMT1 in vitro (Fig. 3). The in planta functions of OsPMT1 and OsPMT2 were demonstrated by cell wall analyses of genome-edited rice mutants deficient in either or both OsPMT1 and OsPMT2, providing in vivo genetic evidence to support these biochemical assignments. The chemical analyses revealed that the ospmt1 ospmt2-2 double-knockout mutant had a dramatically reduced content of cell-wall-bound pCA in all the major vegetative tissues examined (Fig. 4). Further chemical and 2D NMR analyses suggested that lignin-bound pCA was practically eliminated in the culm and leaf blade tissues of the ospmt1 ospmt2-2 double-knockout mutant and significantly reduced in both the ospmt1 and ospmt2-1 single-knockout mutants (Figs. 4 to 7). As the DFRC analysis indicated that p-coumaroylated S and G lignin units were both reduced in the single-knockout mutants (Fig. 5), both OsPMT1 and OsPMT2 contribute to the biosynthesis of both S- and G-type monolignol-pCA conjugates, i.e. S-pCA and G-pCA, respectively, in planta.
Given the elimination of lignin-bound pCA in the culm and leaf blade of the ospmt1 ospmt2-2 double-knockout mutant (Fig. 4), BAHD ATs other than OsPMT1 and OsPMT2 are unlikely to appreciably contribute to lignin p-coumaroylation in these rice organs. Heterologous expression of B. distachyon BdPMT2 was reported to generate p-coumaroylated lignin in transgenic Arabidopsis (Sibout et al. 2016). However, BdPMT2 and its putative ortholog in rice, OsAT8 (Fig. 2), do not have important roles in p-coumaroylation of lignin in adult culms, as lignin-bound pCA was undetectable in culms of the B. distachyon bdpmt1 mutant (Petrik et al. 2014) and the rice ospmt1 ospmt2-2 mutant (Figs. 4 to 7). One explanation is that OsAT8 may function in specific cell types or stages that are poorly represented in mature culms. Although we did detect expression of OsAT8 in lignifying vegetative organs (Supplemental Figs. S1 and S5), an analysis of diverse aerial tissues at different developmental stages found the highest OsAT8 transcript abundance in leaves of 3-day-old seedlings, followed by young nodes and leaves (Lin et al. 2016). Alternatively, BdPMT2 and OsAT8 may not function as bona fide PMTs that produce p-coumaroylated monolignols in grasses and this activity might have been detected in the heterologous system due to, for example, absence of the native substrate.
Recent data suggest that OsPMT1 can also accept feruloyl-CoA and acetyl-CoA as acyl donors in vitro, albeit with lower activities than pCA-CoA (Smith et al. 2022b). Although we detected some fluctuations in the cell-wall-bound FA contents in the PMT-deficient rice mutants, no consistent changes were observed (Fig. 4), suggesting that these changes may not be directly related to the loss of function(s) of OsPMT1 and/or OsPMT2. Other members of the BAHD AT family—OsFMT/OsAT5 and its putative orthologs (Fig. 2)—have been strongly implicated in lignin feruloylation (Karlen et al. 2016; Smith et al. 2022b). Furthermore, BAHD ATs associated with OsAT10 and OsAT9 have been implicated in arabinoxylan p-coumaroylation and feruloylation (Bartley et al. 2013; Li et al. 2018; de Souza et al. 2018, 2019; Mota et al. 2021; Tian et al. 2021; Möller et al. 2022) (Fig. 2). Overall, we conceive that OsPMT1 and OsPMT2 are necessary and sufficient for the p-coumaroylation of lignin in cell walls of mature, vegetative organs of rice.
Disruption of lignin p-coumaroylation alters lignin polymer structures
Disruption of the rice PMTs not only eliminated the lignin pCA decorations but also altered core lignin polymer structures. In the DFRC and 2D NMR analysis, the disruption in ospmt1 ospmt2-2 was associated with a large reduction in the S/G aromatic unit ratio, with a similar but lesser effect in ospmt1 and ospmt2-1 (Figs. 5 to 7). Reductions in S/G lignin unit ratios were also observed in maize ZmpCAT-downregulated RNAi lines (Marita et al. 2014) but were inconsistent in B. distachyon upon BdPMT1 disruption (Petrik et al. 2014). Relative to lignin of the wild type, 2D NMR showed a greater S/G ratio in the bdpmt1 knockout mutant whereas, an RNAi line showed a reduction and an over-expression line an increase (Petrik et al. 2014). The wild-type Brachypodium culm S/G ratio is high (∼1.2) compared to that of rice and maize which might influence the phenomenon, or the ability to detect a shift. A further piece of evidence is the absence of obvious changes in the S/G lignin unit ratios in transgenic poplar and Arabidopsis plants heterologously expressing grass PMTs (Smith et al. 2015; Sibout et al. 2016; Lapierre et al. 2021). In the rice PMT-deficient mutants, the notable changes in the S/G lignin unit ratios may be related to the abundance of S-type monolignol-pCA conjugates (S-pCA), which may be up to 20% of the DFRC-sensitive β–O–4 units in the cell walls of wild-type rice, whereas the other conjugate classes, such as G-pCA, are >5-fold less abundant (Fig. 5). Considering the role of PMT in synthesizing S-pCA from S-OH, it was somewhat surprising that the specific reduction in conjugated S units from S-pCA does not lead to a proportional increase in unconjugated S units from S-OH in the mutant lignin. This disparity might point to another mechanism for the S/G ratio reduction, such as a role for monolignol-appended pCA in aiding the incorporation of S-OH during lignification, as previously proposed (Hatfield et al. 2008; Ralph 2010).
The NMR and GPC analysis of the isolated lignins provide further evidence for the substantial changes in the lignin substructures in ospmt1 ospmt2-2 that are mostly attributable to S/G ratio shifts (Fig. 7). Beyond the direct effect of the elimination of monolignol-pCA conjugates in lignin polymerization (e.g. loss of IIIʹ and Xʹ units derived from monolignol-pCA conjugates), the ospmt1 ospmt2-2 lignin displayed reduced β–O–4 (I and Iʹ) and increased β–5 (II and IIʹ) levels. Such changes in the abundances of these lignin units are likely a consequence of the reduction in the S/G monomer ratio, i.e. decreased incorporation of the total of S-OH and S-pCA relative to the total of G-OH and G-pCA in lignin polymerization. This phenomenon has been observed in other lignin transgenic and mutant plants in which the S/G monomer ratio was altered (Stewart et al. 2009; Anderson et al. 2015; Takeda et al. 2017). The GPC analysis further revealed that the averaged molecular weights of the isolated ospmt1 ospmt2-2 mutant lignins were higher than those of the wild-type control lignins (Table 1). This result was corroborated by the reduced abundance of the polymer end-units (X and X′) in the mutant lignins as determined by 2D NMR (Fig. 7). The increased molecular weight of the isolated lignin in the ospmt1 ospmt2-2 mutant may also be, at least partially, associated with the reduced S/G lignin monomer ratio. The molecular weights of synthetic lignins are negatively associated with S/G monomer ratios during in vitro lignin polymerization (Tobimatsu et al. 2008). In agreement with this observation, CAld5H/F5H-over-expressing poplar transgenic lines with increased S/G lignin monomer ratios displayed reduced molecular weights of the isolated lignin (Stewart et al. 2009).
Our NMR data also suggested that tricin-lignin levels were reduced in the ospmt2-1 and ospmt1 ospmt2-2 mutant lignins (Fig. 6). In a simple model, the disruption in monolignol p-coumaroylation due to the PMT-deficiency could potentially elevate the abundance of the PMT substrate, pCA-CoA. Because tricin biosynthesis also relies on pCA-CoA as a key entry precursor into the flavonoid biosynthetic pathway (Fig. 1), such an increase of pCA-CoA could redirect metabolic flux toward tricin biosynthesis and result in augmentation of tricin units within the final lignin polymer. However, this scenario disagrees with our current observations in the PMT-deficient rice mutants, necessitating further investigation.
Implications for the biosynthesis of p-coumaroylated lignin in grasses
Several recent studies on grass lignin biosynthesis have found evidence for partitioning in grasses of the cinnamate/monolignol pathway enzymes between generation of monolignol-pCA conjugates and nonacylated monolignols (Umezawa et al. 2020; Chandrakanth et al. 2023). Barros et al. (2016) reported that the function in B. distachyon of the grass-specific bifunctional ʟ-phenylalanine/ʟ-tyrosine ammonia-lyase (PTAL) (Fig. 1) may be linked to the biosynthesis of S lignin and cell-wall-bound pCA, both of which can be derived from S-pCA, the major monolignol-pCA conjugate in lignification. Furthermore, OsC3ʹH1 (Takeda et al. 2018) and OsCAld5H1 (Takeda et al. 2019), two major cytochrome P450 enzymes that catalyze aromatic hydroxylation in rice monolignol biosynthesis (Fig. 1), preferentially catalyze the biosynthesis of nonacylated monolignols over monolignol-pCA conjugates in rice, although a recent study reported a contradictory result in CAld5H-downregulated barley (Shafiei et al. 2023). Moreover, two different isoforms of rice 4-coumaroyl-CoA ligase (Os4CL3 and Os4CL4) (Fig. 1) contribute differently to the biosynthesis of nonacylated monolignols and monolignol-pCA conjugates (Afifi et al. 2022).
As has been commonly depicted and assumed, PMTs may act on the abundant monolignols, i.e. G-OH and S-OH, to generate the corresponding monolignol-pCA conjugates, i.e. G-pCA and S-pCA, that diffuse across the cell membrane for lignification (reactions indicated by the solid arrows in Fig. 1). In a possible alternative pathway, H-OH may act as a major substrate for PMT to produce H-pCA that may be further converted into G-pCA and S-pCA via aromatic hydroxylation and O-methylation of the monolignol moieties by as yet uncharacterized enzymes (reactions indicated by dotted arrows in Fig. 1) (Takeda et al. 2018). The possible role of H-pCA as a precursor in the lignin monomer biosynthesis was originally proposed based on the considerably high catalytic activity of OsPMT1 toward H-OH in vitro (Withers et al. 2012). Sorghum SbPMT and switchgrass PvPMT (Fig. 2) were also reported to possess catalytic activity toward H-OH in vitro (Smith et al. 2022b). If the pathway from H-pCA was the major pathway for the generation of G-pCA and S-pCA, the loss of function of PMT might be expected to result in the abnormal accumulation of H-OH and an increase the abundance of nonacylated H units in the mutant lignin. However, neither we nor others (Marita et al. 2014; Petrik et al. 2014) have detected an increase in H lignin units in PMT-deficient mutants (Fig. 5).
Overall, the accumulated data indicate the complexity of the metabolic networks for the biosynthesis of monolignol-pCA conjugates used in grass lignification. More extensive examination of the catalytic activities and gene expression of the monolignol biosynthetic enzymes, the pathway metabolic flux, and the cell wall structures of lignin mutant and transgenic plants is required to understand the biosynthesis of p-coumaroylated lignin in grasses. The mild or absent vegetative growth phenotypes, at least under unstressed conditions, of rice and B. distachyon (Petrik et al. 2014) deficient in p-coumaroylated lignin highlight our absence of understanding of the selective advantage for evolution of pCA and other cell wall decoration in commelinid monocots. Along with the B. distachyon bdpmt1 mutant (Petrik et al. 2014), the rice ospmt1 ospmt2-2 mutant should be a useful subject to investigate the functions and properties of lignin-bound pCA units in grass cell walls, and their influence on grass biomass utilization.
Materials and methods
Phylogenetic reconstruction and gene atlas analysis
Protein sequences were aligned by Clustal W (Larkin et al. 2007) and corrected manually. The unrooted phylogenetic tree was constructed with the maximum likelihood method using MEGAX (Kumar et al. 2018). The DNA microarray-based gene expression data were obtained from the Rice Expression Profile Database (Sato et al. 2012).
Gene expression analysis by RT-qPCR
Total RNA was extracted from culm, leaf sheath, and leaf blade at the heading stage and root at 2-month-old seedling stage of wild-type rice (Oryza sativa), and reverse transcribed as described previously (Koshiba et al. 2013a). RT-qPCR was performed using a StepOne Real-Time PCR System (Applied Biosystems, Foster City, CA, USA) with a ubiquitin gene (OsUBQ5) as an internal control and gene-specific primers listed in Supplemental Table S3.
Recombinant protein expression and enzymatic assay
The expression of recombinant OsPMT1 and OsPMT2 in E. coli cells is described in the Supplemental Methods. Enzymatic assays were performed in accordance with the methods described by Withers et al. (2012) with minor modifications as described in Supplemental Methods. Monolignol substrates (Quideau and Ralph 1992) and the monolignol-pCA conjugate product standards (Suzuki et al. 2002; Zhu et al. 2013) were synthesized, as previously described.
Generation of CRISPR/Cas9 rice mutants
The ospmt1, ospmt2-1, and ospmt1 ospmt2-2 mutant lines were generated via CRISPR/Cas9-mediated mutagenesis using the pZH_OsU6gRNA_MMCas9 vector (Mikami et al. 2015) as described in Supplemental Methods. Regenerated plants and isolated T1 and T2 mutant lines used for the phenotypic characterization and cell wall analyses were grown to maturity in soil pods under growth chamber conditions as described previously (Lam et al. 2017). The 2-month-old seedlings (T2 homozygous mutant lines) used for the root cell wall analysis were grown in a hydroponic system as described by Koshiba et al. (2013a).
Cell wall structural analyses
Extractive-free CWRs for cell wall analyses were prepared from mature (approximately 40 days after heading) T1 (culm, leaf sheath and leaf blade) or 2-month-old T2 (root) mutant plants along with wild-type control plants as described previously (Yamamura et al. 2012). The thioglycolic acid assay (Suzuki et al. 2009), quantitation of cell-wall-bound pCA and FA released by alkaline hydrolysis (Yamamura et al. 2011), and DFRC analysis (Karlen et al. 2016; Takeda et al. 2018), were performed as described previously. For the analysis of lignin-bound pCA, CWR samples were incubated with 0.05 M trifluoroacetic acid at 100°C for 5 h, and the remaining pellets (hemicellulose-free CWR) were washed with distilled water (Bartley et al. 2013) and then subjected to the alkaline hydrolysis for quantitation of the pCA content (Yamamura et al. 2011). The mild acidolysis (Lapierre et al. 2018), 2D HSQC NMR, and GPC were performed as described in Supplemental Methods. Peak assignments for solution-state HSQC spectra were based on a comparison with literature data (Kim and Ralph 2010; Mansfield et al., 2012; Lan et al. 2015; Tobimatsu et al. 2019; Martin et al. 2023).
Statistical analysis
A one-way ANOVA followed by Tukey–Kramer multiple comparison test were performed using GraphPad Prism 8 (GraphPad Software, San Diego, CA, USA).
Accession numbers
The accession numbers for the protein and gene sequences used in this study are listed in Supplemental Table S4.
Acknowledgments
We thank Dr. Seiichi Toki, Dr. Masaki Endo, and Mr. Masafumi Mikami (National Agricultural and Food Organization) for providing the pZH_OsU6gRNA_MMCas9 vector, Dr. Steven D. Karlen (University of Wisconsin) for his assistance and helpful suggestions in chemical analysis, and Dr. Hironori Kaji, Ms. Ayaka Maeno, and Ms. Kyoko Yamada (ICR, Kyoto University) for their assistance in NMR analysis. A part of this study was conducted using the facilities at the DASH/FBAS, RISH, Kyoto University, and the NMR spectrometer at JURC, ICR, Kyoto University.
Author contributions
L.P.Y.L., Y.T., L.E.B. and T.U. conceived research and wrote the manuscript with help from all the others. L.P.Y.L., Y.T., S.S., T.T., S.Y., Y.T.-K. Y.O., K.O., J.R. and L.E.B. designed and performed experiments, and analyzed the data.
Supplementary data
The following supplemental materials are available.
Supplemental Methods. Additional experimental procedures.
Supplemental Figure S1. Gene expression analysis of OsPMT1 and OsPMT2.
Supplemental Figure S2. Genotype and morphology of rice mutants.
Supplemental Figure S3. Predicted effects of CRISPR/Cas9-induced mutations on OsPMT1.
Supplemental Figure S4. Predicted effects of CRISPR/Cas9-induced mutations on OsPMT2.
Supplemental Figure S5. Gene expression analysis of OsAT8.
Supplemental Table S1. Off-target analysis of rice mutants.
Supplemental Table S2. Growth performance of rice mutants.
Supplemental Table S3. Primers and oligonucleotides used in this study.
Supplemental Table S4. Accession numbers of the protein and gene sequences.
Funding
This work was supported in part by grants from the Japan Society for the Promotion of Science (JSPS) (grant nos. KAKENHI #16K14958 and #16H06198), RISH Kyoto University (grant nos. Mission-linked Research Funding #2016-5-2-1 and Humanosphere Science Research 2019 and 2021, and USDA-NIFA hatch project #1015621. L.P.Y.L., S.Y., and Y.T.-K. acknowledge the JSPS fellowship programs (program nos. #17F17103, #22J13457 and #17J0965416, respectively). J.R. was funded by the DOE Great Lakes Bioenergy Research Center (DOE Office of Science BER DE- SC0018409).
Data availability
The authors confirm that the data supporting the findings of this study are available within the article and its supplementary materials.
References
Author notes
The author responsible for distribution of materials integral to the findings presented in this article in accordance with the policy described in the Instructions for Authors (https://dbpia.nl.go.kr/plphys/pages/General-Instructions) is Yuki Tobimatsu.
Conflict of interest statement. None declared.