-
PDF
- Split View
-
Views
-
Cite
Cite
Takaki Yamauchi, Akihiro Tanaka, Mikio Nakazono, Yoshiaki Inukai, Age-dependent analysis dissects the stepwise control of auxin-mediated lateral root development in rice, Plant Physiology, Volume 194, Issue 2, February 2024, Pages 819–831, https://doi.org/10.1093/plphys/kiad548
- Share Icon Share
Abstract
As root elongation rates are different among each individual root, the distance from the root apices does not always reflect the age of root cells. Thus, methods for correcting variations in elongation rates are needed to accurately evaluate the root developmental process. Here, we show that modeling-based age-dependent analysis is effective for dissecting stepwise lateral root (LR) development in rice (Oryza sativa). First, we measured the increases in LR and LR primordium (LRP) numbers, diameters, and lengths in wild type and an auxin-signaling-defective mutant, which has a faster main (crown) root elongation rate caused by the mutation in the gene encoding AUXIN/INDOLE-3-ACETIC ACID protein 13 (IAA13). The longitudinal patterns of these parameters were fitted by the appropriate models and the age-dependent patterns were identified using the root elongation rates. As a result, we found that LR and LRP numbers and lengths were reduced in iaa13. We also found that the duration of the increases in LR and LRP diameters were prolonged in iaa13. Subsequent age-dependent comparisons with gene expression patterns suggest that AUXIN RESPONSE FACTOR11 (ARF11), the homolog of MONOPTEROS (MP)/ARF5 in Arabidopsis (Arabidopsis thaliana), is involved in the initiation and growth of LR(P). Indeed, the arf11 mutant showed a reduction of LR and LRP numbers and lengths. Our results also suggest that PINOID-dependent rootward-to-shootward shift of auxin flux contributes to the increase in LR and LRP diameters. Together, we propose that modeling-based age-dependent analysis is useful for root developmental studies by enabling accurate evaluation of root traits' expression.
Introduction
Main roots develop from the stem cells located in the apical parts (Dolan and Okada 1999; Hochholdinger et al. 2004). The longitudinal organization of adventitious (crown) root cells in Poaceae family, such as rice (Oryza sativa) and maize (Zea mays ssp. mays), is similar to that in the primary roots of Arabidopsis (Arabidopsis thaliana), except for the complexity of structural organization: from the apical part to basal part, they comprise meristematic zone, elongation zone, and differentiation zone (DZ; Coudert et al. 2010; Petricka et al. 2012; Yu et al. 2016). Most root cells are generated from apical meristems (Jiang and Feldman 2005; Motte et al. 2019); thus, cell proliferation and elongation rates are coordinately associated with root elongation rates. As cells at the same position in the different roots correspond to the different time points given that the elongation rates are different among the roots (Yamauchi et al. 2021), effective methods are needed to correct variations in root elongation rates for accurately evaluating developmental processes.
Auxin signaling is mediated by AUXIN RESPONSE FACTORs (ARFs), which bind to auxin response elements on the promoters of auxin-responsive genes (Ulmasov et al. 1997). ARF-dependent transcriptional activation is repressed by AUXIN/INDOLE-3-ACETIC ACID proteins (AUX/IAAs; IAAs) having four conserved amino acid sequence motifs (Rouse et al. 1998). Among them, AUX/IAA domain II is required for the auxin-dependent proteolysis of IAA proteins (Gray et al. 2001). In the rice iaa13 mutant, a single amino acid substitution causes the prevention of the degradation of the IAA13 protein, and this results in decreases in lateral root (LR) density and LR primordium (LRP) density (Kitomi et al. 2012). Further study shows that the amount of aerenchyma, which is a tissue filled with gas (Yamauchi et al. 2018), is reduced in the crown root of iaa13 (Yamauchi et al. 2019). Moreover, IAA13 physically interacts with ARF19 and controls the auxin signaling during LR and aerenchyma formations in rice crown roots (Yamauchi et al. 2019). Interestingly, ARF19 and IAA13 are the homologs of the major contributors of LR initiation in Arabidopsis, i.e. ARF7/19 and SOLITARY ROOT (SLR)/IAA14, respectively (Fukaki et al. 2002; Okushima et al. 2005).
Previously, we demonstrated that the longitudinal patterns of aerenchyma formation in the crown roots of iaa13 and its background wild type (WT) fit well with the Gompertz model (Yamauchi et al. 2020), a sigmoid model that is commonly used to predict population growth (Gompertz 1825; Tjørve and Tjørve 2017). Subsequent age-dependent analysis using the model equations revealed that aerenchyma formation in iaa13 is delayed because of the predominant expression of the mutated IAA13 in the apical part of the crown roots (Inahashi et al. 2018; Yamauchi et al. 2019, 2020). Further studies have shown that the modeling-based analysis is also effective in evaluating the age-dependent patterns of aerenchyma formation in rice and maize under low-oxygen conditions (Yamauchi and Nakazono 2022). Recently, we have also demonstrated that the Gompertz model is useful to reveal the accurate patterns of auxin-dependent aerenchyma formation in the nodal roots of a wild relative of maize (Ning et al. 2023).
Lateral root primordia originate from the pericycle cells in the DZ in the main (primary) roots of Arabidopsis (Petricka et al. 2012). On the other hand, LRP originate from the pericycle and endodermal cells in rice and some other plant species (Yu et al. 2016; Torres-Martínez et al. 2019, 2022; Tanaka et al. 2023), even though the permanent body of LR is entirely of pericycle origin in most species (Torres-Martínez et al. 2019). Lateral root primordium initiation occurred acropetally in the primary roots of Arabidopsis, where the auxin minimum at the region close to the root apical meristem is required for a LR initiation (Dubrovsky et al. 2006; 2011). Moreover, the cyclic cell death of LR cap cells results in the subsequent increase in auxin levels, and this local auxin gradient determines the timing of LR initiation in Arabidopsis (Xuan et al. 2016). Although the source of auxin remains unclear, the reduction and subsequent increase of auxin level is also essential to determine the pattern of acropetal LR formation in rice crown roots (Yamauchi et al. 2019). Lateral root initiation index, which defined as number of LRs and/or primordia formed in a root portion corresponding to a file of 100 cortical cells in the primary roots of Arabidopsis, is proposed to compensate variations of the root growths among different genotypes (Dubrovsky et al. 2009). However, the multiple cortical cell layers might prevent the accurate evaluation of LR initiation index in rice and other plant species.
The mechanisms underlying LRP initiation have been intensively studied in Arabidopsis (Fukaki et al. 2007; Péret et al. 2009; Lavenus et al. 2013; Motte et al. 2019). However, our understanding of the mechanisms regulating LR size (diameter) remains limited, at least partly, owing to the complexity of regulation in LRP initiation and its subsequent development. In Arabidopsis, the function of the BODENLOS (BDL)/IAA12-MONOPTEROS (MP)/ARF5-dependent module, which is preceded by the function of the SLR/IAA14-ARF7/19-dependent LR initiation module, is necessary for proper LR organogenesis (De Smet et al. 2010). Unlike the lateral rootless phenotypes of the slr-1 and arf7arf19 double mutants, the bdl and mp mutants showed defects in later LR organogenesis (De Smet et al. 2010). These observations suggest that LR development in Arabidopsis can be divided into at least the SLR/IAA14-ARF7/19-dependent earlier phase and BDL/IAA12-MP/ARF5-dependent later phase.
In rice, thick first-order LRs with well-developed vasculature form thinner second-order LRs that do not form further branches (Lucob-Agustin et al. 2020; Hasegawa et al. 2021). Recently, Kawai et al. (2022a) showed that regulation of auxin distribution in the LRP, which is determined by PIN-FORMED (PIN) efflux carrier proteins (Adamowski and Friml 2015), is necessary for regulating LR diameters in rice. They also showed that thick LRs require more time to establish LRP than that required by thinner LRs (Kawai et al. 2022b). In Arabidopsis, failure of the apical localization of PIN1, which is caused by disruption of endosomal trafficking, prevents auxin accumulation in the apical region of LRP, thereby increasing LR diameters (Tanaka et al. 2013). Increased LR diameter is detected in Arabidopsis upon overexpression of a gene encoding PINOID (PID), which phosphorylates PIN proteins and stimulates rootward-to-shootward shifts in PIN localization (Friml et al. 2004; Weller et al. 2017). During the establishment of thick LRP in rice, greater auxin levels were detected in the basal parts, and the shootward-to-rootward shift in auxin distribution was observed immediately before the establishment of LRP (Kawai et al. 2022b).
In the present study, we addressed the following questions. (i) Is modeling-based age-dependent analysis effective for evaluating LR development? (ii) How are LRP initiation and LR growth (expansion and elongation) regulated through auxin signaling and transport? To this end, we counted LR and LRP numbers (LRNs) and measured LR and LRP diameters (LRDs) and lengths (LRLs) in the crown roots of the iaa13 mutant and its background WT. Moreover, the longitudinal patterns of LRNs, LRDs, and LRLs were fitted to the regression models, and the distances from the root apices were converted to the times after the root cells entering the DZs. Then, these patterns were compared with the expression of genes related to auxin signaling and transport. Finally, LRNs, LRDs, and LRLs in the arf11 mutant, which defects in the ARF11 function, were evaluated. Overall, we demonstrated the efficacy of age-dependent analysis for understanding the stepwise control of auxin-mediated LR development in rice.
Results
Age-dependent LR development in the WT and iaa13
Twenty-day-old aerobically grown WT (cv. Taichung65; T65) and iaa13 seedlings were further grown under aerated conditions for 48 h, and the LR and LRP numbers (LRNs) were counted, and LR and LRP diameters (LRDs) and lengths (LRLs) were measured every 10 mm (±5 mm) from the crown root apices (Fig. 1, A to D, and Supplemental Fig. S1). The LRNs were significantly smaller in iaa13 than those in the WT at all positions measured (Fig. 1E). Although the LR development in the crown roots of the WT and iaa13 is acropetal (Fig. 1E), LRNs at the most basal parts of the crown roots, i.e. at 10 mm (±5 mm) from the shoot-root junctions were significantly smaller than those at 50 mm from the apices (Supplemental Fig. S2). Even though these results indicate that LR formation is suppressed at the early time points of the crown root development, the LRNs at 10 mm from the shoot-root junctions were also significantly reduced in iaa13 (Supplemental Fig. S2). Interestingly, the LRDs in the WT and iaa13 were comparable at 10 to 30 mm from the apices of the crown roots, whereas those in iaa13 were significantly larger than those in the WT at 40 and 50 mm (Fig. 1F). At 50 mm from the apices of crown roots, LRLs in iaa13 were significantly shorter than those in the WT (Fig. 1G).
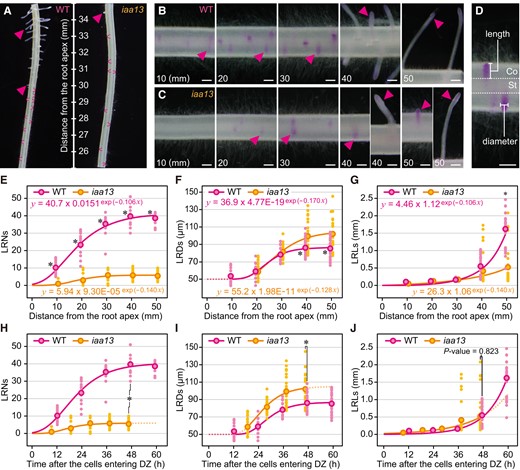
Age-dependent analysis of lateral root (LR) development in crown roots of the wild-type (WT) and iaa13 seedlings. Twenty-day-old aerobically grown WT and iaa13 seedlings were further grown under aerated conditions for 48 h. A) Pictures of LR primordium (LRP) and LR formations in the WT and iaa13 at 25 to 35 mm from the apicies of crown roots. Pictures of Feulgen-stained LRs at 10, 20, 30, 40, and 50 mm from the apicies of crown roots of the WT (B) and iaa13(C). B, C) All images are displayed in the same scale (bars = 200 µm). D) Methods for measuring LR and LRP diameters (LRDs), and lengths (LRLs). For LRDs, we always measured at positions where LRDs are largest. Bar = 200 µm. Gompertz curve fittings for LR and LRP numbers (LRNs) E) and LRDs F) at 10, 20, 30, 40, and 50 mm (±5 mm). G) Exponential curve fitting for the LRLs at 10, 20, 30, 40, and 50 mm (±5 mm). E to G) Significant differences in each parameter at each position of the crown roots between the WT and iaa13 at P < 0.001 are denoted by * (two-sample t test). Time-dependent increases in the LRNs H), LRDs I), and LRLs J) until 60 h after the crown root cells entering differentiation zones (DZs). Gompertz and exponential models were respectively fitted using the SSgompertz and nls functions in R software (version 4.3.1) with all values of the LRNs, LRDs, and LRLs as the response variables and distances from the root tips as explanatory variables. H, I) Significant differences in each parameter at ∼48 h after the crown root cells entering DZs between the WT and iaa13 at P < 0.001 are denoted by * (two-sample t test). E to J) Each dot indicates each value of the LRNs (n = 15), LRDs, and LRLs (n = 27), and the dots with colored borders indicate mean values.
To evaluate the age-dependent patterns of LR initiation and growth, nonlinear models were fitted to the LRNs, LRDs, and LRLs in the crown roots at 10, 20, 30, 40, and 50 mm of the WT and iaa13 (Fig. 1, E to G). The Gompertz models accurately predicted the patterns of LRNs and LRDs (Fig. 1, E and F), whereas the exponential models accurately predicted the patterns of LRLs (Fig. 1G). To convert the distance from the root apices to the time after the root cells entering the DZs, the distances from the root apices at every 1 h were calculated based on the root elongation rates (WT; 0.84 mm h−1, iaa13; 1.07 mm h−1; Supplemental Fig. S3). The calculated distance at each time point was then used as an explanatory variable (x value) in the equations obtained with the models (Fig. 1, H to J). Although the LRNs in iaa13 were much smaller than those in the WT at all time points as was the case with distance-dependent analysis (Fig. 1H), the age-dependent analysis of LRDs identified more apparent differences between WT and iaa13 than those with distance-dependent analysis (Fig. 1, F and I). The differences in LRLs between WT and iaa13 were not significant within ∼48 h after the root cells entering the DZ, whereas the predicted patterns (later at ∼48 h which corresponds to the position at 50 mm from the root apices) showed that the increase in LRLs in iaa13 was retarded compared with the WT (Fig. 1J). To identify the patterns of LRLs later at ∼48 h, we measured LRLs at 10 to 60 mm and at 80 mm from the root apices and then fitted by the Gompertz models (Supplemental Fig. S4A). The results indicate that the increases in the LRLs were apparently retarded in iaa13 until 72 h after the root cells entering the DZ, even though the larger LRP sizes would increase the basal levels of LRLs in iaa13 (Supplemental Fig. S4B).
The age-dependent analysis of LRLs suggest that LR development was retarded in iaa13 (Fig. 1J). To further evaluate the patterns of LR development between the WT and iaa13, we counted the numbers of LRPs and LRs separately (Supplemental Fig. S5, A and B). The LRP numbers peaked at 20 and 30 mm in the WT and iaa13, respectively, and gradually decreased thereafter (Supplemental Fig. S5A). The patterns of LRP numbers could be fitted by the Gaussian models (Supplemental Fig. S5A). The patterns of LR numbers resembled to those of the LRNs (total of the LR and LRP numbers; Fig. 1E), and the increases in LR numbers were first detected at 20 and 40 mm in the WT and iaa13, respectively (Supplemental Fig. S5B). The age-dependent analyses revealed that LRP numbers more steeply increased and decreased in the WT than in iaa13, and the LR numbers rapidly increased in the WT (Supplemental Fig. S5, C and D). These results suggest that duration of the LRP establishment in iaa13 is longer than that in the WT.
Rates of increases in LR(P) numbers, diameters, and lengths in the WT and iaa13
To further understand the stepwise processes in LR development, the increased rates of LRNs, LRDs, and LRLs were calculated at each position from the root apex (distance-dependent; Fig. 2, A to C). The peaks of the increased rates of LRNs (WT: 1.50 mm−1 at 14 mm; iaa13: 0.30 mm−1 at 17 mm) and LRDs (WT: 2.30 µm mm−1 at 23 mm; iaa13: 2.59 µm mm−1 at 26 mm) preceded those of the LRDs and LRLs (WT: 173.41 µm mm−1 at 50 mm; iaa13: 27.16 µm mm−1 at 50 mm), respectively (Fig. 2, A to C). The positions of the peaks of LRNs (at 17 mm) and LRDs (at 26 mm) in iaa13 were farther from the root apex than those in the WT (LRNs: at 14 mm; LRDs: at 23 mm; Fig. 2, A and B). The peak values of LRNs and LRLs were much lower in iaa13, whereas the peak values of LRDs were not largely different between the WT and iaa13 (Fig. 2, A to C).
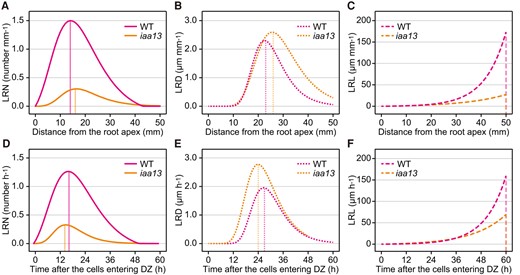
Comparison of the rates of increases in lateral root (LR) and LR primordium (LRP) numbers (LRNs), diameters (LRDs), and lengths (LRLs) in crown roots of the wild-type (WT) and iaa13 seedlings. Distance-dependent rates of the increases in LRNs A), LRDs B), and LRLs C) in crown roots of the WT and iaa13 were calculated as the increase in each parameter at each 1 mm. Age-dependent rates of the increases in LRNs D), LRDs E), and LRLs F) in crown roots of the WT and iaa13 were calculated as the increase of each parameter at each 1 h after the crown root cells entering differentiation zones (DZs). The vertical lines indicate the positions A, B, C) or time points D, E, F) showing the maximum rates of parameter increases.
Subsequently, the increased rates of LRNs, LRDs, and LRLs at each time point after the crown root cells entering the DZs were calculated (age-dependent; Fig. 2, D to F). Although the order of the peaks of LRNs (WT: 1.26 h−1 at 17 h; iaa13: 0.33 h−1 at 15 h), LRDs (WT: 1.94 µm h−1 at 27 h; iaa13: 2.78 µm h−1 at 24 h), and LRLs (WT: 159.72 µm h−1 at 60 h; iaa13: 25.28 µm h−1 at 60 h) was the same as that of the distance-dependent patterns, the peaks of LRNs (at 15 h) and LRDs (at 24 h) in iaa13 were earlier than those in the WT (LRNs: at 17 h; LRDs: at 27 h; Fig. 2, D and E). Interestingly, the peak value of the increased rate of LRD in iaa13 (2.78 µm h−1) was apparently higher than that in the WT (1.94 µm h−1; Fig. 2E). Although the increased rates of LRLs were almost comparable between the WT and iaa13 until 36 h, the rates increased more rapidly in the WT thereafter (Fig. 2F). These results suggest that IAA13 mainly functions in the initiation of LRP, and directly or indirectly affects the establishment of LRP and LR growth.
Age-dependent expression of auxin-related genes in the WT and iaa13
We previously showed that IAA13- and ARF19-mediated auxin signaling determines crown root identity (Yamauchi et al. 2019). The age-dependent transcriptional patterns of IAA13 and ARF19 (and the other auxin-related genes) were determined based on their distance-dependent transcriptional patterns (Supplemental Fig. S6), which were then compared with the normalized ratio of the rates of LRNs, LRDs, and LRLs increases (Fig. 3). Consistent with their functions, the transcript levels of IAA13 and ARF19 peaked at the earliest time point (∼3 h), which preceded the peak of the increased rate of LRNs in the WT (Fig. 3, A and B). Because the IAA13 and ARF19 have a role in the auxin signaling at the apical part of the crown roots (Yamauchi et al. 2019), their expression levels were not strongly affected by the dominant negative IAA13 protein in iaa13 at the earliest time point (Fig. 3, A and B).
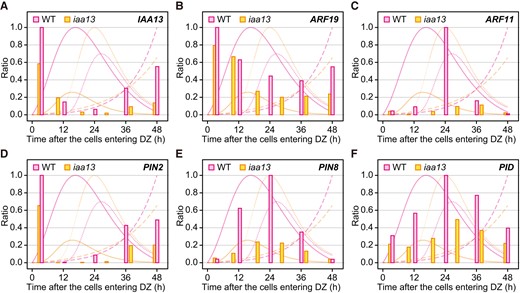
Age-dependent expression analysis of auxin-related genes in crown roots of the wild-type (WT) and iaa13 seedlings. Mean values of the relative transcript levels of IAA13A), ARF19B), ARF11C), PIN2D), PIN8E), and PIDF) at each time point (n = 3). The transcript levels at 0 to 5, 5 to 15, 15 to 25, 25 to 35, and 35 to 45 mm from the root apices of the WT and at 0 to 5, 5 to 15, 15 to 25, 25 to 35, 35 to 45, and 45 to 55 mm of iaa13 roots (Supplemental Fig. S6) were converted to each time point after the crown root cells entering differentiation zones (DZs). The maximum transcript level of each gene in the WT or iaa13 was set as 1.0, and the relative values were calculated accordingly. The rates of time-dependent increases in the lateral root and lateral root primordia numbers (solid lines), diameters (narrow dashed lines), and lengths (wide dashed lines) of the WT and iaa13 are shown in the background of each panel.
We had previously identified genes whose transcript levels were significantly decreased in iaa13 (Yamauchi et al. 2019). Among them, the transcription factor ARF11, which is a homolog of MP/ARF5 in Arabidopsis (Wang et al. 2007), and the auxin-transport genes PIN2, PIN8, and PID were selected for further analyses (Supplemental Table S1) because auxin signaling and distribution patterns are important for LR development (Benková et al. 2003; Adamowski and Friml 2015). The transcriptional pattern of PIN2 resembled that of IAA13 (Fig. 3D), suggesting that PIN2 also functions in the determination of crown root identity. The transcript level of ARF11 showed a strong peak at ∼24 h after the root cells entering the DZ in the WT, but it severely decreased in iaa13 (Fig. 3C). The peak of ARF11 transcription in the WT was almost the same as the peak of LRDs (∼27 h; Fig. 3C), suggesting that ARF11 expression peaks at the later stage of LRP formation. The transcript levels of PIN8 and PID in the WT gradually increased until at ∼24 h, suggesting that PIN8 and PID function in regulating the increase in LRDs (Fig. 3, E and F). The transcript levels of PIN8 and PID in iaa13 showed less obvious peaks than those in the WT (Fig. 3, E and F).
LRP-specific expression of auxin-related genes in the WT
To investigate the tissue(organ)-specific transcription of auxin-related genes, the cortex (Co), central cylinder (CC), and LRP were isolated from the crown root segments at 20 mm (±2 mm) from the root apices of the WT, which corresponds to ∼24 h after the root cells entering the DZ, using laser microdissection (Fig. 4, and Supplemental Fig. S7). As previously described, the transcript levels of IAA13 and ARF19 were higher in Co and LRP than those in the CC (Supplemental Fig. S7; Yamauchi et al. 2019). The transcript levels of ARF11, PIN2, PIN8, and PID were much higher in the LRP than in the Co and CC (Fig. 4), even though the average transcript level of PIN2 was slightly higher in the Co than in the CC (Fig. 4B). These results further support the idea that ARF11, PIN8, and PID mainly function in LR development.
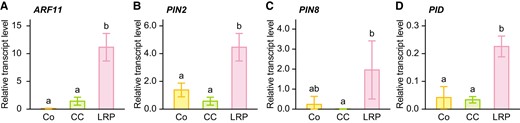
Tissue (organ)-specific expression analysis of auxin-related genes in crown roots of the wild-type (WT) seedlings. Relative transcript levels of ARF11(A), PIN2(B), PIN8(C), and PID(D) in the cortex (Co), central cylinder (CC), and lateral root primordium (LRP). Each tissue was isolated from paraffin-embedded sections of the crown roots at 20 mm (±2 mm), corresponding to ∼24 h (± 6 h) after the crown root cells entering the differentiation zone, using laser microdissection. The gene encoding transcription initiation factor IIE was used as an internal control. Values are means ± SD (n = 3). Different lower-case letters denote significant differences among different tissues (P < 0.05, one-way ANOVA followed by Tukey's test for multiple comparisons).
Expression patterns of auxin-related genes in a single LR of the WT and iaa13
As the transcript levels of ARF11, PIN8, and PID were LR-specific (Fig. 4, A, C, and D), those in each individual LR could be masked by the smaller LRNs in iaa13 (Fig. 5A). To reveal the transcriptional patterns in a single LR, the transcript levels of ARF11, PIN2, PIN8, and PID, as well as the auxin-responsive SMALL AUXIN-UP RNA (SAUR22; Supplemental Table S1; Yamauchi et al. 2019) were normalized to LRNs at the same time point. As most tip parts of crown roots include apical meristems and have no LRs, we used the data from later than ∼12 h after the root cells entering the DZ (Fig. 5, B to F). The transcript level in a single LR of ARF11 was peaked at ∼24 h in the WT, whereas the peak was retarded and weaken in iaa13 (Fig. 5B). The transcriptional patterns in a single LR of PIN2 resembled those of SAUR22 (Fig. 5, C and F), suggesting that PIN2 transcription was induced by auxin. Interestingly, the transcript levels of PIN2 and SAUR22 were much higher in iaa13 than in the WT after ∼36 h (Fig. 5, C and F). These results suggest that the auxin level in a single LR is higher in the iaa13 than that in the WT. By contrast, the transcript level in a single LR of PIN8 showed similar patterns between the WT and iaa13 (Fig. 5D). The transcript level of PID in a single LR peaked at ∼12 h and gradually decreased thereafter with time in the WT, whereas that of PID in iaa13 at ∼18 h was higher than the peak level in the WT and remained high at the later time points (Fig. 5E). These results suggest that ARF11 mainly contributes to the later stage of LRP formation and subsequent LR growth, whereas PID contributes to the increase of LRD.
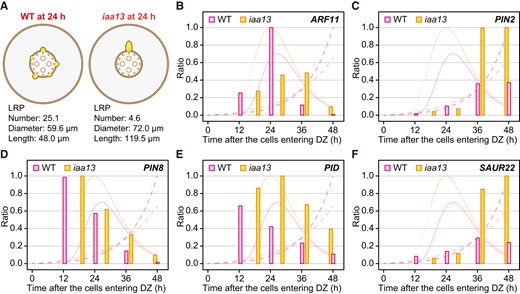
Expression analysis of auxin-related genes in each individual lateral root (LR) or LR primordium (LRP). A) Schematic diagrams of LR development in crown roots of the wild type (WT) and iaa13 seedlings at 24 h after the root cells entering the differentiation zones (DZs). LRPs are depicted based on the relative differences in LR and LRP numbers, diameters, and lengths in the WT and iaa13. The transcript levels of ARF11(B), PIN2(C), PIN8(D), PID(E), and SAUR22 (F) in the crown roots of the WT and iaa13 normalized to the LR and LRP numbers (LRNs; Fig. 1H) at each time point. The maximum transcript levels of each gene in the WT or iaa13 were set as 1.0 and the relative values were calculated accordingly. Only the data at time points when the LRNs are higher than 1.0 was used for the experiment. B to F) The rates of the time-dependent increases in the LR and LRP diameters (narrow dashed lines) and lengths (wide dashed lines) of the WT and iaa13 are shown in the background of each panel.
LR-related phenotypes of the arf11 mutant
To understand the ARF11 functions in LR development in the crown roots of rice, the LR-related phenotypes of the homozygous arf11 mutant (arf11; arf11/arf11; cv. Nipponbare background), which has a Tos17 retrotransposon insertion in the fifth exon of the ARF11 gene (Sims et al. 2021), were compared with the segregated wild-type (WT; ARF11/ARF11; Fig. 6, A and B). As the elongation rates of the crown roots were comparable between the WT (1.09 mm h−1) and arf11 (1.08 mm h−1; Fig. 6C), LR-related phenotypes were analyzed at 50 mm (±5 mm) from the root apices (at ∼48 h after the root cells entering the DZ). As a result, we found that LRN in arf11 was smaller than that in the WT (Fig. 6D). Although LRDs were not significantly different between the WT and arf11 (Fig. 6E), LRL was significantly shorter in the arf11 (Fig. 6F). These results suggest that rice ARF11 mainly functions in the later stage of LRP formation and subsequent LR growth.
![Analysis of lateral root (LR) and LR primordium (LRP) development in crown roots of the homozygous arf11 mutant (arf11; arf11/arf11) and segregated wild-type (WT; ARF11/ARF11) seedlings. Twenty-day-old aerobically grown WT and arf11 seedlings were further grown under aerated conditions for 48 h. Pictures of LR and LRP formations in the WT (A) and arf11(B) at 50 mm from the apices of crown roots. Bars = 1 mm. C) Root elongation rates of the crown roots of the WT and arf11. Lateral root and LRP numbers [LRNs D)], diameters [LRDs E)], and lengths [LRLs F)] in the crown roots of the WT and arf11 at 50 mm (±5 mm) from the apices of crown roots, which corresponds to at ∼48 h after the root cells entering the differentiation zones. Boxplots show the median (horizontal lines), 25th to 75th percentiles (edges of the boxes), minimum to maximum values (edges of the whiskers), and mean values (dots with black borders in the boxes). Each dot indicates the values of root elongation rates [n = 8 C)], LRNs [n = 8 D)], LRDs [n = 24 E)], and LRLs [n = 24 F)]. Significant differences between the genotypes at P < 0.05 are denoted by * (two-sample t test).](https://oup.silverchair-cdn.com/oup/backfile/Content_public/Journal/plphys/194/2/10.1093_plphys_kiad548/1/m_kiad548f6.jpeg?Expires=1747905530&Signature=H0c5xpXWYbYkBM4L9OBfp3J4ePJjYp2wPpjIqTVscN1qFf~Zt9yMCBXL31c-nbUnKmM7OyfPe-CLplloYpRMXPeUiMJUb0G~zYSAYVpPbDKobFZ8kegYUNgvNDincg0CaZJfMPmgrqc4RECyjzInYeqCRN6Goc8~oEnZsxxqYuc4e2nrIZTH6rLIaB4V9Q~HNGaM7ojhO4zb258ULGAKb2oenFVXnPap~15bRosRSKYqZGs0xMUsE4nLCfq0ebxICkHmn05LdkmPSsbJwMQ4ubX~lRf8izNGmmAGLrg~nmo5zbSnVf-TA5-KzwzLkE0TI3OY859mOp569TDtLfLckA__&Key-Pair-Id=APKAIE5G5CRDK6RD3PGA)
Analysis of lateral root (LR) and LR primordium (LRP) development in crown roots of the homozygous arf11 mutant (arf11; arf11/arf11) and segregated wild-type (WT; ARF11/ARF11) seedlings. Twenty-day-old aerobically grown WT and arf11 seedlings were further grown under aerated conditions for 48 h. Pictures of LR and LRP formations in the WT (A) and arf11(B) at 50 mm from the apices of crown roots. Bars = 1 mm. C) Root elongation rates of the crown roots of the WT and arf11. Lateral root and LRP numbers [LRNs D)], diameters [LRDs E)], and lengths [LRLs F)] in the crown roots of the WT and arf11 at 50 mm (±5 mm) from the apices of crown roots, which corresponds to at ∼48 h after the root cells entering the differentiation zones. Boxplots show the median (horizontal lines), 25th to 75th percentiles (edges of the boxes), minimum to maximum values (edges of the whiskers), and mean values (dots with black borders in the boxes). Each dot indicates the values of root elongation rates [n = 8 C)], LRNs [n = 8 D)], LRDs [n = 24 E)], and LRLs [n = 24 F)]. Significant differences between the genotypes at P < 0.05 are denoted by * (two-sample t test).
Discussion
The results of the present study demonstrate that modeling-based age-dependent analysis is an effective method for evaluating the stepwise LR development. The distance-dependent analysis showed that the differences in LRDs and LRLs were first detected at the same position, i.e. at 40 mm from the root apices, between the WT and auxin-signaling-defective mutant, iaa13, which has fewer LRNs in the crown roots (Fig. 1, E to G). Moreover, the peak value of the increased rate of LRD in iaa13 was not largely different from that in the WT (Fig. 2B). However, we considered that the different elongation rates of the crown roots between the WT and iaa13 might lead to misestimation of these parameters (Supplemental Fig. S3). We recently showed that the Gompertz model accurately predicts the longitudinal patterns of aerenchyma formation (Yamauchi et al. 2020). The Gompertz models also well predicted the patterns of LRNs and LRDs, whereas the exponential models were better for LRLs (Fig. 1, E to G). Subsequent age-dependent analyses revealed that LRDs in iaa13 were greater than those in the WT from the early time point (at ∼18 h; Fig. 1I), whereas LRLs were comparable until ∼48 h and were more rapidly increased thereafter in the WT; i.e. the beginning of LR elongation was retarded in iaa13 (Fig. 1J). By comparing the increased rates of LRDs, we identified an apparently higher rate and a longer duration of LRD increase in iaa13 (Fig. 2D). Recently, Kawai et al. (2022b) showed that thick LRP in the seminal roots of rice require more time to establish apparent apical meristems than thinner LRP. Interestingly, our age-dependent analysis also showed the prolonged duration of the LRD increase in iaa13 with thick LRs compared with that in the WT (Figs. 1, I and 2E). This supports that a longer duration of LRD increase is required for the formation of larger LRP.
Age-dependent expression analyses revealed that IAA13 and ARF19 were predominantly expressed at the earliest time point after the crown root cells entering the DZs (Fig. 3, A and B). Moreover, these genes were expressed more in cortex and LRP than in the central cylinder (Supplemental Fig. S7, A and B). As previously shown, IAA13- and ARF19-dependent auxin signaling is required for LR formation in the pericycle as well as aerenchyma formation in the cortex (Yamauchi et al. 2019). The early peaks of the expression levels of IAA13 and ARF19 further support the idea that IAA13 and ARF19 determine the identity of the crown roots. ARF19 and IAA13 are the homologs of Arabidopsis ARF7/19 and SLR/IAA14, respectively, which are major contributors to LR formation (Fukaki et al. 2002; Okushima et al. 2005). Recently, Lee et al. (2019) demonstrated that ARF7/19 are also key regulators of adventitious root development in Arabidopsis. This evidence further supports that the functions of the IAA13-ARF19-dependent module in rice are conserved with the functions of the ARF7/19-SLR/IAA14-module in Arabidopsis.
A comparison of gene expression levels at 20 mm from the crown root apices, which corresponds to ∼24 h after the root cells entering the DZ, revealed that the expression levels of ARF11 and the auxin-transport genes PIN2, PIN8, and PID were reduced in iaa13 (Fig. 3, C to F, and Supplemental Fig. S6, C to F). The age-dependent expression pattern of PIN2 resembled that of IAA13 (Fig. 3, A and D), even though the relative expression level of PIN2 in the cortex was weaker than that of IAA13 (Fig. 4B and Supplemental Fig. S7A). As rice pin2 mutants show curly root phenotypes and altered patterns of LR formation (Inahashi et al. 2018), PIN2 could function in the control of auxin distribution patterns at the apical part of rice crown roots to determine the identities of the crown-root cells, similar with its function in the primary roots of Arabidopsis (Müller et al. 1998). On the other hand, the expression levels of ARF11, PIN8, and PID showed LRP-specific patterns (Fig. 4, A, C, and D). These results suggest that these genes mainly function in LR development as the downstream of the auxin signaling mediated by IAA13-ARF19.
Because of the LRP-specific expression of ARF11, PIN8, and PID (Fig. 4, A, C, and D) and because of the smaller LRNs in iaa13 (Figs. 1, H and 5A), we hypothesized that the expression levels of these genes in each individual LR(P) are masked in the transcriptional analysis with using crown root segments (Fig. 3). Indeed, the gene expression patterns in a single LR were, to some extent, different from those in the crown root segments (Fig. 5). Interestingly, the expression level of ARF11, a homolog of MP/ARF5 in Arabidopsis (Wang et al. 2007), in a single LR showed a sharp peak at ∼24 h in the WT, whereas the peak was weaker and broader in iaa13 (Fig. 5B). The peak timing of the ARF11 expression (at ∼24 h) was almost the same as that of the rates of LRD increase (at ∼27 h; Fig. 5B). This suggests that ARF11 functions in the later stage of the establishment of LRP and subsequent LR growth rather than the increase of LRD. Thus, the reduced LRN and retarded initiation of the LRL increase in iaa13 might be at least partly caused by the lower expression level of ARF11 (Figs. 1, H and J, and 5B). Indeed, the arf11 mutant showed shorter LRL than the segregated WT (Fig. 6, A, B, and F). Recently, Sims et al. (2021) showed that LRN is reduced in rice arf11 mutant. We confirmed that LRN was reduced in arf11 in our growth conditions (Fig. 6D). So far, we could not conclude that the reduced LRN in arf11 is caused by the defects in the establishment of LRP or the arrest of subsequent LR growth, the latter of which is supported by the reduced LRL in this mutant (Fig. 6F). On the other hand, our results indicate that ARF11 functions downstream of the ARF19-IAA13 module in rice during the LR development as is the case with MP/ARF5 being defined as downstream of ARF7/9-SLR/IAA14 module in Arabidopsis (De Smet et al. 2010). As the ectopic expression of MP/ARF5 in the slr-1 background stimulates the emergence of fused or irregularly spaced LRs (De Smet et al. 2010), there remains a possibility that ARF11 affects LRD only in the iaa13 background. Further studies are needed to understand the detailed function of rice ARF11(MP) in LR development.
Among auxin-transport genes, the expression pattern of PIN8 in a single LR was comparable between the WT and iaa13 (Fig. 5D). Although the possibility that PIN8 contributes to LR development by controlling intracellular auxin distribution patterns cannot be ruled out (Lee et al. 2020), this result indicates that PIN8 does not contribute to the difference in LRDs between the WT and iaa13. On the other hand, the peak of PID expression broadened and increased in iaa13 (Fig. 5E). In Arabidopsis, PID phosphorylates PIN proteins, thereby stimulating rootward-to-shootward shifts in PIN localization (Friml et al. 2004; Weller et al. 2017). During the formation of thick LRP in rice, increased auxin accumulation was detected in the basal part of the LRP (Kawai et al. 2022a). These suggest that the broader and higher PID expression results in a higher auxin distribution to the basal region of each individual LR (Fig. 5E), eventually leading to the increases of LR diameters in iaa13. Interestingly, the shootward-to-rootward shift in auxin distribution is detected immediately before the establishment of LRP (Kawai et al. 2022a). The expression pattern of PID in the WT and iaa13 was negatively associated with those of PIN2 and the auxin-responsive gene SAUR22. As PIN2 expression was higher in the apical part of the roots (Fig. 3D and Supplemental Fig. S6D; Feraru and Friml 2008), gradual decreases in PID expression might be associated with the shootward-to-rootward shift in auxin distribution and the establishment of LRP. Thus, the broader and higher expression level of PID might explain why thick LRP need more time to establish apical meristems than thinner LRP (Kawai et al. 2022a).
In conclusion, we demonstrate that modeling-based age-dependent analysis is an effective approach to evaluate the stepwise control of LR development in rice. Indeed, the results obtained in the analyses provide insights into the molecular mechanisms of LR development. We identified ARF11(MP) as the downstream of the ARF19-IAA13 module and that controls the later LRP development and LR growth. Moreover, we found that establishment-to-elongation switching of LR is essential for determining the diameters of LRP, and PID-dependent shift in the PIN localization is a candidate for controlling this process. Further studies using the modeling-based age-dependent analysis will contribute to our understanding of the developmental processes of roots and other tissues/organs in plants.
Materials and methods
Plant materials and growth conditions
Seeds of the rice (Oryza sativa) iaa13 mutant (Kitomi et al. 2012) and its background WT (cv. T65), and the rice arf11 mutant (Tos17 insertion mutant; NC2659; cv. Nipponbare background; Miyao et al. 2003) and the segregated WT were surface-sterilized in 0.5% (v/v) sodium hypochlorite for 30 min and rinsed thoroughly with deionized water. The seeds were germinated on Petri dishes with deionized water in a growth chamber at 28°C under dark conditions. After 2 days, the germinated seeds were placed on a mesh float with an aerated quarter strength nutrient solution at 28 °C under constant light conditions (photosynthetically active radiation, 200 to 250 μmol m−2 s−1) for 4 days. Six-day-old seedlings were transferred to 5 L pots (8 to 12 plants per pot, 250 mm height × 120 mm length × 180 mm width) containing an aerated full-strength nutrient solution. After 7 days, 13-day-old rice seedlings were transferred to a newly prepared nutrient solution and further grown for seven days. The 20-day-old rice seedlings were then transferred to 5 L pots (8 plants per pot) containing aerated full-strength nutrient solution, and grown for 48 h. The composition of the full-strength nutrient solution has been described by Colmer et al. (2006).
Genotyping of the arf11 mutant
For the genotyping of the arf11 mutant, the total DNAs were extracted from the seedling leaves and used as templates for PCR amplification. To detect the entire Tos17 elements, KOD One PCR Master Mix (TOYOBO) was used for PCR with the ARF11 gene-specific primers (forward: 5′-TTCCGAACTACCCAAACCTG-3′; reverse: 5′-TTCCTTGCACACTGAATTTTTG-3′). The thermal cycling conditions were initial denaturation (94°C for 2 min), and 35 cycles of denaturation (98°C for 10 s), annealing (60°C for 5 s), and extension (68°C for 30 s).
Feulgen staining
To detect LR and LRP among the crown roots of the WT and iaa13 seedlings, 10-mm-long crown root segments were hydrolyzed with 6 N HCl under vacuum for 15 min at room temperature. The crown-root segments were then washed thrice with distilled water and incubated in Schiff's solution (Sigma-Aldrich) for 5 min. The magenta-stained LR and LRP were photographed using an optical microscope (SZX16, OLYMPUS) with a CCD camera (DP71, OLYMPUS).
Regression model analysis
The Gompertz models or exponential models were fitted to the dataset of LRNs, LRDs, or LRLs in the crown roots of the WT or iaa13 using standard statistical tools in R software (version 4.3.1; https://rdrr.io/r/stats/stats-package.html). The Gaussian models were fitted to the dataset of LRP numbers in the crown roots of the WT or iaa13 using gausspr function in the kernlab package in R software (version 4.3.1). For regression model fitting, all values of LRNs, LRDs, LRLs, LR numbers, or LRP numbers in the crown roots of the WT or iaa13 were used as response variables and distance from the crown root apices were used as explanatory variables.
Conversion of distance-dependent to time-dependent data
The values of LRNs, LRDs, and LRLs at each time point were calculated using the equations obtained from the regression models. The distance from the crown root tips at each time point, which was calculated based on the root elongation rates (WT; 0.84 mm h−1, iaa13; 1.07 mm h−1; Supplemental Fig. S3), was used as the explanatory variable in the equations. The positions at 10, 20, 30, 40, 50, 60, and 80 mm from the apices of crown roots were converted to 11.8, 23.7, 35.5, 47.4, 59.2, 71.1, and 94.7 h (WT), and 9.3, 18.7, 28.0, 37.4, 46.7, 56.0, and 74.7 h (iaa13) after the root cells entering the DZs. The curve graphs for the time-dependent data were depicted based on the calculations of the LRNs, LRDs, and LRLs at each time point.
Microarray data analysis
Microarray data were deposited in the Gene Expression Omnibus (GEO; http://www.ncbi.nlm.nih.gov/geo/) under accession number GSE130131 (Yamauchi et al. 2019). Total RNA was extracted from 10 mm long crown root segments at 15 to 25 mm from the apices. For microarray data analysis, the Benjamini–Hochberg false discovery rate method was used to obtain corrected P-values for multiple testing. The fold change of each probe between two conditions was calculated using an average of three biological replicates (Yamauchi et al. 2019).
Laser microdissection
Crown root segments 18 to 22 mm from the apices of crown roots were fixed in 100% methanol and embedded in paraffin and sectioned at a thickness of 16 µm. The serial root sections were placed onto PEN membrane glass slides (Thermo Fisher Scientific) for laser microdissection as described by Takahashi et al. (2010). The cortex (Co), central cylinder (CC) and LRP were collected from the tissue sections using a Veritas Laser Microdissection System LCC1704 (Thermo Fisher Scientific). Total RNA was extracted from each tissue section using a PicoPure RNA isolation kit (Thermo Fisher Scientific) according to the manufacturer's instructions. The extracted total RNA was quantified using a Quant-iT RiboGreen RNA Assay Kit (Thermo Fisher Scientific), and the quality was evaluated using an RNA6000 Pico kit on an Agilent 2100 Bioanalyzer (Agilent Technologies) according to the manufacturers' instructions.
RT-qPCR analysis
Root segments at 0 to 5, 5 to 15, 15 to 25, 25 to 35, 35 to 45, and 45 to 55 mm from the apices of crown roots were ground in liquid nitrogen. Total RNA was extracted from frozen fixed root tissues using an RNeasy Plant Mini Kit (QIAGEN) according to the manufacturer's instructions. For RT-qPCR, 1 ng of total RNA was used as the templates. Transcript levels were measured using a StepOnePlus Real-Time PCR System (Applied Biosystems) and the One Step SYBR PrimeScript RT-PCR Kit II (Takara Bio), as described by Yamauchi et al. (2017). Transcript levels were normalized to the expression level of TFIIE (transcription initiation factor IIE). The primer sequences used for RT-qPCR are listed in Supplemental Table S1. For the conversion of distance-dependent to time-dependent data, the expression levels of genes at each position from the apices of crown roots were converted to each time point after the crown root cells entering the DZs as described in the section “Conversion of distance-dependent to time-dependent data”.
Statistical analyses
Statistical differences between means were calculated using two-sample t-tests. For multiple comparisons, data were analyzed using one-way analysis of variance (ANOVA) and post hoc Tukey's test using SPSS Statistics version 25 (IBM Software).
Accession numbers
Sequence data from this article can be found in the RAP-DB and MSU databases as listed in Supplemental Table S1.
Acknowledgments
The Tos17 line NC2659 was kindly provided by the National Agriculture and Food Research Organization. We thank Masako Hattori for her technical assistance.
Author contributions
T.Y. and A.T. performed the experiments and analyzed the data; T.Y. designed the research and wrote the manuscript with the contribution of M.N. and Y.I.
Supplemental data
The following materials are available in the online version of this article.
The following materials are available in the online version of this article.
Supplemental Figure S1. Representative images of lateral root (LR) development in crown roots of the wild-type (WT) and iaa13 seedlings.
Supplemental Figure S2. Analysis of lateral root (LR) and LR primordium (LRP) numbers at the basal parts of the crown roots of the wild type (WT) and iaa13 seedlings.
Supplemental Figure S3. Elongation rates of crown roots of the wild-type (WT) and iaa13 seedlings.
Supplemental Figure S4. Age-dependent analysis of lateral root (LR) and LR primordium (LRP) lengths in crown roots of the wild-type (WT) and iaa13 seedlings.
Supplemental Figure S5. Age-dependent analyses of lateral root (LR) numbers and LR primordium (LRP) numbers in crown roots of the wild-type (WT) and iaa13 seedlings.
Supplemental Figure S6. Distance-dependent expression analysis of auxin-related genes in crown roots of the wild-type (WT) and iaa13 seedlings.
Supplemental Figure S7. Tissue (organ)-specific expression analysis of IAA13 and ARF19 in crown roots of the wild-type (WT) seedlings.
Supplemental Table S1. List of auxin-related genes and primers used for RT-qPCR analysis.
Funding
This work was partly supported by the Grant-in-Aid for Transformative Research Areas (A) (Ministry of Education, Culture, Sports, Science and Technology KAKENHI Grant Numbers 23H04195 to T.Y. and 20H05912 to M.N.), Grant-in-Aid for Scientific Research on Innovative Areas (Japan Society for the Promotion of Science KAKENHI Grant Number 22H04715 to Y.I.) and Japan Science and Technology Agency (PRESTO Grants JPMJPR17Q8 to T.Y. and JPMJPR15Q3 to Y.I.).
Data availability
The authors confirm that all experimental data are available and accessible via the main text and/or the supplemental data.
Dive Curated Terms
The following phenotypic, genotypic, and functional terms are of significance to the work described in this paper:
References
Author notes
The author responsible for distribution of materials integral to the findings presented in this article in accordance with the policy described in the Instructions for Authors (https://dbpia.nl.go.kr/plphys/pages/General-Instructions) is Takaki Yamauchi ([email protected]).
Conflict of interest statement. The authors declare that they have no conflicts of interest.