-
PDF
- Split View
-
Views
-
Cite
Cite
Xiaoxia Yang, Yufen Che, Veder J García, Jianqiang Shen, Yutong Zheng, Zhezheng Su, Li Zhu, Sheng Luan, Xin Hou, Cyclophilin 37 maintains electron transport via the cytochrome b6/f complex under high light in Arabidopsis, Plant Physiology, Volume 192, Issue 4, August 2023, Pages 2803–2821, https://doi.org/10.1093/plphys/kiad268
- Share Icon Share
Abstract
Plants have evolved multiple mechanisms to cope with diverse types of light stress, particularly the regulation of the electron transport chain (ETC). Under high light (HL) conditions, the balance of electron flux in the ETC is disturbed, which leads to the overaccumulation of reactive oxygen species (ROS) and results in photodamage and photoinhibition. The cytochrome (Cyt) b6/f complex, which coordinates electron transfer between photosystems I and II (PSI and PSII), plays an essential role in regulating the ETC and initiating photoprotection. However, how the Cyt b6/f complex is maintained under HL conditions remains unclear. Here, we report that the activity of the Cyt b6/f complex is sustained by thylakoid-localized cyclophilin 37 (CYP37) in Arabidopsis (Arabidopsis thaliana). Compared with wild-type plants, cyp37 mutants displayed an imbalance in electron transport from Cyt b6/f to PSI under HL stress, which led to increased ROS accumulation, decreased anthocyanin biosynthesis, and increased chlorophyll degradation. Surprisingly, CYP37's role in regulating ETC balance was independent of photosynthesis control, which was indicated by a higher Y (ND), an indicator of P700 oxidation in PSI. Furthermore, the interaction between CYP37 and photosynthetic electron transfer A (PetA), a subunit of the Cyt b6/f complex, suggests that the central function of CYP37 is to maintain Cyt b6/f complex activity rather than to serve as an assembly factor. Our study provides insights into how plants balance electron flow between PSII and PSI via Cyt b6/f complex under HL.
Introduction
Photosynthesis harvests energy from sunlight to synthesize carbohydrates. First, sunlight is absorbed by the light-harvesting complexes; then, the energy is transferred to the reaction center of photosystems, where electrons are produced by charge separation. Photosystem II (PSII) and photosystem I (PSI) work coordinately for efficient electron transfer and the formation of ATP and NADPH that are required for the metabolic processes for carbohydrate synthesis, and the Cyt b6/f complex is an intermediary, transferring the electrons from PSII to PSI (Moustakas et al. 2022).
In oxygenic photosynthesis, electron transport occurs mainly via linear electron transport (LET) driven by PSII and PSI and via cyclic electron transport (CET) driven by PSI alone. While LET generates both ATP and NADPH, CET around PSI is exclusively involved in ATP synthesis without the net production of NADPH (Munekage et al. 2002; Yamori and Shikanai 2016). In addition, pseudo-CET (the water‒water cycle) coordinates with CET to protect PSI against photodamage (Sun et al. 2021). In pseudo-CET, electrons are transferred from water in PSII, via the Cyt b6/f complex, to PSI, where it produces superoxide (Osmond et al. 2000). The reactive oxygen species (ROS) are scavenged by superoxide dismutase (SOD) and ascorbate peroxidase (APX), the reducing equivalents NAPDH are consumed, and ascorbate is regenerated, thus avoiding an overreduction of PSI at the acceptor side (Endo and Asada 2006).
When the balance of electrons between PSII and PSI is disturbed, various ROS are produced, especially under excessive amounts of light (Dietz et al. 2016; Foyer and Hanke 2022). Under high light (HL) conditions, the accumulated ROS will damage photosystems and thylakoid membranes, impairing redox homeostasis. This phenomenon is known as “photoinhibition”, and it can inactivate either or both photosystems (Powles 1984; Aro et al. 1993; Gururani et al. 2015). Photoinhibition dramatically decreases photosynthesis performance and plant growth (Takahashi and Murata 2008; Kato et al. 2012; Simkin et al. 2017). PSII is extremely sensitive to light-induced oxidative inactivation, but the PSII repair system can efficiently reverse inactivation (Aro et al. 1993; Yokthongwattana and Melis 2006). PSI is also susceptible to light-induced inhibition, but no repair system has been identified for PSI photoinhibition. Thus, PSI photoinhibition is more deleterious to plant fitness since the PSI recovery process is much longer and slower (Kudoh and Sonoike 2002; Li et al. 2004; Huang et al. 2010).
PSI photoinhibition occurs when the PSI acceptor side is overreduced and O2 is utilized as the alternative electron acceptor. In this case, the radical superoxide (O2•−) will be produced and subsequently disproportionated to hydrogen peroxide (H2O2) and O2 (Mehler 1951; Asada et al. 1974; Takagi et al. 2016; Kozuleva et al. 2021). The produced H2O2 can react with the Fe/S clusters, resulting in the formation of hydroxyl radicals (•OH), which inactivates PSI electron transport (Sonoike et al. 1997; Sonoike 2011).
Since the level of PSI photoinhibition is closely related to the energy balance between the donor and acceptor sides of PSI, plants have developed protection mechanisms for PSI photoinhibition on both sides (Shimakawa and Miyake 2018; Lima-Melo et al. 2021). On the PSI donor side, protection mechanisms include the downregulation of PSII, induction of nonphotochemical quenching (NPQ), and restriction of electron flow through Cyt b6/f (Tikkanen and Aro 2014). These actions serve to downregulate the electron flow to the PSI donor side, thus reducing the PSI acceptor-side overreduction, as well as O2 reduction. Furthermore, electron transport regulation by the Cyt b6/f complex is considered to be the most important protection mechanism against photoinhibition. It is known as “photosynthetic control”, a process triggered by the acidification of the thylakoid lumen. In particular, it protects against the overreduction of the PSI donor and acceptor sides and maintains the CO2 assimilation rate during the beginning of plant adaptation to HL stress (Price et al. 1995, 1998; Ruuska et al. 2000; Tikkanen et al. 2015; Rantala et al. 2020).
The Cyt b6/f complex's ability to fine-tune the electron transport capacity comes from its central role in the electron transport chain (ETC), as it functions in both LET and CET (Price et al. 1995, 1998; Yamori et al. 2011). The Cyt b6/f complex is the smallest redox-active complex of the ETC, and its structure has been elucidated previously (Kurisu et al. 2003; Stroebel et al. 2003; Baniulis et al. 2009; Malone et al. 2019). It functions as a dimer, and each monomer consists of 8 subunits that are essential for the function and accumulation of the Cyt b6/f complex (Cramer et al. 2006; Baniulis et al. 2009). Among the 8 subunits, PetA (photosynthetic electron transfer A, also known as cytochrome f) is the largest, with a molecular mass of 31 kDa, and it transfers electrons from PetC (Rieske iron–sulfur protein) to plastocyanin (PC) in the thylakoid lumen (Luján et al. 2017; Malone et al. 2021). The assembly factor of the Cyt b6/f complex has been identified recently (Sandoval-Ibanez et al. 2022). However, little is known about the auxiliary factors that assist the Cyt b6/f complex in regulating the ETC, particularly under HL conditions.
Here, we identified an auxiliary factor of the Cyt b6/f complex that is critical in HL conditions in Arabidopsis (Arabidopsis thaliana). The associated gene cyclophilin 37 (CYP37) belongs to the plant cyclophilin gene family and is highly responsive to HL. CYP37 interacts with PetA, the essential subunit for the full function of the Cyt b6/f complex. In addition, under HL conditions, the loss of CYP37 strongly impaired anthocyanin accumulation and increased the production of ROS. Furthermore, it led to photoinhibition of both PSII and PSI, a lower electron transport rate (ETR) around PSII and PSI, and inhibition of electron transport at the PSI donor side. These effects were caused by defects in the Cyt b6/f complex's function, rather than in its assembly or abundance.
Results
Chloroplast thylakoid lumen-localized CYP37 responds to HL exposure
To investigate the function of CYP37 in Arabidopsis, we bioinformatically analyzed potential cis-regulatory elements (CREs) in the 2,000 bp upstream of the CYP37 translational start site. Multiple CREs involved in phytohormone and environmental responses were predicted in this region, among which light-responsive elements were in the majority (Fig. 1A). These results indicate that CYP37 potentially plays a role in responding to environmental changes, specifically to HL stimuli. To confirm the prediction, we determined CYP37 transcripts after HL exposure in wild-type (WT) plants. The CYP37 transcripts started to accumulate after 3 h of HL exposure and maintained a significantly higher expression level compared with what was observed in growth light (GL) conditions (Fig. 1B). Besides, the CYP37 protein levels were also increased after HL treatment (Supplemental Fig. S1). These results imply that the transcription of CYP37 may respond to HL stimuli.
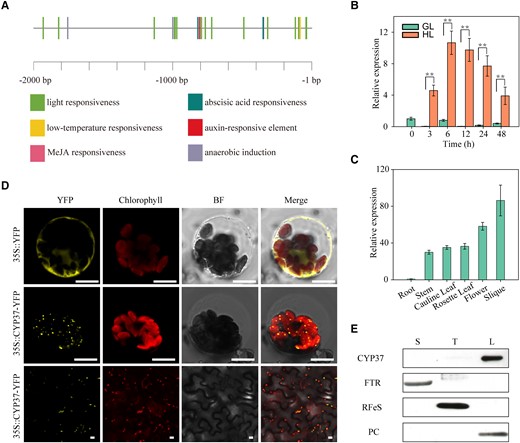
The expression of thylakoid lumen-localized CYP37 was induced under HL conditions. A) CRE prediction 2,000 bp upstream of the CYP37 translational start site. Putative phytohormone and environmental stimuli-inducing CREs were identified in the PlantCARE database (https://bioinformatics.psb.ugent.be/webtools/plantcare/html/). B)CYP37 transcripts were induced by HL. The transcripts were quantified by RT-qPCR analysis in 3-wk-old Arabidopsis Col-0 plants grown in soil under GL (90 μmol·m−2·s−1) and HL (350 μmol·m−2·s−1) conditions. Samples were harvested after 3, 6, 12, 24, and 48 h of consistent light exposure. The GAPDH gene was employed as a reference gene. Data represent the means ± Sd, n = 3. **P < 0.01 (Student's t-test). C) The CYP37 gene was mainly expressed in Arabidopsis tissues containing chloroplasts. Spatial profiling was performed in the tissues of roots, stems, cauline leaves, rosette leaves, flowers, and siliques at the flowering stage by RT-qPCR analysis. Data represent the means ± Sd, n = 3. D) CYP37 protein localized in chloroplasts. Cauliflower mosaic virus 35S promoter (35S pro)-driven yellow fluorescence protein (YFP) was used as a negative control. YFP fused to the C-terminus of CYP37 proteins transiently expressed in Arabidopsis protoplasts (middle panel) and N. benthamiana epidermal cells (bottom panel). Fluorescent signals were observed by confocal microscopy. 35S pro::YFP, control with empty vector; 35S pro::CYP37-YFP, YFP fused to the C-terminus of CYP37. YFP, yellow fluorescent protein; Chlorophyll, chlorophyll autofluorescence; BF, bright-field image under transmitted light; Merge, merged image of YFP, chlorophyll, and bright field. Scale bars: 10 μm. E) CYP37 protein localized in the thylakoid lumen of chloroplasts. Immunoblotting was performed using samples from chloroplast-fractionated stroma (S), thylakoid (T), and lumen (L) proteins. FTR, ferredoxin‒thioredoxin reductase, a stroma protein marker; RFeS, Rieske iron–sulfur protein, a thylakoid membrane protein marker; PC, plastocyanin, a thylakoid lumen protein marker.
Previous studies reported that CYP37 proteins are present in chloroplast thylakoid lumens (Schubert et al. 2002). To assess and validate the spatial localization in plants, we measured the CYP37 transcripts in tissues from 40-d-old plants. The results showed that the CYP37 gene was transcribed in the tissues containing chloroplasts (Fig. 1C), indicating that it might localize in chloroplasts. To confirm this hypothesis, we fused a yellow fluorescent protein (YFP) at the carboxyl terminus of CYP37 protein (CYP37-YFP), which is driven by a cauliflower mosaic virus promoter (CaMV 35S). The fused protein was transiently expressed in Arabidopsis protoplasts, resulting in punctate fluorescent signals in protoplasts (Fig. 1D, middle panel). To further investigate CYP37 subcellular localization, chloroplast was localized using its natural autofluorescence. We observed that the fluorescent signals from the CYP37-YFP were randomly distributed in the chloroplasts (Fig. 1D, middle panel). In addition, we established the distribution of YFP in protoplasts as a negative control (Fig. 1D, upper panel). Furthermore, we confirmed the chloroplast localization of CYP37 proteins in the epidermal cells of Nicotiana benthamiana leaves (Fig. 1D, lower panel). These results implied that the CYP37-YFP-fused proteins may be imported into chloroplasts.
To assess endogenous CYP37 protein localization in chloroplasts, we sequentially isolated stroma, thylakoid membranes, and thylakoid lumens from Arabidopsis WT leaves. Furthermore, we employed anti-ferredoxin‒thioredoxin reductase, anti-Rieske iron–sulfur protein (RFeS), and anti-PC antibodies to confirm the purity of these 3 fractions (Fig. 1E). Using the anti-CYP37 antibody, we determined that the endogenous CYP37 protein was present only in the thylakoid lumen fractions (Fig. 1E). These results indicate that the CYP37 protein indeed localizes in the thylakoid lumen of chloroplasts. Combined with transcript profiling and localization assays, CYP37 is a functional protein in the thylakoid lumen of chloroplasts, probably in an HL-responsive manner.
The HL-inducible anthocyanin synthesis pathway is disrupted in the cyp37-1 mutant
To investigate the potential functions of CYP37 in response to HL exposure, we obtained a cyp37-1 transfer DNA (T-DNA) insertion mutant (CS1001179). Regarding the franking sequence tag, the T-DNA interrupted the ninth exon of the CYP37 gene, AT3G15520 (Fig. 2A). We examined the transcripts and proteins of CYP37 in the cyp37-1 mutant simultaneously. The reverse transcription polymerase chain reaction (RT-PCR) results suggested that the CYP37 transcripts were disrupted in the mutant (Fig. 2B). Additionally, CYP37 proteins were unable to be detected by the anti-CYP37 antibody in the cyp37-1 chloroplast extract (Fig. 2C). These results indicated that the cyp37-1 mutant is a knock-out allele.
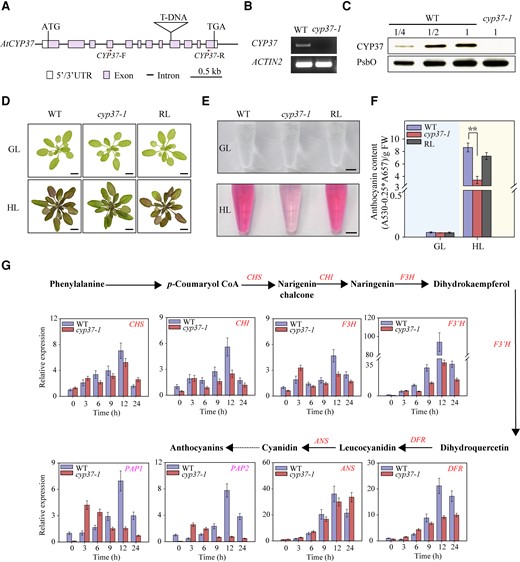
Decreased anthocyanin accumulation in cyp37-1 mutants under HL conditions. A) Schematic diagram of the CYP37 gene. Purple rectangles indicate exons, black lines indicate introns, and white rectangles indicate untranslated regions (UTRs). Triangles represent T-DNA insertion, and the ATG start codon and TGA stop codon are labeled. Red arrows indicate primers used in RT-PCR. CYP37-F and CYP37-R indicate forward primer and reverse primer used in cyp37-1 genotyping. B) The cyp37-1 mutant was identified by RT-PCR. CYP37 transcripts in the WT and cyp37-1 mutant were reverse transcribed and amplified by PCR. The ACTIN2 gene was used as a reference gene. C) Immunoblotting analysis of CYP37 proteins in WT and cyp37-1 mutant plants. Protein extracts were measured by chlorophyll amount, and the sample containing 3 μg chlorophyll was labeled 1 unit (U). U, 1/2 U, and 1/4 U of protein samples from WT, as well as 1 U protein samples from cyp37-1 mutant, were loaded. Photosystem II subunit O (PsbO) was used as a loading control. D) Anthocyanin accumulation was deficient in cyp37-1 mutant under HL conditions. WT, cyp37-1 mutant, and cyp37-1 RL plants were grown in soil under GL conditions (16 h light/8 h dark, 90 μmol·m−2·s−1) for 4 wk (upper panel) and then transferred to HL conditions (10 h light/14 h dark, 350 μmol·m−2·s−1) for 7 d (lower panel). The images were digitally extracted for comparison. Scale bars: 1 cm. E) Anthocyanin contents in WT, cyp37-1 mutants, and cyp37-1 RL plants under 350 μmol·m−2·s−1 light intensity. Scale bars: 0.5 cm. F) Concentration of anthocyanin in WT, cyp37-1 mutants, and cyp37-1 RL plants under 90 (left panel) and 350 (right panel) μmol·m−2·s−1 light intensity. Data represent the means ± Sd, n = 3. **P < 0.01 (Student's t-test). GL conditions and HL conditions were marked with light blue (left panel) and light yellow (right panel) backgrounds, respectively. G) The relative expression of anthocyanin biosynthesis genes in the WT and cyp37-1 mutant under HL (350 μmol·m−2·s−1) exposure. The glyceraldehyde-3-phosphate dehydrogenase (GAPDH) gene was used as a reference gene. The distinct anthocyanin biosynthetic pathway in Arabidopsis. Arrows indicate enzymatic reactions; red words indicate anthocyanin biosynthetic genes, and magenta words indicate positive regulators of anthocyanin synthesis. PAL, phenylalanine ammonia lyase; C4H, cinnamate 4-hydroxylase; 4CL, 4-coumaryl CoA ligase; CHS, chalcone synthase; CHI, chalcone isomerase; F3H, flavanone 3-hydroxylase; F3′H, flavonoid-3′-hydroxylase; DFR, dihydroflavonol reductase; ANS, anthocyanidin synthase; PAP1, production of anthocyanin pigment 1; PAP2, production of anthocyanin pigment 2. Data represent the means ± Sd, n = 3.
Considering the transcriptional manner of the CYP37 gene, we grew WT and cyp37-1 mutant plants under long-day conditions. When grown under GL, 4-wk-old seedlings did not show phenotypic differences between the WT and the mutant (Fig. 2D, upper panel). The seedlings were subsequently exposed to HL conditions for another week. The WT plants displayed intense pigmentation throughout the rosette leaves and petioles, which was subtle in the mutant plants (Fig. 2D, lower panel). For further investigation, we generated a cyp37-1 rescue line (RL) in the T-DNA insertion background (Supplemental Fig. S2) and obtained another T-DNA insertion mutant cyp37-2 (Supplemental Fig. S3). The RL showed phenotypic similarity to the WT plants under HL conditions (Fig. 2D).
Previous reports suggest that anthocyanin biosynthesis is induced by HL exposure in Arabidopsis (Vanderauwera et al. 2005). To confirm phenotypic differences under HL exposure, we isolated anthocyanins from 5-wk-old seedlings (Fig. 2E). Together with the anthocyanin extracts from the seedlings under GL, the anthocyanin concentration was quantified. The results showed that the anthocyanin concentration in the cyp37-1 mutant significantly decreased compared with that in the WT and RL under HL conditions (Fig. 2F). This result implies that HL-induced anthocyanin biosynthesis might be impaired due to a lack of CYP37.
To understand anthocyanin biosynthesis pathway changes in the cyp37-1 mutant, we performed transcriptional profiling of anthocyanin biosynthesis-related regulatory genes and structural genes using reverse transcription quantitative PCR (RT-qPCR). According to transcript quantification, the expression of the genes involved in catalyzing naringenin chalcone to cyanidin was decreased transcriptionally (Fig. 2G). To investigate potential regulators, we quantified the transcripts of anthocyanin biosynthesis-associated transcription factors, including production of anthocyanin pigment 1 (PAP1) and production of anthocyanin pigment 2 (PAP2) (Maier et al. 2013). Fewer PAP1 and PAP2 transcripts were observed in the cyp37-1 mutant, contrasting with the measurements in the WT plant. The mutant failed to maintain the expression of anthocyanin biosynthesis enzyme genes, probably by downregulating the transcriptional regulators, after sustained HL exposure (Fig. 2G).
CYP37 prevents the overaccumulation of ROS under HL conditions
To further investigate the role of CYP37 in responding to HL stress, we conducted HL (350 μmol·m−2·s−1) treatment on 3-wk-old WT and cyp37-1 seedlings. After 2 d of exposure, the leaves of cyp37-1 mutant plants showed pale green leaves. In addition, we measured the chlorophyll content from these plants. The results showed that the chlorophyll content declined significantly in the cyp37-1 mutant compared with that in the WT (Fig. 3, A and B). Furthermore, more severe chlorosis was observed when the light intensity increased to 750 μmol·m−2·s−1 in the cyp37-1 mutant (Fig. 3A, right panel). Previous studies have shown that the absorption of excess light leads to ROS accumulation, causing photooxidative stress and photosynthesis inhibition (Erickson et al. 2015). When exposed to HL, excessive ROS may cause subsequent bleaching of leaf color (Sato et al. 2015). To shed light on CYP37 functions in oxidative damage, we performed oxidative stress treatments on plants in GL (90 μmol·m−2·s−1) with the ROS-generating herbicide methyl viologen (MV), which is known to generate H2O2 and O2•− (Carmody et al. 2016). The presence of MV promotes the production of superoxide radicals in the chloroplast stroma by accepting electrons from PSI (Huang et al. 2018). Consistent with what was observed in the HL treatment, after 3 d of treatment, we found that cyp37-1 leaves were severely bleached after exposure to both 3 and 5 μM MV treatments compared with WT leaves (Fig. 3C). Moreover, decreased chlorophyll content was found in cyp37-1 compared with WT after MV treatment (Fig. 3D). These results indicated that the cyp37-1 mutant plants are hypersensitive to oxidative stress.
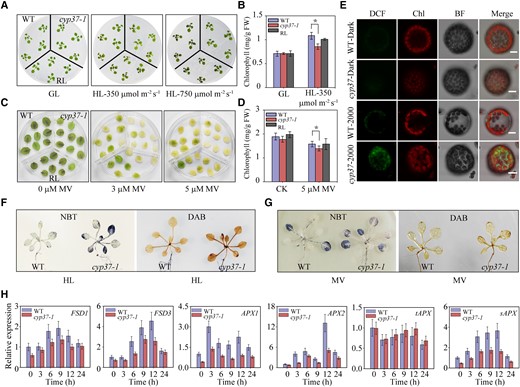
Lack of CYP37 leads to decreased chlorophyll content and increased ROS accumulation under HL and oxidative stress. A) Chlorophyll degradation in cyp37-1 mutant plants under HL stress. Wild type (WT), cyp37-1, and RL plants grew on 1/2 MS medium in growth chambers (16 h light/8 h dark, 90 μmol·m−2·s−1) for 2 wk, following GL conditions (16 h light/8 h dark, 90 μmol·m−2·s−1) (left panel), as well as HL stress (10 h light/14 h dark, 350 μmol·m−2·s−1) (middle panel) and HL stress (10 h light/14 h dark, 750 μmol·m−2·s−1) (right panel) for 2 d. B) Chlorophyll concentrations in the WT, cyp37-1, and RL plants under GL (90 μmol·m−2·s−1) and HL conditions (350 μmol·m−2·s−1) for 2 d. FW, fresh weight. Data represent the means ± Sd, n = 3. *P < 0.05 (Student's t-test). C) Detection of oxidative stress by MV treatment showed decreased chlorophyll caused by accumulated ROS in cyp37-1. Rosette leaves of 3-wk-old WT, cyp37-1, and RL seedlings supplemented with 0, 3, and 5 μM MV for 2 d. D) Total chlorophyll content of the recovered seedlings after prolonged exposure to 1/2 MS medium containing the indicated content of MV. WT, cyp37-1, and RL seedlings growing on ½ MS medium for 10 d were transferred to ½ MS medium containing the indicated concentration of MV for another 2 d in growth chambers (16 h light/8 h dark, 90 μmol·m−2·s−1). Data represent the means ± Sd, n = 3. *P < 0.05 (Student's t-test). E) Mutant cyp37-1 accumulated more ROS in cells under HL intensity. Intracellular ROS accumulation in WT and cyp37-1 mesophyll protoplasts under HL treatment for 15 min. Protoplasts extracted from WT and cyp37-1 plants were subsequently treated with or without HL (2000 μmol·m−2·s−1) and incubated with the ROS-sensitive fluorescent dye 2′, 7′-dichlorodihydrofluorescein diacetate (H2DCFDA) at a final concentration of 5 µM for 5 min at room temperature. Fluorescence signals were determined by confocal microscopy as described in the Materials and Methods. DCF (the oxidation product of H2DCFDA) fluorescence was falsely colored green. BF, bright-field image under transmitted light; Merge, merged image of DCF and bright field. Scale bars: 10 μm. F) ROS accumulation in cyp37-1 mutant plants under HL (750 μmol·m−2·s−1) stress. Two-wk-old WT and cyp37-1 mutant plants were subjected to HL treatment for 5 h. NBT staining was used to assess the superoxide anion. DAB staining was used to determine the hydrogen peroxide content. G) Mutant cyp37-1 accumulated more ROS in leaves under oxidative stress. Representative images of H2O2 accumulation as determined by NBT and DAB staining after MV treatment. H) The relative expression levels of the ROS marker genes FeSODs and APXs were measured by RT-qPCR in WT and cyp37-1 after HL (350 μmol·m−2·s−1) treatment. The results were normalized to GAPDH. FSD, Fe-dependent superoxide dismutases, catalyzing O2.− to H2O2; APX, ascorbate peroxidase, catalyzing the reduction of H2O2 into water. APX1&APX2, cytosolic APX; tAPX, thylakoid membrane-bound APX; sAPX, stromal APX. Data represent the means ± Sd, n = 3.
Previous reports showed that anthocyanin-deficient mutants are thought to accumulate more endogenous ROS. These mutants are sensitive to ROS-generating stresses by degrading more chlorophyll under HL conditions (Xu et al. 2017). Given the chlorosis in cyp37-1 plants caused by HL and oxidative stress, we investigated ROS accumulation in mesophyll protoplasts under HL. Mesophyll protoplasts were isolated from cyp37-1 and WT lines and exposed to 2000 μmol·m−2·s−1 HL for 15 min. Intracellular ROS were indicated by 2′, 7′-dichlorodyhydrofluorescein diacetate in the cyp37-1 protoplasts but were rarely observed in the WT protoplasts (Fig. 3E). In addition, to determine the ROS status, we performed nitroblue tetrazolium (NBT) staining to detect superoxide anions and diaminobenzidine tetrahydrochloride (DAB) staining to detect hydrogen peroxide. According to histochemical staining, the cyp37-1 mutant had a higher ROS level than the WT after 2 h of HL exposure (Fig. 3F). ROS levels were determined by NBT and DAB staining in MV-treated plants. Consistent with HL treatment, the cyp37-1 mutant accumulated more ROS than the WT plant under oxidative stress (Fig. 3G).
To understand the molecular mechanism of ROS overaccumulation in the cyp37-1 mutant, we analyzed the transcriptional levels of the enzymes in the antioxidant signaling pathway before and after HL treatment. The transcripts of cytosolic H2O2-scavenging enzymes ascorbate peroxidase 1 (APX1) and ascorbate peroxidase 2 (APX2) declined in the cyp37-1 mutant after HL treatment (Fig. 3H). Notably, the transcripts of chloroplastic H2O2 scavengers, stromal APX instead of thylakoid APX, were downregulated in the mutant under HL, as well as SOD isoenzymes FeSOD (Fig. 3H).
Lack of CYP37 impaired photosynthetic parameters under HL conditions
Under GL conditions, the electron transport between PSII and PSI could sustain balance, and electrons are passed from one carrier to another in an orderly manner. However, HL stress might create an energy imbalance and lead to the reduction or oxidation of the intersystem ETC. The imbalance between produced and consumed energy causes adverse reactions, producing ROS during photosynthesis (Calzadilla and Kirilovsky 2020). The cyp37-1 mutant displayed no visible phenotype upon growth under standard conditions but showed decreased anthocyanin and elevated ROS accumulation under HL (Figs. 2 and 3). This observation prompted us to examine the photosynthetic performance of this mutant during acclimation from GL (90 μmol·m−2·s−1) to HL conditions (HL, 350 μmol·m−2·s−1). To examine the responses of PSI and PSII to HL in cyp37-1 mutant plants, we subjected 4-wk-old WT and cyp37-1 mutant plants to GL and HL conditions for 4 d. The PSI and PSII parameters were measured simultaneously by a chlorophyll fluorometer Dual-PAM-100. There was no difference between WT and cyp37-1 in photosynthetic parameters under GL (Fig. 4A). However, the quantum yield of PSI photochemistry Y (I) of cyp37-1 was lower than that of WT at all times under HL (Fig. 4B). Decreased photochemically active PSI in Arabidopsis can affect a variety of photosynthetic parameters. Y (ND), a parameter that represents the quantum yield of nonphotochemical energy dissipation due to donor-side limitation and indicates the ratio of oxidized P700 to total photooxidizable P700, is an indicator of P700 oxidation and is used to monitor the DpH-dependent downregulation of the Cyt b6/f complex (photosynthetic control). Y (ND) of cyp37-1 is significantly higher than that of WT under HL conditions, which implies that the photochemical reaction in PSI of cyp37-1 is limited at the electron donor side and a higher P700 oxidation occurs in cyp37-1. The higher Y (ND) induced within 60 s of HL treatment in the cyp37-1 mutant plant indicated a more PSI donor-side limitation and less electron transportation to PSI. By comparison, ETR (I), the ETRs through PSI, significantly decreased in cyp37-1 under HL (Fig. 4B and Supplemental Fig. S4). Despite the significant decrease in PSI parameters, no parameters of PSII were drastically affected in cyp37-1 after HL treatment for 3 d. However, when we extended the HL treatment to 6 d, Y (II) and ETR (II) also decreased significantly (Supplemental Fig. S5). The decreased parameters caused by the absence of CYP37 can complement the levels of WT in RL.
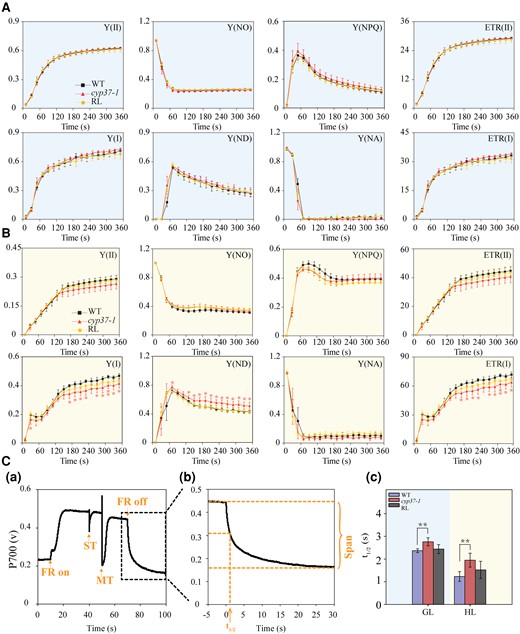
PSII and PSI parameters in the WT and cyp37-1 mutant under GL and HL conditions. A) The PSII and PSI parameters had a negligible difference in performance between the WT and cyp37-1 mutants under GL conditions. The induction of photosynthesis in WT, cyp37-1, and RL plants grown under GL conditions (90 μmol·m−2·s−1) was monitored by a chlorophyll fluorometer Dual-PAM-100 (Walz, Germany) after 30 min of dark adaptation. GL conditions are marked with a light blue background. Y (II), effective quantum yield of PSII; Y (NO), nonregulated energy dissipation in PSII; Y (NPQ), regulated energy dissipation in PSII; ETR (II), ETRs through PSII; Y (I), effective quantum yield of PSI; Y (ND), quantum yield of nonphotochemical energy dissipation of PSI due to donor-side limitation; Y (NA), quantum yield of nonphotochemical energy dissipation of PSI due to acceptor-side limitation; ETR (I), ETRs through PSI. Data represent the means ± Sd, n = 3. B) Y (I), Y (ND), and ETR (I) were significantly affected in cyp37-1 after HL treatment for 3 d. The induction of photosynthesis in WT, cyp37-1, and RL plants grown under HL (350 μmol·m−2·s−1) conditions was monitored by a chlorophyll fluorometer Dual-PAM-100 (Walz, Germany) after 30 min of dark adaptation. HL conditions were marked with a light yellow background. Data represent the means ± Sd, n = 3. *P < 0.05 (Student's t-test). C) The reduction in P700 was slower in cyp37-1 than in WT under both GL and HL conditions. a) Typical trace of measurements of the P700 redox state during ST flashes (50 ms, PQ pool oxidized) followed by MT flashes (50 ms, PQ pool is fully reduced) in the presence of FR background light. b) Enlarged display of the P700 signal after the FR light was removed. c) The half-life (t1/2) of dark decay kinetics to the steady state estimated from the P700 signal after FR light was removed. WT and cyp37-1 were grown under GL (90 μmol·m−2·s−1) and HL (350 μmol·m−2·s−1) conditions, respectively. The outline in C(a) indicates an enlarged display of the P700 signal in C(b). Data represent the means ± Sd, n = 7. **P < 0.01 (Student's t-test).
P700 is the reaction center chlorophyll pair acting as the primary electron donor of PSI. The redox state of P700 is a relative indicator of PSI activity. The transition of the P700 signal from the maximum oxidation state to the reduction state was analyzed after the FR light was turned off (Fig. 4, C, a and b). The maximum oxidation state of P700 rapidly decreased in WT, whereas P700+ was reduced slowly in cyp37-1 (Fig. 4, C, c). The P700 active center of cyp37-1 preferred to be maintained in its oxidized state, which may be due to the limitation of electron donation to PSI. In addition, the slower transition of the P700 signal from the maximum oxidation state to the reduction state in cyp37-1 also reflects a lower CET rate in cyp37-1. Therefore, we hypothesize that a lack of CYP37 destroys the redox balance of the PSI reaction center, thus causing more severe oxidative damage to PSI.
ROS overaccumulation resulting from the dysfunction of electron transport in the cyp37 mutant under HL stress conditions
To elucidate the role of CYP37 in photosynthesis electron transport, we investigated the ETR at PSI—in the presence of a specific inhibitor of photosynthetic electron transport 2,5-dibromo-3-methyl-6-isopropyl-p-benzoquinone (DBMIB), which prevents plastoquinone (PQ) oxidation by binding the Cyt b6/f complex and triggers superoxide accumulation (Petrillo et al. 2014). The ETR (I) represents the relative rate of electron transport through PSI during steady-state photosynthesis (Okegawa et al. 2007). When treated with the DBMIB, a lower ETR (I) and more ROS accumulation were observed in WT and cyp37-1 plants under GL conditions (Fig. 5, A and B). Furthermore, the ETR (I) in cyp37-1 plants after 4 d of HL exposure showed the same level as that in WT plants treated with 10 μM DBMIB (Fig. 5C). These results indicate that CYP37 is involved in electron transport from the Cyt b6/f complex to PSI. In addition, superoxide accumulated identically at a high level between 10 μM DBMIB-treated WT and HL-treated cyp37-1 plants (Fig. 5D). Previous studies reported that DBMIB prevents plastoquinols from reducing the Cyt b6/f complex and generates superoxide (Brunkard et al. 2015). In addition, in the time course experiments, we observed that ETR reduction and ROS induction occurred simultaneously (Supplemental Fig. S4). Taken together, we conclude that a possible dysfunction of the Cyt b6/f complex in electron transport exists in cyp37-1 mutant plants, which may cause ROS overaccumulation under HL conditions.
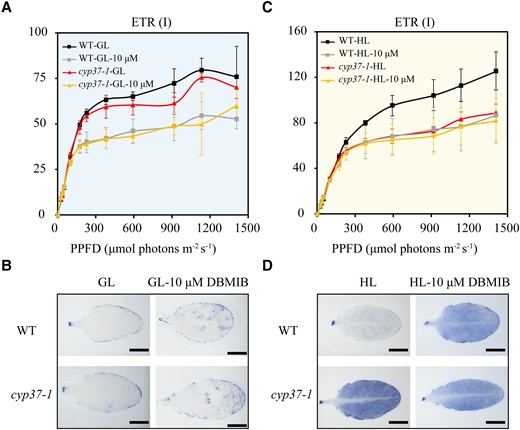
ETR (I) with or without DBMIB showed CYP37 potential functions in transferring electrons. A) No difference was observed in the ETR (I) of WT and cyp37-1 grown under GL conditions (90 μmol·m−2·s−1) with or without 10 μM DBMIB treatment. WT and cyp37-1 plants were grown under GL for 5 wk, then detached leaves were treated with mock or 10 μM DBMIB in the dark for 2 h and then used for ETR (I) determination. Data represent the means ± Sd, n = 3. PPFD, photosynthetic photon flux density. B) NBT staining showed that superoxide radical accumulation was the same as WT in cyp37-1 mutant plants under GL conditions with or without 10 μM DBMIB treatment. Scale bars: 0.5 cm. C)cyp37-1 mutant showed a similar level of ETR (I) as that observed in WT plants treated with 10 μM DBMIB under HL conditions (350 μmol·m−2·s−1). WT and cyp37-1 plants were grown in GL conditions for 5 wk and then exposed to HL conditions for 4 d. The leaves were treated with mock or 10 μM DBMIB in the dark for 2 h and then used for ETR determination. Data represent the means ± Sd, n = 3. D) The cyp37-1 mutant showed a similar level of ROS accumulation as that observed in WT plants treated with 10 μM DBMIB under HL stress conditions.
CYP37 physically interacts with subunit PetA of the Cyt b6/f complex
To investigate the role of CYP37 in photosynthesis, we performed blue-native gel electrophoresis followed by a 2D SDS‒PAGE immunoblot. After 30 min of exposure, we observed a blot using an anti-CYP37 antibody that was coupled with the PSII and Cyt b6/f complexes (Fig. 6A). The results imply that CYP37 may only weakly associate with these 2 complexes (Fig. 6A). To identify potential CYP37 interactors, we created a lumen protein library and conducted a screen using CYP37 protein as bait. Subsequently, we found a putative CYP37 interactor and characterized the cytochrome b6/f subunit PetA by Sanger sequencing. To determine whether they were interactors, we conducted a yeast two-hybrid assay using split-ubiquitin systems. The results showed that CYP37 might interact with PetA (Fig. 6B).
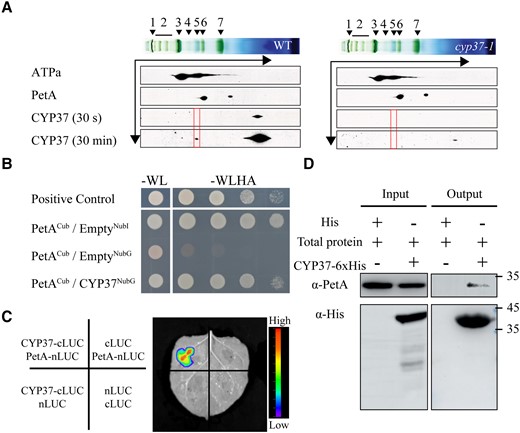
CYP37 interacts with PetA. A) BN/2D-SDS-PAGE immunoblots of thylakoid proteins showed that CYP37 interacted with thylakoid membrane complexes, as indicated by red frames. WT and cyp37-1 plants were grown in GL (90 μmol·m−2·s−1) conditions. Antibodies were applied as indicated: anti-ATPa (ATP synthase subunit a), anti-PetA (photosynthetic electron transfer A), and anti-CYP37.1, NDH (NADPH dehydrogenase complexes); 2, PSII SC (PSII supercomplexes); 3, PSI-M (PSI monomers), PSII-D (PSII dimers), PSII-M (PSII monomers) & LHC-T (LHC trimers); 4, PSI-M (PSI monomers), CF1 (a catalytic component of ATP synthase); 5, PSII-M (PSII monomers), Cyt b6/f (cytochrome b6/f complex); 6, LHCII assembly (light-harvesting complex II assembly); 7, LHCII-T (light-harvesting complex II trimers). The CYP37 protein was measured by western blotting using the anti-CYP37 antibody and exposed for 30 s at first and 30 min subsequently. Black arrows indicate the electrophoresis directions in first dimension BN-PAGE and second dimension SDS-PAGE. B) Yeast two-hybrid assay of the interactions between mature CYP37 and PetA proteins. A split-ubiquitin system was used to screen interactions. Yeast strain NMY32 was cotransformed with NubG-CYP37 prey and PetA-Cub bait and streaked on the permissive medium without Trp and Leu, as well as the selective medium without Trp, Leu, His, and Ade. NubG, N-terminal ubiquitin Gly; Cub, C-terminal ubiquitin. C) A split-luciferase complementary assay showed the interactions between CYP37 and PetA proteins. CYP37-cLUC (luciferase) was coexpressed with PetA-nLUC in N. benthamiana leaves. The luminescence signals were visualized 48 h after inoculation. The luminescence intensity was depicted by the color scale. D) CYP37, tagged by 6 histidine (His) residues, was expressed in E. coli. Total native proteins (input) from highlight exposed 5-wk-old plants were incubated with CYP37-His and immunoprecipitated with talon metal affinity resin (output). The input and output were visualized by anti-PetA and anti-His antibodies, followed by anti-rabbit and anti-mouse antibodies, respectively, after fractionation by SDS‒PAGE.
To confirm the interaction in vivo, we performed firefly luciferase complementation imaging assays in N. benthamiana leaves. Firefly luciferase (LUC) was split into N-terminal (nLUC) and C-terminal (cLUC) domains. cLUC was fused to the N-terminus of CYP37, and nLUC was fused to the C-terminus of PetA. Furthermore, as negative controls, we used nLUC and cLUC driven by 35S promoters. We set 4 cotransfections to test the interaction between CYP37 and PetA. Agrobacterium tumefaciens carrying T-DNAs that expressed nLUC and cLUC were infiltrated into the leaves. The luminescence signals were measured by a CCD camera and superimposed as heat-map photos (Fig. 6C, right panel). We detected reconstituted luminescence in the leaves co-expressing CYP37-cLUC and PetA-nLUC, while there were no luminescence signals in the leaves expressing control vectors (Fig. 6C), further supporting the interactions observed in the Y2H experiments.
In addition, to validate the interaction between CYP37 and PetA, we conducted coimmunoprecipitation (Co-IP) to enrich the CYP37 proteins tagged by histidine residues using talon metal affinity resin. Incubating it with the total native proteins, PetA proteins were immunoblotted by anti-PetA antibodies from the pulled proteins (Fig. 6D). Altogether, these results indicate that CYP37 directly interacts with PetA, a subunit of the Cyt b6/f complex, in vivo and in vitro.
CYP37 does not act as an assembly factor for the Cyt b6/f complex
To further investigate the role of CYP37 in the accumulation of the Cyt b6/f complex, we employed blue-native polyacrylamide gel electrophoresis (BN-PAGE) and 2D-SDS‒PAGE to analyze thylakoid samples from 3-wk-old WT and cyp37-1 plants (Fig. 7A). Surprisingly, the amount of components from PSII and PSI were at the same level in cyp37-1 mutant plants as in WT plants (Fig. 7A and Supplemental Fig. S6). To verify the CYP37 independent of the thylakoid protein complex assemblies, immunoblotting was employed to explore the stabilization of subunits from Cyt b6/f, PSI, PSII, and ATP synthase a in the WT and cyp37-1 plants (Fig. 7B), and no difference was observed in the accumulation of photosystem protein. This indicates that the lack of CYP37 did not impact the assembly and stabilization of the photosystem complexes. Combined with what we observed in Fig. 4, CYP37 might mediate the electron transport process of photosynthesis through the maintenance of Cyt b6/f under HL conditions.
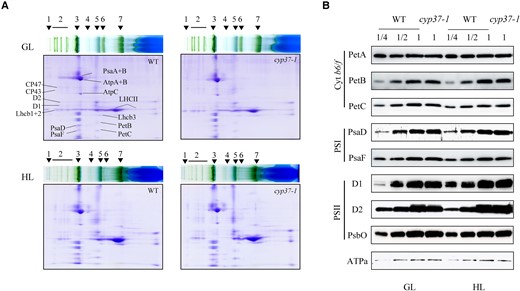
CYP37 did not affect the assembly and accumulation of thylakoid membrane proteins. A) Blue native/PAGE and subsequent analysis of thylakoid membrane complexes in 2D SDS/PAGE. Three-wk-old WT and cyp37-1 mutant plants grown in GL conditions (16 h light/8 h dark, 90 μmol·m−2·s−1) were transferred to HL conditions (10 h light/14 h dark, 350 μmol·m−2·s−1) and treated for 5 d. 1, NDH (NADPH dehydrogenase complexes); 2, PSII SC (PSII supercomplexes); 3, PSI-M (PSI monomers), PSII-D (PSII dimers), PSII-M (PSII monomers), and LHC-T (LHC trimers); 4, PSI-M (PSI monomers), CF1 (a catalytic component of ATP synthase); 5, PSII-M (PSII monomers), Cyt b6/f (cytochrome b6/f complex); 6, LHCII assembly; 7, LHCII-T. B) Five micrograms of proteins (based on chlorophyll quantification) from isolated chloroplasts were separated by 12% SDS‒PAGE and immunoblotted with anti-PetA (photosynthetic electron transfer A), anti-PetB (photosynthetic electron transfer B), anti-PetC (photosynthetic electron transfer C), anti-PsaD (photosystem I subunit D), anti-PsaF (photosystem I subunit F), anti-D1 (PSII reaction center protein A), anti-D2 (photosystem II reaction center protein B), anti-psbO (photosystem II subunit O), and anti-ATPa (ATP synthase subunit a) antibodies. The sample containing 5 μg chlorophyll was labeled 1 unit (U). U, 1/2 U, and 1/4 U of protein samples from WT, as well as 1 U protein samples from cyp37-1 mutant, were loaded. Three-wk-old WT and cyp37-1 mutant plants grown in GL (16 h light/8 h dark, 90 μmol·m−2·s−1) were transferred to HL conditions (10 h light/14 h dark, 350 μmol·m−2·s−1) and treated for 7 d.
Discussion
Although light is indispensable for photosynthesis, excessive light causes oxidative stress that decreases photosynthetic capacity, plant growth, and crop yield (Takahashi and Murata 2008; Kato et al. 2012; Simkin et al. 2017). Specifically, excessive light causes an imbalance between the production of electrons at the photosystems and the consumption of electrons at the chloroplast sinks, which results in the generation of ROS, which oxidize proteins, lipids, and metabolites (Powles 1984; Aro et al. 1993; Gururani et al. 2015; Bassi and Dall’Osto 2021). Therefore, it is important to investigate how plants adapt to photooxidative stress.
Here, we identified 1 relevant regulator in Arabidopsis, CYP37, which is a cyclophilin localized in the thylakoid lumen. Previous studies suggested that cyclophilins may be involved in adaptation to light or heat stresses, regulation of gene expression, and maintenance of chloroplast protein complexes (Lima et al. 2006; Fu et al. 2007; Bissoli et al. 2012; Bannikova et al. 2013). In our research, we found that CYP37 is highly responsive to HL treatment (Fig. 1B; Supplemental Fig. S1), which is also indicated by the abundance of light-responsive CREs existing in its promoter (Fig. 1A). This suggests that CYP37 is important for plants acclimating to HL conditions.
The anthocyanin biosynthesis pathway was disrupted in cyp37-1 mutant plants under HL stress
In response to stresses, plants accumulate anthocyanin to prevent cell damage; 1 role of anthocyanin is to decrease ROS by acting as an antioxidant (Grotewold 2006; Albert et al. 2009; Agati et al. 2012; Page et al. 2012). Previous studies found several transcription factors induced by HL that regulate anthocyanin biosynthesis (Vanderauwera et al. 2005; Lotkowska et al. 2015). Surprisingly, in the cyp37-1 knock-out mutant, anthocyanin accumulation after HL treatment was less than half of that in the WT plants (Fig. 2F).
To understand the mechanism, we quantified the expression of genes involved in anthocyanin biosynthesis and the regulators of those genes under HL treatment (Fig. 2G). In WT plants, gene expression peaked at 12 h of HL exposure. In cyp37-1 mutant plants, 2 regulators of anthocyanin biosynthesis, PAP1 and PAP2, spiked rapidly and peaked at 3 h of HL treatment (Fig. 2G), perhaps because of the higher ROS levels in cyp37-1 mutant plants. Previous work showed that PAP1 and PAP2 are induced by ROS stress in Arabidopsis (Tohge et al. 2005; Vanderauwera et al. 2005; Xu et al. 2017). Surprisingly, the expression levels of PAP1 and PAP2 declined steadily after 3 h and were much lower than those in WT plants (Fig. 2G). The decline in PAP1 and PAP2 expression may explain why anthocyanin accumulation was lower in cyp37-1 mutant plants (Fig. 2, D to F) at the end of the HL treatment.
One possible reason why PAP1 and PAP2 declined after 3 h is that the dramatically higher ROS levels in the cyp37-1 mutant (Fig. 3, E and F) may have impaired thylakoid function and disrupted the anthocyanin biosynthesis pathway. Previous work showed that excessive levels of intracellular ROS actually repress anthocyanin accumulation (Vandenabeele et al. 2004; Vanderauwera et al. 2005). These results suggest that CYP37 may be involved in reducing ROS accumulation and regulating the ETC in response to HL.
In response to oxidative stress, cyp37-1 mutant plants accumulated more ROS than WT plants
Under HL conditions, the cyp37-1 mutant displayed more severe chlorophyll degradation than the WT (Fig. 3, A and B). Chlorophyll degradation typically results from oxidative stress, which is induced by HL. The degradation of chlorophyll suggests that CYP37 may be involved in preventing ROS accumulation. To test this hypothesis, we exposed WT and cyp37-1 mutant plants to HL treatment and MV treatment, which induce oxidative stress by different means. Then, we measured ROS levels in several ways: chlorophyll degradation, NBT and DAB staining, and ROS-sensitive fluorescent dye (Fig. 3, A to G). The cyp37-1 mutant plants showed higher levels of ROS accumulation than the WT plants under both HL and MV treatments.
We also found that under HL conditions, several ROS scavenger genes (including SODs and APXs) had substantially lower expression levels in the cyp37-1 mutant plants than in the WT plants (Fig. 3H). Similar to anthocyanin biosynthesis, we believe that excessively high ROS levels may disrupt the biosynthesis pathway of scavenger genes by generally impairing thylakoid function.
The higher ROS accumulation in cyp37-1 mutant plants was caused by an imbalance in electron transport from PSII to PSI, which resulted from Cyt b6/f complex dysfunction
ROS are mainly produced in the chloroplasts due to the light-driven electron flow in the thylakoids (Dietz et al. 2016; Raja et al. 2017). Under HL conditions, ROS production is accelerated at PSI and PSII because of the imbalanced electron transport in the ETC. This causes electrons to accumulate at several sites, including the Cyt b6/f complex (Baniulis et al. 2013), the PQ pool (Khorobrykh et al. 2015), and the plastid terminal oxidase (PTOX) (Heyno et al. 2009), and thus oxygen is reduced to superoxide anion radicals. Specifically, isolated Cyt b6/f complexes have been shown to produce H2O2 when decylPQH2 and PC were used as an electron donor and electron acceptor, respectively (Sang et al. 2011).
ROS production during photosynthesis is inherent even under optimal conditions; thus, plants have evolved several mechanisms to cope with photodamage caused by ROS. For instance, the PSII repair cycle repairs damage to PSII caused by ROS (Theis and Schroda 2016; d’Alessandro and Havaux 2019). Additionally, the electron flux into and through the ETC adjusts to changing light intensity, particularly via the Cyt b6/f complex. In LET, electrons are transferred in sequence from PSII, through the cytochrome b6/f complex (Cyt b6/f), to PSI. CET includes PSI and Cyt b6/f, and CET transfers electrons from ferredoxin (Fd) to the PQ pool and generates an electrochemical proton gradient via the Q cycle of the Cyt b6/f complex across the thylakoid membrane (Shikanai 2007).
However, in the cyp37-1 mutant plants under HL conditions, the photosynthesis performance of PSII and PSI was impaired. These results indicate a lower effective quantum yield of PSII and PSI in cyp37-1 mutant plants than in WT plants (Fig. 4, A and B, Supplemental Fig. S3), probably due to ROS overaccumulation in the mutants (Fig. 3, E and F).
Several pieces of evidence suggest that the reason why ROS overaccumulate in cyp37-1 mutants under HL conditions is that the electron transfer at Cyt b6/f is interrupted.
First, we observed that in the cyp37-1 mutants, under HL conditions, PSI quickly showed reduced functioning, whereas PSII did not (Fig. 4B). This suggests that the ETR between PSII and PSI was impaired. Within 60 s of HL treatment, we observed a higher Y (ND) in the cyp37-1 mutant, which suggests a donor-side limitation of PSI (Fig. 4B). We also observed a longer half-life of P700 reduction in the mutant plants under HL conditions, which implies that fewer electrons were transported to PSI (Fig. 4C).
On the other hand, PSII performance in the first 120 s of HL treatment was similar between the cyp37-1 mutant and WT plants; PSII performance was measured by Y (II) and ETR (II) (Fig. 4B). This implies that electron production and transfer at PSII were not substantially affected in the cyp37-1 mutants in the first 120 s of HL stress. Taken together, the fact that PSI performance rapidly declined under HL stress but PSII performance did not suggests that in cyp37-1 mutants, there is a limitation in electron transfer between PSI and PSII.
Second, when electron transfer through Cyt b6/f was specifically inhibited in WT plants, they showed similar levels of ETR reduction and ROS overaccumulation under HL conditions as the cyp37-1 mutant did (Fig. 5C). This suggests that the electron transfer through Cyt b6/f may be inhibited in the cyp37-1 mutants, leading to ROS overaccumulation. Cyt b6/f is an important electron transporter between PSII and PSI, and plays important roles in both LET and CET. Defects in electron transfer through Cyt b6/f would result in less-efficient electron transfer to PSI as well as ROS accumulation. We interrupted electron transfer through Cyt b6/f in WT plants by applying 10 μM of DBMIB, a specific inhibitor of electron transport around Cyt b6/f (Petrillo et al. 2014). As expected, we observed lower ETR (I) and greater ROS accumulation under GL conditions (Fig. 5, A and B). Interestingly, cyp37-1 mutants (without DBMIB treatment) showed similar levels of ETR (I) and ROS accumulation as those observed in WT plants treated with DBMIB under HL conditions (Fig. 5C). This suggests that the electron transport through Cyt b6/f is dysfunctional in cyp37-1 mutant plants.
Third, we found that CYP37 interacts as an auxiliary factor with PetA, a subunit of the Cyt b6/f complex. This finding supports the hypothesis that CPY37 is involved in the functioning of the Cyt b6/f complex. Based on a BN-PAGE analysis, we found that the lack of CYP37 did not affect the assembly or expression of the Cyt b6/f complex or any thylakoid membrane protein (Fig. 7). These results led us to hypothesize that CYP37 interacts with the Cyt b6/f complex as an auxiliary factor. We confirmed this hypothesis with several results. CYP37 associated weakly with the Cyt b6/f complex under GL conditions (Fig. 6A) and specifically interacted with subunit PetA of the Cyt b6/f complex (Fig. 6, B, C, and D). The results indicate that CYP37 acts primarily on electron transfer through the Cyt b6/f complex.
Previous studies found that under HL stress, CET-deficient mutants showed reduced photosynthesis control, less NPQ, and more PSI photoinhibition (Okegawa et al. 2020). However, in cyp37-1 mutants, the CET does not appear to be impaired. Compared with the WT, cyp37-1 mutants showed higher Y (ND), which represents greater photosynthesis control, but showed lower Y (I) and ETR (I), which represents lower efficiency of PSI. This is different from what was observed in proton gradient regulation 5 (pgr5) mutants: photosynthesis control was diminished (lower Y (ND)) due to the deficit in PGR5-dependent CET, and lower NPQ and higher photodamage to PSI were observed under HL stress. This indicates that the defects in electron transport balance caused by the loss of CYP37 were not caused by CET-contributed photosynthesis control.
We also observed a lower relative rate of electron transport through PSII and PSI (ETR (II) and ETR (I)) in the cyp37-1 mutant (Fig. 4B, Supplemental Fig. S3). Since the Cyt b6/f complex lies between PSII and PSI in the ETC, this suggests that the activity of the Cyt b6/f complex is compromised.
Knowing that CYP37 is an auxiliary factor of the Cyt b6/f complex allows us to explain our previous results more clearly. The loss of CYP37 impaired the functioning of the Cyt b6/f complex under HL conditions, causing an imbalance in the electron flow from the Cyt b6/f complex to PSI through both LET and CET, resulting in higher Y (ND) and lower Y (I) in the cyp37-1 mutant within the first 60 s of HL treatment (Fig. 4B), which could finally cause more ROS accumulation in the cyp37-1 mutant (Fig. 5). Going with the auxiliary factor role of CYP37, a lower P700 reduction rate was also observed in cyp37-1 mutant plants even under GL conditions due to the important role of the Cyt b6/f complex in CET (Fig. 4C). Our results establish that CYP37 is an altruistic factor supporting the Cyt b6/f complex and is particularly important in HL conditions.
Conclusion
Light intensity and spectral quality are highly variable in nature. Excessive light or fluctuating light causes photodamage, which impairs plant growth and productivity. The regulation of photosynthetic electron transport around the photosynthetic machinery in the thylakoid membrane has been recognized as crucial to maintaining plant growth and photosynthesis under stress conditions. The results presented in this work indicated that CYP37, a thylakoid-localized cyclophilin, helps to regulate electron transport from PSII to PSI by coordinating with the Cyt b6/f complex, and it interacts specifically with the PetA subunit. The loss of CYP37 hindered the activity of the Cyt b6/f complex and reduced electron flow from the Cyt b6/f complex to PSI. This caused electron accumulation upstream of the Cyt b6/f complex, yielding more ROS between PSII and the Cyt b6/f complex (Fig. 8). The overaccumulation of ROS caused photodamage, chlorophyll degradation, and a reduction in anthocyanin biosynthesis (Fig. 8). Although the function of the Cyt b6/f complex has been studied for decades, the precise details of how it is regulated remain unknown. Our results illuminate how plants fine-tune the Cyt b6/f complex's activity to adapt the ETC to HL, and they suggest how crop yield under HL might be improved.

A proposed working model for CYP37-mediated photosynthetic electron transport under GL and HL conditions. A and B) Electron transport in WT and cyp37 mutant plants under GL conditions. C and D) Electron transport in WT and cyp37 mutant plants under HL conditions. GL conditions and HL conditions were marked with light blue (upper panel) and light yellow (lower panel) backgrounds, respectively. Solid red lines indicate the LET process. Dashed blue lines indicate the CET process. Distinct arrow line thickness indicates different electron flow efficiency. ROS, reactive oxygen species; PSII, photosystem II; PSI, photosystem I; Cyt b6/f, Cytochrome b6/f; OEC, oxygen-evolving complex; FNR, ferredoxin NADP+ oxidoreductase; Fd, ferredoxin; PC, plastocyanin; PQ, plastoquinone.
Materials and methods
Plant materials and growth conditions
The A. thaliana (ecotype Columbia-0) T-DNA insertion line cyp37-1 (stock CS1001179, locus AT3G15520) was obtained from the Arabidopsis Biological Resource Center (ABRC; http://abrc.osu.edu/). The T-DNA insertion site was confirmed by PCR using the primers listed in Supplemental Table S1. The transcriptional level of CYP37 was further confirmed by RT-PCR and immunoblotting. A mutant allele, cyp37-2 (SALK_142757C), was requested from Arashare (https://www.arashare.cn/index/). The T-DNA was inserted in the 3′untranslated region of the CYP37 gene, which is 19 bp downstream of the stop codon. The T-DNA insertion site was confirmed by PCR using the primers listed in Supplemental Table S1. The CYP37 transcripts were quantified by RT-qPCR using GAPDH as a normalization (Supplemental Fig. S3). Arabidopsis plants were grown in a greenhouse (22 °C, 16 h light/8 h dark, 90 μmol·m−2·s−1 light intensity, 60% humidity). To ensure germination, the seeds were stratified at 4 °C in the dark for 2 d and transferred to the greenhouse at 22 °C in the light (90 μmol·m−2·s−1). For HL stress treatment, plants were subjected to the indicated light intensity in a growth chamber with a temperature and light control system. We used 3 types of HL in our study. HL (350 μmol·m−2·s−1) was applied for long-term treatment. Elevated HL intensity (750 μmol·m−2·s−1) was used to stimulate rapid responses physiologically and molecularly. The 2,000 μmol·m−2·s−1 HL treatment was used to stress Arabidopsis protoplasts for 15 min, as protoplasts are breakable under long-term exposure to HL conditions. For plate-grown plants, seeds were surface-sterilized with 75% (v/v) ethanol for 3 min initially, followed by 50% (v/v) bleach for 5 min. Seeds were plated on 0.8% (g mL−1) agar plates with 1/2 MS medium after being washed 3 times with sterilized distilled water.
Promoter analysis
The reference sequence 2 kb upstream of Arabidopsis CYP37 was downloaded from the National Center for Biotechnology Information (NCBI) website (https://www.ncbi.nlm.nih.gov). The regulatory cis-elements were analyzed using PlantCARE (Lescot et al. 2002).
RNA isolation, reverse transcription, and RT-qPCR assays
Total RNA was isolated from frozen samples using TRIzol Reagent (Invitrogen, California, USA) according to the manufacturer's instructions. Two micrograms of total RNA were reverse transcribed with the Prime Script RT Reagent Kit (TaKaRa, Tokyo, Japan) using oligo-dT12-18 as a primer. All RT-qPCR assays were performed with a SYBR Premix Ex Taq kit (TaKaRa, Tokyo, Japan) on a 7300 plus real-time PCR system (Applied Biosystems) following the program described as follows: melting at 95 °C for 30 s and amplification with 40 cycles of 95 °C for 5 s and 60 °C for 34 s. Each assay consisted of 3 technical replicates with GAPDH as a reference gene in RT-qPCR. The primers used for RT-qPCR are listed in Supplemental Table S1.
Plasmid construction, plant transformation, and subcellular localization
The coding sequence of CYP37 without a stop codon was amplified with the primers listed in Supplemental Table S2 using Columbia-0 cDNA as a template. Amplicons were purified and subcloned into the plant transformation vector pEarleyGate 101 with YFP and hemagglutinin as C-terminal tags via gateway cloning (Supplemental Table S2). The genes were driven by the cauliflower mosaic virus 35S promoter. To examine the subcellular localization of CYP37, we observed the 35S::CYP37-YFP fusion protein using a confocal laser scanning microscope (Leica Microsystems, Germany) with 488 nm excitation wavelength with 30% intensity and 510 nm (800 V gain) emission wavelength in Arabidopsis protoplasts and N. benthamiana epidermal cells. Chlorophyll autofluorescence was visualized at 650 nm (600 V gain) with a bandpass filter.
For complementation of the cyp37-1 mutant, full-length fragments of CYP37 were amplified from genomic DNA and subcloned into the plant expression vector pCAMBIA1300 driven by the cauliflower mosaic virus 35S promoter and cut with 2 restriction enzymes BamHI and SmaI (Supplemental Table S2). The construct was introduced into A. tumefaciens strain GV3101 and transformed into cyp37-1 mutant plants by the floral dip method (Clough and Bent 1998).
All primers for plasmid construction are listed in Supplemental Table S2.
Chloroplast fractionation and immunoblotting analysis
Intact chloroplasts were isolated from approximately 15 g of fresh leaves according to previous descriptions (Kauss et al. 2012). Intact chloroplasts were fractionated into stroma, thylakoid membrane, and thylakoid lumen as described in the protocol (Shi et al. 2021). Protein samples collected from chloroplast fractionation were separated by SDS‒PAGE and transferred to nitrocellulose membranes for immunoblotting analysis.
Chlorophyll and anthocyanin content quantification
Chlorophyll contents (chlorophyll a and b) were isolated with 80% (v/v) acetone and quantified with a NanoDrop Spectrophotometer (Thermo Fisher Scientific) following the manufacturer's instructions. The total chlorophyll contents of individual plants were calculated as previously described (Porra et al. 1989).
For the anthocyanin measurement, 30 mg of plants frozen in liquid nitrogen was ground with a mortar and pestle, mixed vigorously with 1 mL of extraction buffer (1% [v/v] HCl, 18% [v/v] 1-propanol, and 81% [v/v] water), and incubated at 98 °C for 3 min. Samples were incubated at room temperature in darkness for 10 to 12 h and centrifuged at 12,000 × g at room temperature for 20 min. The anthocyanin quantity was measured by the absorption at 530 nm over the absorption at 657 nm. All data were normalized by the fresh weight used in each sample (Lotkowska et al. 2015).
Detection of ROS
To investigate intracellular ROS, we isolated Arabidopsis protoplasts from WT and cyp37-1 plants that were subsequently subjected to dark and HL conditions (2000 μmol·m−2·s−1) for 30 min and incubated with 5 μM H2DCFDA. Intracellular ROS was determined by monitoring the fluorescence of 2′, 7′-dichlorofluorescein (DCF), the product of oxidized H2DCFDA, as described previously (Wang et al. 2015). The intracellular ROS distribution, as well as the chloroplast autofluorescence, were visualized under a confocal laser scanning microscope (Leica Microsystems, Germany) with a 488 nm (30% intensity) excitation laser. The fluorescence of DCF was determined at 525 nm (800 V gain), and chloroplast autofluorescence was visualized at 650 nm (600 V gain) with a bandpass filter.
Histochemical staining of 2-wk-old plants for H2O2 and superoxide in GL or treated with HL (350 μmol·m−2·s−1) for 3 d was performed by incubation with either 0.1 mg mL−1 3,3-diaminobenzidine (DAB) in 50 mM Tris-acetate buffer, pH 5.0 (Sangon Biotech), or 0.1 mg mL−1 NBT (Sangon Biotech) in 10 mM potassium phosphate buffer (pH 7.8) for 24 h in darkness, as previously described (Hou et al. 2015; Jiang et al. 2016). The leaves were bleached using 95% (v/v) ethanol for 10 min at 80 °C to remove the chlorophyll.
In vivo measurement of chlorophyll fluorescence
Chlorophyll fluorescence and P700 absorption changes in the PSI reaction center were measured simultaneously using a portable chlorophyll fluorometer (Dual-PAM-100, Walz). For the monitoring of PSI and PSII parameters, plants were adapted in the dark for 30 min. A saturating pulse was subsequently applied to measure the maximum fluorescence and the maximum change in P700. After that, leaves were illuminated at saturating light of GL (90 μmol·m−2·s−1) or HL (350 μmol·m−2·s−1) for 6 min. The chlorophyll fluorescence parameters were calculated as follows: Y (II) = (Fm′−Fs)/Fm′; Y (NO) = Fs/Fm; Y (NPQ) = Fs/Fm′ − Fs'/Fm (Genty et al. 1989); Y (I) = 1 – Y (ND) – Y (NA); Y (ND) = P/Pm; Y (NA) = Pm−Pm′/Pm; Fs and Fm were the minimum and maximum fluorescence after dark adaptation, respectively; Fs′ and Fm′ represent the minimum and maximum fluorescence after light adaptation, respectively; and Fs was the light-adapted steady-state fluorescence monitored just before applying a saturation pulse. Y (II) represents the effective quantum yield of PSII photochemistry. The ETRs through PSII and PSI are directly related to Y (II) or Y (I), respectively. ETR (II) = Y (II) * PPFD * 0.5 * 0.84; ETR (I) = Y (I) * PPFD * 0.5 * 0.84. PPFD represents the irradiation level at 400−700 nm, and the constants represent the assumed average leaf absorptance of PPFD and the fraction of the light absorbed by a given photosystem.
Measurement of P700 redox kinetics
The redox state of P700 was evaluated as the light-induced absorbance change at approximately 820 nm using a Dual-PAM-100 fluorimeter (Walz, Germany). The P700 signal was measured during a single turnover (ST) flash (50 ls) followed by multiple turnover (MT) flashes (50 ms) in the presence of far-red (FR) background light (Yang et al. 2018). Application of ST (50 ls) and MT (50 ms) flashes of red saturating light causes transient reduction of P700+. Kinetic measurements of dark reduction of P700+ (t1/2) after turning off the FR light reflect the extent of CET around PSI (Wang et al. 2020).
Yeast two-hybrid analysis
Yeast two-hybrid assays were performed using the yeast (Saccharomyces cerevisiae) strain NMY32 supplied by Dualsystems Biotech, following the manufacturer's instructions with a minor modification (Peng et al. 2006; Bruckner et al. 2009). Briefly, the PetA coding sequence without the transit peptide was cloned into the Cub bait vector pCCW-STE, whereas the mature coding sequence of the CYP37 gene was cloned into the NubG prey vector pDSL-N (Supplemental Table S2). Interactions were determined by growing diploid yeast colonies on a selective medium (-WLHA, lacking Trp, Leu, His, and Ade). Plasmid PetA-NubG served as a negative control, whereas pAlg5-NubI and PetA-NubI were employed as the positive controls (NubG, N-terminal ubiquitin Gly; NubI, N-terminal ubiquitin Ile; Cub, C-terminal ubiquitin).
Firefly luciferase (LUC) complementation imaging assay
For Firefly LUC complementation imaging assays, the nucleotide sequence encoding the CYP37 protein was amplified and fused to the C-terminus of the pCAMBIA-cLUC vector, while the PetA gene was amplified and fused to the N-terminus of the pCAMBIA-nLUC vector (Supplemental Table S2). pCAMBIA-nLUC and pCAMBIA-cLUC were used as negative controls. Constructs were transformed into A. tumefaciens strain GV3101 using the freeze‒thaw method. Agrobacterium infiltration was carried out with syringes into 1-mo-old N. benthamiana leaves. After 2 d, the abaxial sides of N. benthamiana leaves were sprayed with 1 mM luciferin and kept in the dark for 5 min. An in vivo imaging system (Berthold LB 985) was used to capture the LUC signal at room temperature. Luminescence imaging of N. benthamiana leaves was captured. Data shown in Fig. 6C were based on 6 repetitions and 3 biological replicates.
Co-IP assay
CYP37 tagged by 6 histidine (His) residues was expressed in Escherichia coli cells. The cells were lysed in phosphate-buffered saline (PBS) buffer, sonicated, and centrifuged. The supernatant was incubated with the talon metal affinity resin (Clontech, 1103612A) with rotation at 4 °C for 2 h. The beads were washed 3 times with PBS buffer and ready for Co-IP. Total protein isolates using PBS buffer from the 4-d HL exposed WT plants were incubated with the beads on a rotation shaker at 4 °C for 2 h. The Co-IP products were washed 5 times with PBS buffer. Total proteins combined with CYP37-His and Co-IP samples were resuspended in Laemmli sample buffer and heated at 95 °C for 10 min. These samples were fractionated by 12% (w/v) SDS‒PAGE gel and immunoblotted by anti-His (Abclonal, AE003) and anti-PetA antibodies followed by anti-mouse (Biodragon, NMB1073154) and anti-rabbit antibodies (Biodragon, NMB1073155).
Separation of thylakoid membrane protein complexes by BN-PAGE
BN-PAGE was performed as previously described (Kügler et al. 1997) with a modification. For 2D-SDS‒PAGE, lanes of blue-native gel were excised with a razor blade and incubated in 2×SDS sample buffer containing 2.5% (v/v) β-mercaptoethanol for 15 min at 75 °C. BN-PAGE lanes were placed on top of a 12% SDS‒PAGE gel after incubation and subjected to a second dimension.
Immunoblotting analysis
Protein samples corresponding to equal amounts of chlorophyll were separated on 12% SDS‒PAGE gels and transferred to nitrocellulose membranes (BioTrace NT nitrocellulose, Mexico), followed by immunoblotting analysis. After blocking nonspecific binding with 5% milk, the blot was subsequently incubated with antibodies and detected using the Super Signal West Pico PLUS Chemiluminescent Substrate kit (Thermo Scientific, USA). Anti-PetA, PetB, PetC, PsaD, PsaF, D1, D2, PsbO, and ATPa antibodies were generated as described in our previous report (Hou et al. 2015), and anti-His antibody (Abclonal, AE003) was purchased from Abclonal (https://abclonal.com.cn/).
Statistical analyses
Unpaired Student's t-test (2-tailed) was performed for the statistical analyses.
Accession numbers
Sequence data from this article can be found in the GenBank data libraries under accession numbers AT3G15520 (CYP37), ATCG00540 (PetA), ATCG00720 (PetB), AT4G03280 (PetC), AT4G02770 (PsaD), AT1G31330 (PsaF), ATCG00020 (PsbA), ATCG00270 (PsbD), AT5G66570 (PsbO).
Acknowledgments
The authors would like to thank Mr Bhaskar Roberts for kindly providing advice on language editing.
Author contributions
X.Y., Y.C., S.L., and X.H. designed the research; X.Y., V.J.G., Y.Z., Z.S., L.Z., and X.H. performed the experiments; X.Y. and Y.C. wrote the paper; J.S. and X.H. revised the manuscript. S.L. and X.H. provided general supervision, and all authors read and approved the final manuscript.
Supplemental data
The following materials are available in the online version of this article.
Supplemental Figure S1. CYP37 protein levels under low light and high light conditions.
Supplemental Figure S2.CYP37 transcripts and CYP37 proteins in wild-type (WT), cyp37-1, and rescue line (RL) plants.
Supplemental Figure S3. Characterization and phenotypic analysis of cyp37-2.
Supplemental Figure S4. Electron transport rate through photosystem I (ETR (I)) and ROS accumulation in wild-type (WT) and cyp37-1 plants under high light conditions.
Supplemental Figure S5. PSII and PSI parameters in WT and cyp37-1 mutant after high light treatment for 6 d.
Supplemental Figure S6. CYP37 did not affect the assembly of thylakoid membrane protein complexes.
Supplemental Table S1. Primers used in this study.
Supplemental Table S2. Constructs in this study.
Funding
This work was supported by the National Key Research and Development Program of China (2021YFA0909600), Natural Science Foundation of Hubei Province (2022CFA025), and the Foundation of Hubei Hongshan Laboratory (2021hszd011).
References
Author notes
Xiaoxia Yang, Yufen Che and Veder J García contributed equally to this work.
The author responsible for distribution of materials integral to the findings presented in this article in accordance with the policy described in the Instructions for Authors (https://dbpia.nl.go.kr/plphys/pages/General-Instructions) is Xin Hou.
Conflict of interest statement. None declared.