-
PDF
- Split View
-
Views
-
Cite
Cite
Wenxiang Xie, Shuo Liu, Huiling Gao, Jun Wu, Dilin Liu, Toshinori Kinoshita, Chao-Feng Huang, PP2C.D phosphatase SAL1 positively regulates aluminum resistance via restriction of aluminum uptake in rice, Plant Physiology, Volume 192, Issue 2, June 2023, Pages 1498–1516, https://doi.org/10.1093/plphys/kiad122
- Share Icon Share
Abstract
Aluminum (Al) toxicity represents a primary constraint for crop production in acidic soils. Rice (Oryza sativa) is a highly Al-resistant species; however, the molecular mechanisms underlying its high Al resistance are still not fully understood. Here, we identified SAL1 (SENSITIVE TO ALUMINUM 1), which encodes a plasma membrane (PM)-localized PP2C.D phosphatase, as a crucial regulator of Al resistance using a forward genetic screen. SAL1 was found to interact with and inhibit the activity of PM H+-ATPases, and mutation of SAL1 increased PM H+-ATPase activity and Al uptake, causing hypersensitivity to internal Al toxicity. Furthermore, knockout of NRAT1 (NRAMP ALUMINUM TRANSPORTER 1) encoding an Al uptake transporter in a sal1 background rescued the Al-sensitive phenotype of sal1, revealing that coordination of Al accumulation in the cell, wall and symplasm is critical for Al resistance in rice. By contrast, we found that mutations of PP2C.D phosphatase-encoding genes in Arabidopsis (Arabidopsis thaliana) enhanced Al resistance, which was attributed to increased malate secretion. Our results reveal the importance of PP2C.D phosphatases in Al resistance and the different strategies used by rice and Arabidopsis to defend against Al toxicity.
Introduction
Aluminum (Al) is the most abundant metallic element in the earth's crust; nevertheless, Al toxicity issues only occur in acidic soils, which comprise up to 40% of the world's arable land (von Uexkull and Mutert 1995). In mildly acidic, neutral, or alkaline soil, most Al is present as nontoxic insoluble forms of Al, aluminum oxides, and aluminosilicates. However, in acidic soils with a pH value of 5.5 or below, a portion of Al can be solubilized into trivalent Al (Al3+), which is highly toxic to plant root growth. An overwhelming number of studies reveal that most phytotoxic Al3+ is bound to the root cell wall, which has a negative charge (Kochian 1995; Rengel and Reid 1997; Ma 2007). The tight binding of Al to the cell wall impairs the extensibility and function of the cell wall, inducing the inhibition of cell elongation (Tabuchi and Matsumoto 2001; Ma et al. 2004). A small portion of Al can enter the cell to bind or interact with symplastic targets to exert its toxic effects, which include disrupting the cytoskeleton and signal transduction (Grabski and Schindler 1995; Jones and Kochian 1995; Blancaflor et al. 1998; Jones et al. 1998). Al also targets DNA to induce DNA rigidity and fragmentation (Karlik et al. 1980; Karlik and Eichhorn 1989; Kopittke et al. 2016). Although the apoplastic component cell wall is the major Al-binding site, the relative importance of apoplastic versus symplastic toxicity of Al remains unresolved (Delhaize and Ryan 1995; Kochian 1995).
Plants have evolved various Al-resistance mechanisms to adapt to Al-toxic environments. A widespread and crucial Al-resistance mechanism involves plant roots secreting organic acids such as malate, citrate, and oxalate to chelate and detoxify Al (Ma et al. 2001; Ryan et al. 2001; Kochian et al. 2004; Liu et al. 2014). Different plant species may exude different organic acids to cope with Al toxicity. For example, wheat (Triticum aestivum) and oilseed rape (Brassica napus L.) secrete both malate and citrate (Delhaize et al. 1993; Ligaba et al. 2004, 2006; Ryan et al. 2009; Tovkach et al. 2013), while maize (Zea mays) and soybean (Glycine max) mainly secrete citrate for Al detoxification (Pellet et al. 1995; Yang et al. 2000). Buckwheat (Fagopyrum esculentum) and tomato (Lycopersicon esculentum) exude oxalate in response to Al stress (Ma et al. 1997; Zheng et al. 1998; Yang et al. 2011). In Arabidopsis (Arabidopsis thaliana), plant roots secrete mainly malate for Al detoxification, although a smaller amount of citrate is also secreted to defend against Al toxicity (Hoekenga et al. 2003; Liu et al. 2009). Anion transporters involved in malate and citrate secretion have been identified (Sasaki et al. 2004; Furukawa et al. 2007; Magalhaes et al. 2007) and belong to the Al-activated malate transporter and the multidrug and toxin extrusion families, respectively. AtALMT1 and AtMATE are responsible for the exudation of malate and citrate in Arabidopsis roots, respectively (Hoekenga et al. 2006; Liu et al. 2009).
The plasma membrane (PM) H+-ATPase generates a proton (H+) gradient across the PM that provides the driving force for the active transport of ions. Al stress was reported to activate or inhibit PM H+-ATPase activity depending on the plant species. For example, the PM H+-ATPase activity is activated by Al stress in soybean and Arabidopsis (Shen et al. 2005; Zhang et al. 2019a), whereas it is inhibited in barley (Hordeum vulgare) and summer squash (Cucurbita pepo) (Matsumoto 1988; Ahn et al. 2001). Al-induced activation of PM H+-ATPase is suggested to facilitate the secretion of organic acids in soybean and Arabidopsis (Shen et al. 2005; Zhang et al. 2019a).
Phosphorylation of the penultimate threonine residue within the C-terminal autoinhibitory domain of PM H++-ATPase and subsequent binding of 14-3-3 protein to the phosphorylated residue are required for the activation of PM H+-ATPase (Fuglsang et al. 1999; Svennelid et al. 1999; Wang et al. 2014). The phosphorylated penultimate threonine of PM H+-ATPase can be dephosphorylated by the D subfamily of type 2C protein phosphatases (PP2C.Ds) to inhibit PM H+-ATPase activity (Spartz et al. 2014; Ren et al. 2018; Akiyama et al. 2022). The Arabidopsis genome encodes 9 PP2C.D members that have been implicated in the regulation of various biological processes, including apical hook development, cell expansion, immune response, and leaf senescence (Spartz et al. 2014; Xiao et al. 2015; Couto et al. 2016; Ren et al. 2018). Nevertheless, whether PP2C.Ds are involved in Al resistance remains unknown.
Rice (Oryza sativa) is a highly Al-resistant species when compared with other crops and Arabidopsis. Rice secretes a small amount of citrate via OsFRDL4 (Oryza sativa FERRIC REDUCTASE DEFECTIVE 3-Like 4) to defend against Al stress; however, the organic acid secretion does not play a substantial role in Al resistance in rice when compared with other species (Ma et al. 2002; Yokosho et al. 2011). Several Al-resistance genes have been identified in both the monocot rice and dicot Arabidopsis. Functional analyses of these Al-resistance genes revealed that the 2 species have some common Al-resistance mechanisms. For example, the C2H2-type zinc-finger transcription factor STOP1/ART1 plays a crucial role in Al resistance by transcriptional regulation of a set of Al-resistance genes (Iuchi et al. 2007; Yamaji et al. 2009). STAR1 and STAR2/ALS3 interact with each other to form a functional bacterial-type ATP-binding cassette (ABC) transporter that is suggested to detoxify Al by transporting UDP-glucose to modify the cell wall or by sequestering Al in vacuoles (Larsen et al. 2005; Huang et al. 2009, 2010; Dong et al. 2017), although the underlying mechanism remains to be precisely determined. The half-size ABC transporter ALS1 localized to the tonoplast mediates the vacuolar sequestration of Al (Larsen et al. 2007; Huang et al. 2012). Rice also possesses different mechanisms to detoxify Al. Rice increases the accumulation of 4-coumaric acid and ferulic acid in response to Al stress to modify the cell wall and thereby reduce Al binding to the cell wall for defense against Al stress (Liu et al. 2020). The PM-localized NRAMP family member NRAT1 transports trivalent Al from the apoplasm to the cytoplasm to alleviate cell wall Al toxicity (Xia et al. 2010). In Arabidopsis, a similar NRAT1 homolog functioning in Al transport does not exist (Lu et al. 2018).
To further decipher Al-resistance mechanisms in rice, we have conducted a forward genetic screen to identify mutants with altered Al sensitivity using a highly efficient screening method (Liu et al. 2016). In this study, we identified and characterized an Al-hypersensitive mutant, sal1. Gene cloning led to the identification of SAL1 as a PP2C.D phosphatase. We demonstrated that SAL1 positively regulates Al resistance through dephosphorylating and inhibiting PM H+-ATPase. Furthermore, we found that mutation of NRAT1 in a sal1 background rescues increased Al sensitivity in sal1, and mutation of PP2C.D phosphatase-encoding genes in Arabidopsis increased Al resistance, suggesting that different strategies are adopted by rice and Arabidopsis to cope with Al toxicity.
Results
Identification of a rice mutant sal1 with hypersensitivity to Al
We previously carried out a forward genetic screen on an ethyl methanesulfonate (EMS)-mutagenized population and identified several mutants with altered Al sensitivity (Liu et al. 2016). In this study, we focused on one of these isolated mutants, called sal1 (SENSITIVE TO ALUMINUM 1). Under control conditions for 5 d, the sal1 roots grew slightly slower, albeit not statistically significantly slower, than the wild-type (WT) roots (Fig. 1A). However, the mutant roots grew remarkably slower than the WT roots under Al stress conditions (Fig. 1A), indicating that sal1 is hypersensitive to Al stress. As a control, we used CRISPR/Cas9 technology (Ma and Liu 2016) to generate a knockout mutant of an essential Al-resistance gene, ART1 (Yamaji et al. 2009), which has a 1-bp (A) insertion located 82 bp downstream of the start codon of ART1. sal1 showed similar Al hypersensitivity to that observed for art1 (Fig. 1A). To further characterize the Al-sensitive phenotype of sal1, we exposed WT, sal1, and art1 to Al for 24 and 48 h and then compared their Al tolerance during the first and second 24-h treatments. Surprisingly, unlike art1, sal1 did not display greater root growth inhibition in response to Al stress than WT during the first 24-h treatment (Fig. 1B). Nevertheless, during the second 24-h treatment, the root growth of sal1 was much more severely inhibited by Al stress than that of WT (Fig. 1B). These results imply that mutation of SAL1 impairs long-term Al tolerance.
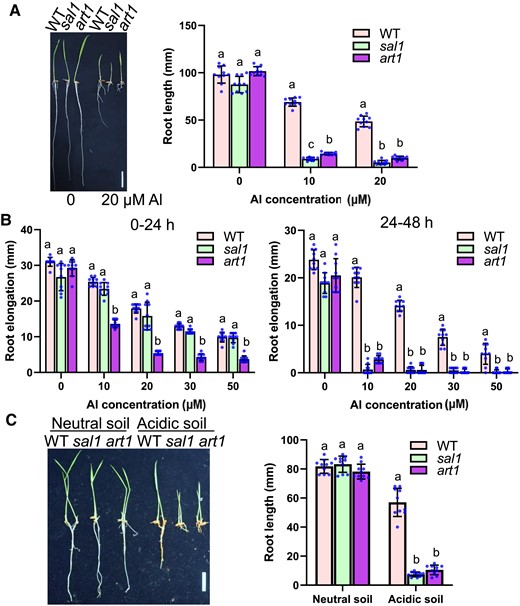
Mutation of SAL1 reduces Al resistance. A) Root growth of WT, sal1, and art1 in response to different Al concentrations. Germinated seeds were grown on a 0.5 mM CaCl2 solution containing 0, 10, or 20 µM Al at pH 4.5 for 5 d at 28 °C. Scale bar = 2 cm. B) Root elongation of 0 to 24 h (left panel) and 24 to 48 h (right panel) treatments of different Al concentrations. Five-day-old seedlings of WT, sal1, and art1 were exposed to a 0.5 mM CaCl2 solution containing 0, 10, 20, 30, or 50 µM Al at pH 4.5 for 48 h. Root elongation during the first and second 24-h treatments was measured and compared. C) Comparison of root growth of WT, sal1, and art1 on different soils. Germinated seeds were grown on neutral soil (pH 6.9) or acidic soil (pH 4.2) for 5 d. Concentrations of soluble Al in the neutral and acidic soils were 10.7 ± 1.2 and 129.2 ± 3.3 µM Al (n = 6), respectively. Scale bar = 2 cm. In all bar graphs of this figure, values are means ± SD (n = 10), and means with different letters are significantly different (P < 0.05, analysis of variance test followed by Tukey's test).
The root growth rate of sal1 was similar to that of WT and art1 when grown in neutral soil with a pH of 6.9 (Fig. 1C); however, in acidic soil with a pH of 4.2, the root growth of sal1 and art1 was similarly markedly more inhibited than that of WT (Fig. 1C), revealing the hypersensitivity of sal1 to the Al toxicity of acidic soils.
To investigate whether sal1 is specifically sensitive to Al toxicity, we tested the response of sal1 to other stresses, including low pH, cadmium (Cd), copper (Cu), and lanthanum (La). The mutant was slightly more sensitive to low pH than WT (Supplemental Fig. S1A). The mutant also showed increased sensitivity to Cu and La but not to Cd (Supplemental Fig. S1B). These results demonstrated that sal1 is sensitive to multiple stresses.
In addition, the sal1 mutant also displayed morphological alterations, including decreased plant height and panicle length, increased tiller number, and delayed flowering time (Supplemental Fig. S1C).
Mutation of SAL1 causes an increase in Al uptake
The effect of the sal1 mutation on Al accumulation was investigated by exposing WT and sal1 to 20 µM Al for 6 h, and then the roots were stained with an Al indicator Eriochrome Cyanine R. Compared to the WT, the mutant was stained more heavily in root tips (Fig. 2A), indicating that sal1 accumulated more Al than WT. Roots were then exposed to Al for 0.5, 3, and 6 h to determine differences in Al accumulation in compartments between WT and sal1. Root tip segments (0 to 1 cm) were fractionated into the cell wall and cell sap to measure the Al content in each compartment. Although the Al content in the cell wall was similar between the WT and mutant, the mutant accumulated more Al in the cell sap than WT at all time points (Fig. 2, B and C). Furthermore, we used morin, which detects cytosolic Al but not cell wall-bound Al (Eticha et al. 2005), to stain Al in WT and sal1. A comparison of morin and Al-dependent fluorescence revealed that sal1 accumulated more Al in the cytosol than WT (Fig. 2D). These results suggest that the sal1 mutation promoted Al uptake into the cell.
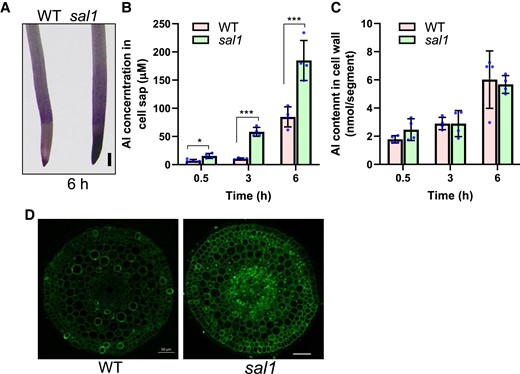
Increased Al accumulation in the sal1 mutant. A) Eriochrome Cyanine R staining of WT and sal1 mutant after 20 µM Al treatment for 6 h. Scale bar = 200 μm. B, C) Al concentration in cell sap (B) and Al content in cell wall (C) of WT and sal1 root tips. Five-day-old seedlings were exposed to a 0.5 mM CaCl2 solution (pH 4.5) containing 20 µM Al for 0.5, 3, or 6 h, and then root tips (0 to 1 cm) were harvested and fractionated into cell sap and cell wall for Al determination. Values are means ± SD of 4 biological replicates. Asterisks indicate values statistically different (Student's t test, *P < 0.05, ***P < 0.001). D) Morin staining of WT and sal1 after exposure to 20 µM Al for 6 h. Roots were transversely sectioned around 3 mm from apexes for the morin staining and fluorescence observation. Scale bar = 50 μm.
SAL1 encodes a PP2C.D phosphatase
Genetic analysis of sal1 was conducted using an F2 population generated from a cross between sal1 and the WT control. Among 481 F2 seedlings, 118 plants showed hypersensitivity to Al stress (root elongation < 5 mm), while the other 363 plants displayed similar Al tolerance to WT (root elongation > 7 mm) (Supplemental Fig. S2A). The ratio of the number of Al-sensitive seedlings to the number of Al-tolerant seedlings fitted to 1:3 (χ2 = 0.034, P > 0.9), suggesting that a single recessive gene controlled the hypersensitivity of sal1 to Al.
To clone the sal1 mutated gene, we used the same F2 population and pooled DNA from 92 seedlings with increased sensitivity to Al for whole-genome sequencing. The WT DNA was also sequenced and used as a control. We located sal1 to a small region of the long arm of chromosome 6 by MutMap analysis (Abe et al. 2012) (Supplemental Fig. S2B). The mapping-by-sequencing results were confirmed by developing 4 derived cleaved amplified polymorphic sequence (dCAPS) markers based on the mutations in sal1 in the candidate region (Supplemental Table S1), and the 92 Al-sensitive F2 seedlings were used to conduct a linkage analysis. All 4 markers showed linkage to the mutant phenotype to different extents (Supplemental Table S1), confirming that the sal1 mutated gene was located in this region. Among the 4 markers, only marker WX4 developed from a C-to-T substitution at +1625 bp from the start codon of LOC_Os06g50380 was completely linked to the mutant phenotype (Supplemental Table S1). This substitution caused an amino acid alteration from alanine to valine (Fig. 3A). These results suggest that the mutation in LOC_Os06g50380 is responsible for the increased sensitivity of sal1 to Al.
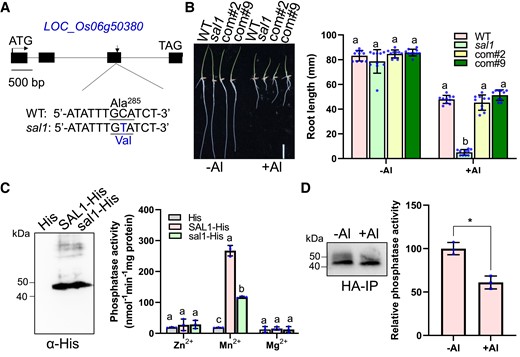
Complementation test of sal1 and the effect of sal1 mutation and Al stress on SAL1 phosphatase activity. A) Gene structure and mutation site of SAL1 (LOC_Os06g50380). Black boxes and horizontal lines between the boxes indicate exons and introns, respectively. sal1 has a C-to-T substitution at +1625 bp from the start codon of SAL1, causing an amino acid change from alanine to valine. B) The increased Al sensitivity of sal1 is rescued in 2 complementation lines. Germinated seeds of WT, sal1, and 2 complementation lines (com#2 and com#9) were grown on a 0.5 mM CaCl2 solution containing 0 or 20 µM Al at pH 4.5 for 5 d, and then the root length was measured and compared. Scale bar = 2 cm. C) Phosphatase activity assay of SAL1-His and sal1-His. The SAL1-His and sal1-His recombinant proteins purified from E. coli were detected by an immunoblot analysis using anti-His antibody (left panel) and then subjected for the phosphatase activity assay using pNPP as the substrate in the presence of 10 mM ZnCl2, MnCl2, or MgCl2 (right panel). D) Al stress reduces the SAL1 phosphatase activity. Five-day-old seedlings of a sal1 complementation line was exposed to 0 (−Al) or 20 μM Al (+Al) for 6 h, and root tips were sampled for SAL1-HA immunoprecipitation. The SAL1-HA immunoprecipitates detected by anti-HA antibody (left panel) were subjected for the phosphatase activity assay (right panel) using pNPP as the substrate in the presence of 10 mM MnCl2. Values are means ± SD of 10 (B) or 3 (C and D) biological replicates. Means with different letters are significantly different (P < 0.05, analysis of variance test followed by Tukey's test). Asterisks indicate values statistically different (Student's t test, **P < 0.01).
To confirm that LOC_Os06g50380 is SAL1, we performed a complementation test by transforming a WT version of LOC_Os06g50380 harboring a 3.5 kb promoter and the gene sequence fuzed with the 3 x hemagglutinin (HA) tag into the sal1 mutant. Measurement of root elongation in response to Al stress showed that the Al-hypersensitive phenotype of sal1 was rescued in 2 complementation lines (Fig. 3B), confirming that SAL1 is LOC_Os06g50380, whose mutation causes the Al hypersensitivity.
SAL1 contains 4 exons and 3 introns and encodes a protein of 392 amino acids in length. BLAST searches revealed that SAL1 encodes a D-clade member of the PP2C phosphatase family. Rice and Arabidopsis genomes have 10 and 9 PP2C.D phosphatases, respectively, and SAL1 is closely related to Arabidopsis AtPP2C.D7 (Supplemental Fig. S3A). The previous research identified 8 motifs in the PP2C.D subfamily, although the function of each motif remains unknown (Xue et al. 2008). The sal1 mutation with the alanine-to-valine substitution occurred in the seventh motif, which is fully conserved in the PP2C.D family (Supplemental Fig. S3B) (Xue et al. 2008). PP2C family proteins require Mn2+ or Mg2+ to exert their phosphatase function (Shi 2009; Fuchs et al. 2013). To examine whether SAL1 can function as a phosphatase, we purified both WT and mutant SAL1 proteins from Escherichia coli (Fig. 3C) and then tested their phosphatase activity in the presence of Mn2+, Mg2+, or Zn2+ by using para-nitrophenylphosphate (pNPP) as the substrate. SAL1 displayed phosphatase activity in the presence of Mn2+ but not in the presence of Mg2+ or Zn2+ (Fig. 3C), suggesting that SAL1 is a Mn2+-dependent phosphatase. Phosphatase activity of the sal1 mutant protein was observed but significantly reduced when compared with WT SAL1 activity (Fig. 3C), suggesting that the seventh motif is related to the phosphatase activity of the PP2C family and that sal1 is a dysfunctional allele but not a knockout allele. We attempted to generate SAL1 knockout mutants using CRISPR/Cas9 technology but failed, indicating that SAL1 may be essential for the rice plant growth.
To investigate whether Al stress can affect SAL1 phosphatase activity, we exposed a complementation line of sal1 to 0 or 20 μM Al for 6 h and then sampled the root tips for SAL1-3HA immunoprecipitation. The analysis of the phosphatase activity of SAL1-3HA immunoprecipitates using pNPP as the substrate revealed that Al stress inhibited SAL1-3HA phosphatase activity (Fig. 3D).
Expression pattern of SAL1
SAL1 was expressed in all tissues examined, including roots, leaf blades and sheathes, stems, and panicles (Fig. 4A). Time-course expression analysis showed that SAL1 expression in roots was induced by Al stress and reached a maximum after Al treatment for 6 h (Fig. 4B). In concert with the Al-induced expression of SAL1, the SAL1 protein level was increased slightly under Al stress as revealed by immunoblot analysis of SAL1-3HA in a complementation line of sal1 (Fig. 4C).

Expression pattern of SAL1. A) Expression analysis of SAL1 in roots, stems, leaf blades, leaf sheaths, and panicles. B) Response of SAL1 expression to Al toxicity at different time points. Five-day-old WT seedlings grown on a 0.5 mM CaCl2 solution (pH 4.5) were exposed to the same solution containing 20 μM Al for 0, 3, 6, 12, or 24 h, and then root tips (0 to 1 cm) were sampled for the expression analysis. C) Representative pictures (left panel) and relative protein level (right panel) of SAL1-3HA under −Al or +Al conditions. Seedlings of a sal1 complementation line was exposed to 0 (−Al) or 20 μM Al (+Al) for 6 h, and root tips were sampled for immunoblot analysis of SAL1-3HA and actin control. SAL1-3HA protein levels were first normalized to their respective Actin controls and then normalized to the protein level under the −Al condition. Data shown are means ± SD of three biological replicates. Asterisk indicates values statistically different (Student's t test, *P < 0.05). D–H) Tissue-specific GUS expression of pSAL1:SAL1-GUS transgenic lines. GUS staining in roots (D), root tip region (transverse section) (E), mature root region (transverse section) (F), leaves (G), and stems (transverse section) (H). Scale bar = 200 μm in (D, F, and G), 50 μm in (E), and 2 mm in (H).
To examine the tissue-specific expression pattern of SAL1, we constructed a translational fusion between SAL1 and GUS under the control of the SAL1 promoter (pSAL1:SAL1-GUS) and then transformed the fusion gene into rice plants. GUS staining in a pSAL1:SAL1-GUS transgenic line showed that GUS was expressed in roots, leaves, and stems (Fig. 4, D to H). In roots, GUS expression was detected in all cells of the root tip region, whereas in the mature region, the GUS signal was preferentially observed in outer cell layers and central tissues (Fig. 4, E and F). In the leaves and stems, GUS expression was highly detected in vascular tissues (Fig. 4, G and H).
SAL1 localizes to the PM
The subcellular localization of SAL1 was examined by constructing 35S:SAL1-GFP (GFP fuzed to the C-terminus of SAL1) and then introducing the construct into sal1 mutant plants. The expression level of SAL1/sal1 in the transgenic lines was 1.8- to 5.8-fold greater than that observed in the mutant (Supplemental Fig. S4A). Unfortunately, we were unable to detect the SAL1-GFP signal in the transgenic lines. Nevertheless, the 35S:SAL1-GFP transgene rescued the Al-hypersensitive phenotype of sal1 (Supplemental Fig. S4B), indicating that SAL1-GFP is functional in vivo. We then introduced the 35S:SAL1-GFP fusion gene into rice protoplasts to determine the subcellular localization of SAL1. SAL1-GFP localized to the PM (Fig. 5), which contrasts with the localization of the GFP control in the cytoplasm. We also analyzed the subcellular localization of the sal1 mutant protein and showed that sal1-GFP also localized to the PM (Fig. 5), indicating that the sal1 mutation did not affect its subcellular localization. Domain searches revealed that SAL1 did not have a transmembrane domain but contained 3 potential palmitoylation sites (Cys12, Cys151 and Cys152) for membrane anchoring. Mutation of Cys12 to Gly12 did not affect the subcellular localization of SAL1-GFP, whereas mutations of C151 and C152 partially disrupted the PM localization of SAL1-GFP (Fig. 5). However, when all 3 sites were mutated, SAL1-GFP localized in the cytoplasm (Fig. 5). These results suggest that the 3 sites, Cys12, Cys151, and Cys152, may be palmitoylated and involved in anchoring SAL1 to the PM.
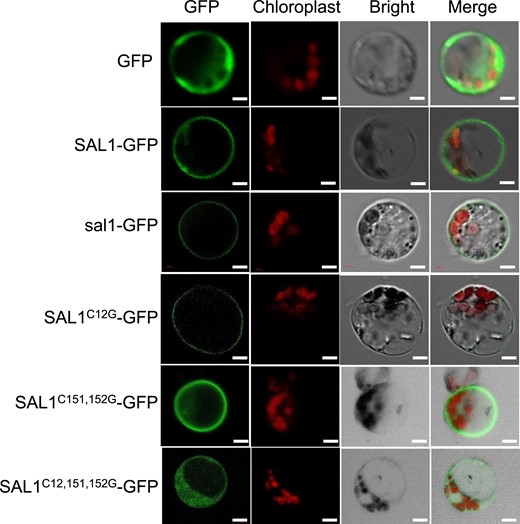
SAL1 is localized to the plasma membrane. Subcellular localization of WT or mutated SAL1-GFP under the CaMV 35S promoter control. Each construct indicated in the figure was transformed into rice protoplasts for GFP and chlorophyll fluorescence observation. Single, double, or triple Cys-to-Gly mutations of three potential sites Cys12, Cys151, and Cys152 were generated to examine their effects on the SAL1 subcellular localization. Scale bar = 5 μm.
SAL1 interacts with and inhibits PM H+-ATPase
The split-ubiquitin yeast 2-hybrid system (Johnsson and Varshavsky 1994) was used to search for proteins interacting with SAL1. We identified 261 SAL1-interacting clones covering 141 proteins through this screening (Supplemental Table S2). We selected OSA7, a PM H+-ATPase, as an important target of SAL1 because PM H+-ATPase has been documented to be negatively regulated by PP2C.D members in A. thaliana (Spartz et al. 2014; Ren et al. 2018). We confirmed that SAL1 interacted with OSA7 using the split-ubiquitin yeast 2-hybrid assay (Fig. 6A). In addition to OSA7, SAL1 interacted with other PM H+-ATPases, including OSA6 and OSA8 to OSA10 (Fig. 6A). An analysis of previous RNA-seq data (Liu et al. 2020) revealed that the transcript of OSA7 in roots was much more abundant than that of other PM H+-ATPase-encoding genes (Supplemental Fig. S5A). Thus, we selected OSA7 as a representative to further test the interaction between SAL1 and PM H+-ATPases. A split luciferase (LUC) complementation assay showed that the LUC signal was detected only with the SAL1-cLUC and OSA7-nLUC combination (Fig. 6B), reflecting the in planta interaction between SAL1 and OSA7. A co-immunoprecipitation (co-IP) assay revealed that the SAL1-3HA fusion protein co-immunoprecipitated with OSA7-2Flag (Fig. 6C) but not with a PM-localized negative control Rac1-2Flag (Kawano et al. 2014). Therefore, SAL1 interacts directly with PM H+-ATPase OSA7.
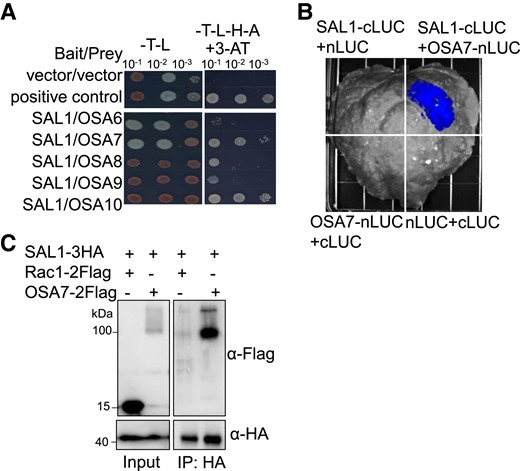
SAL1 interacts with PM H+-ATPase OSA7. A) Interaction of SAL1 with rice PM H+-ATPases in a yeast 2-hybrid assay. The CDS of SAL1 and OSA6 to OSA10 were constructed into pBT3-STE and pPR3-N vectors, respectively. Yeast cells co-expressing SAL1 and OSA6 to OSA10 were grown on SD (-Leu/Trp) and SD (-Leu/Trp/His/Ade) containing 2 mM 3-AT media for 3 d. B) Interaction of SAL1 with OSA7 in a split LUC complementation assay. CaMV 35S promoter-driven construct pairs indicated in the figure were co-expressed in N. benthamiana leaves, and then the LUC activity due to LUC reconstitution was measured for the different combinations. C) Co-IP of SAL1 with OSA7. SAL1-3HA was co-expressed with OSA7-2Flag or Rac1-2Flag in rice protoplasts. Crude protein extracts were immunoprecipitated with anti-HA magnetic beads and then detected with anti-Flag antibody.
We co-expressed SAL1 with PM H+-ATPase-encoding genes in yeast strain RS-72 to investigate whether SAL1 can regulate PM H+-ATPase activity. The RS-72 yeast cells can survive on galactose but not glucose media because the endogenous yeast H+-pump PMA1 is under the control of the galactose-induced GAL1 promoter (Fuglsang et al. 1999, 2007). Expression of OSA7, OSA8, or OSA9 enabled the yeast cells to grow on the glucose medium (Fig. 7A). However, when we co-expressed SAL1 with these H+-ATPase-encoding genes, the yeast cells were again unable to grow on the glucose medium (Fig. 7A), demonstrating that SAL1 negatively regulates PM H+-ATPase function.
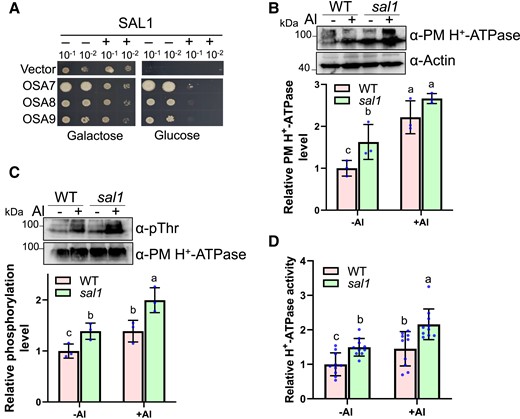
SAL1 inhibits PM H+-ATPase activity. A) Expression of SAL1 in yeast abolishes the complementation of PM H+-ATPase activity by OSA7, OSA8, or OSA9. B-D, sal1 mutation, and Al stress increase PM H+-ATPase abundance (B), phosphorylation level (C), and activity (D). Five-day-old seedlings were exposed to 0 (−Al) or 20 μM Al (+Al) at pH 4.5 for 6 h, and then root tips (0 to 1 cm) were sampled for immunoblot analysis and ATPase activity assay. B) Total protein was isolated for the immunoblot analysis of PM H+-ATPase using the native anti-PM H+-ATPase antibody. Actin control was used for the normalization of the PM H+-ATPase level. Values are means ± SD of three biological replicates. C) Plasma membrane fraction was isolated for the immunoblot analysis. Phosphorylated PM H+-ATPase was detected using anti-pThr antibody recognizing the penultimate threonine of PM H+-ATPase, and the PM H+-ATPase was used as the loading control and normalization. Values are means ± SD of three biological replicates. D) Relative vanadate-sensitive ATP hydrolytic activity in plasma membrane fractions. Values are means ± SD of 9 biological replicates. In all bar graphs of this figure, means with different letters are significantly different (P < 0.05, analysis of variance test followed by Tukey's test).
Next, we examined the effect of Al stress and the sal1 mutation on PM H+-ATPase levels in rice plants. An immunoblot analysis using a previously generated polyclonal antibody recognizing PM H+-ATPase (Hayashi et al. 2010; Toda et al. 2016) showed that Al stress promoted PM H+-ATPase accumulation in root tips (0 to 1 cm; Fig. 7B), which may be partially attributed to Al-induced expression of OSA7 (Supplemental Fig. S5B). The sal1 mutation increased the PM H+-ATPase abundance and the OSA7 transcript levels significantly when compared with those of the WT control under −Al conditions (Fig. 7B; Supplemental Fig. S5B). In the presence of Al, the PM H+-ATPase abundance in sal1 was not significantly different from that in WT although the expression level of OSA7 was higher in the mutant than in the WT (Fig. 7B; Supplemental Fig. S5B). Phosphorylation of the plant conserved penultimate threonine of PM H+-ATPase is associated with its activity (Fuglsang et al. 1999; Svennelid et al. 1999; Wang et al. 2014), and Arabidopsis PP2C.D1 negatively regulates H+-ATPase AHA2 by dephosphorylating the penultimate threonine Thr-947 of AHA2 (Spartz et al. 2014). Thus, we compared the phosphorylation level of the penultimate threonine of PM H+-ATPase in the root tips of WT and the sal1 mutant under −Al or +Al conditions by using the anti-pThr antibody that recognizes the penultimate threonine of rice PM H+-ATPase (Hayashi et al. 2010; Toda et al. 2016). Al stress caused an increase in the phosphorylation level of PM H+-ATPase (Fig. 7C), and the mutant showed a higher PM H+-ATPase phosphorylation level than the WT control (Fig. 7C), suggesting that SAL1 dephosphorylates the penultimate threonine of PM H+-ATPase. We then determined PM H+-ATPase activity in the root tips of WT and the mutant under different Al conditions. Al stress and the sal1 mutation increased PM H+-ATPase activity (Fig. 7D). Together, these results demonstrated that SAL1 interacts with and dephosphorylates PM H+-ATPase to negatively regulate the PM H+-ATPase function.
Increased PM H+-ATPase activity causes the hypersensitivity of sal1 to Al
To determine whether the elevated PM H+-ATPase activity caused the Al-hypersensitive phenotype of sal1, we exposed WT and sal1 roots to Al and/or the H+-ATPase inhibitor vanadate for 24 and 48 h. There was no difference in Al and/or vanadate-induced inhibition of root elongation between WT and sal1 during the first 24 h treatment (Fig. 8A). However, during the second 24 h treatment, the increased sensitivity of sal1 to Al was rescued largely by adding vanadate (Fig. 8A), implying that the Al-sensitive phenotype of sal1 was induced by the increase in H+-ATPase activity. We also exposed WT and mutant roots to Al and/or the H+-ATPase activator fusicoccin (FC) for 24 h. The mutant showed higher sensitivity to FC than the WT (Fig. 8B). Although the supply of FC alone did not affect WT root elongation, the supply of both FC and Al inhibited WT root elongation more than the supply of Al alone (Fig. 8B). FC treatment also increased the sensitivity of the mutant to Al compared to WT. These data suggested that increasing the H+-ATPase activity enhances Al sensitivity.
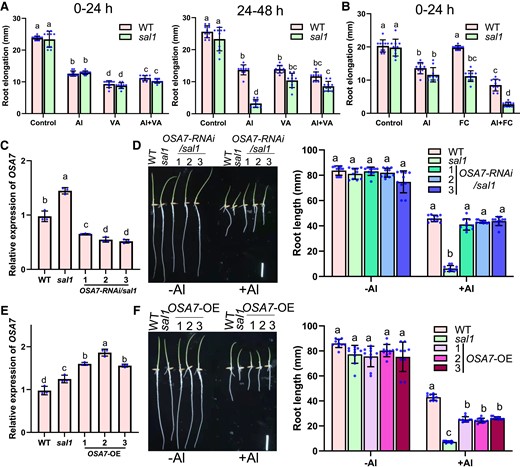
Increased PM H+-ATPase activity induces the Al hypersensitivity of sal1. A, B) Effect of vanadate (VA) A) or FC B) supply on Al resistance during the first and/or second 24-h treatment. Five-day-old seedlings of WT and sal1 were exposed to a 0.5 mM CaCl2 solution (pH 4.5) containing 0 or 20 µM Al and with or without 250 µM Na3VO4 or 1 µM FC for 24 and/or 48 h, and root elongation was calculated for the evaluation of Al resistance. C, D) Knockdown of OSA7 by RNAi rescues the Al-hypersensitive phenotype of sal1. C) Decreased expression of OSA7 in three OSA7-RNAi lines with sal1 background. D) Comparison of Al-resistance phenotype in WT, sal1, and three OSA7-RNAi lines with sal1 background. E, F) Overexpression of OSA7 increases Al sensitivity. Scale bar = 2 cm. E) Increased expression of OSA7 in three pZmUBQ:OSA7 overexpression lines with WT background. F) Comparison of Al-resistance phenotype in WT, sal1, and three OSA7 overexpression lines. Geminated seeds were exposed to a 0.5 mM CaCl2 solution (pH 4.5) containing 0 or 20 µM Al for 5 d, and the root length was measured for the evaluation of Al resistance. Scale bar = 2 cm. Values are means ± SD of 10 (A, B, D, and F) or 3 (C and E) biological replicates. In all bar graphs of this figure, means with different letters are significantly different (P < 0.05, analysis of variance test followed by Tukey's test).
To further prove that the increased PM H+-ATPase activity is responsible for the Al-hypersensitive phenotype of sal1, we attempted to knockout OSA7 in a sal1 background using CRISPR/Cas9 technology. However, we failed to obtain the osa7 knockout mutant, implying the essential role of OSA7 in rice plant growth. Thus, we generated OSA7 knockdown lines by RNAi technology in sal1 and WT backgrounds. We evaluated Al resistance in 3 OSA7-RNAi/sal1 lines, which expressed OSA7 at levels that were 36% to 45% of that in sal1 (Fig. 8C). The Al-hypersensitive phenotype of sal1 was fully rescued in the OSA7-RNAi/sal1 lines (Fig. 8D). In 3 OSA7-RNAi/WT lines, the expression level of OSA7 was 47% to 60% of that in WT (Supplemental Fig. S6A). We did not observe significant difference in Al resistance between WT and the OSA7-RNAi/WT lines (Supplemental Fig. S6, B and C).
We also attempted to increase OSA7 expression using the ubiquitin promoter to examine its effect on Al resistance. The expression level of OSA7 in 3 OSA7 overexpression lines was 1.6- to 1.9-fold higher than that in WT (Fig. 8E). All 3 lines showed higher sensitivity to Al than WT, but lower Al sensitivity than sal1 (Fig. 8F). Together, these results indicated that increasing PM H+-ATPase activity causes hypersensitivity of sal1 to Al.
Knockout of NRAT1 rescues the Al-hypersensitive phenotype of sal1
NRAT1 is a PM-localized transporter mediating Al uptake, and knockout of NRAT1 causes enhanced Al sensitivity because of increased Al toxicity in the cell wall (Xia et al. 2010). NRAT1 is an NRAMP family member that transports metal ions using the protomotive force (Courville et al. 2006; Nevo and Nelson 2006). To investigate whether the sal1 mutation promotes Al uptake through enhancing NRAT1-mediated transport of Al, we mutated NRAT1 in both WT and sal1 by CRISPR/Cas9-mediated editing of the NRAT1 coding region. We generated a knockout mutation with 1-bp (A) insertion located 114 bp downstream of the start codon of NRAT1 in both WT and sal1 backgrounds. The nrat1 mutation in the WT background reduced Al resistance (Fig. 9, A and B), which is consistent with a previous observation (Xia et al. 2010). Interestingly, mutation of NRAT1 in the sal1 background rescued the Al-hypersensitive phenotype of sal1 at all Al concentrations (Fig. 9, A and B).
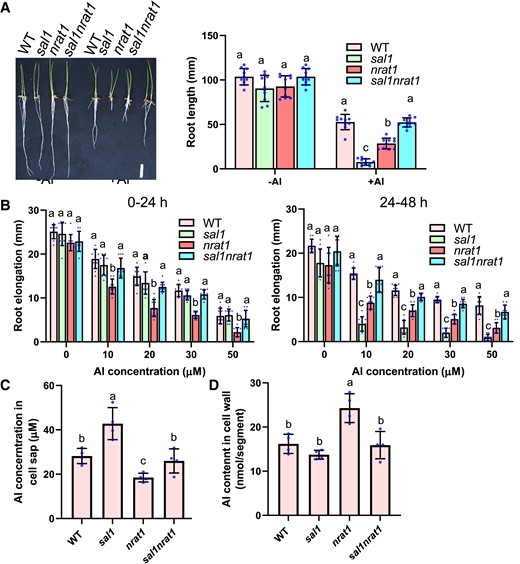
Mutation of NRAT1 recovers the Al-hypersensitive phenotype of sal1. A) Comparison of root growth of WT, sal1, nrat1, and sal1nrat1 in response to Al stress. Geminated seeds were exposed to a 0.5 mM CaCl2 solution (pH 4.5) containing 0 (−Al) or 20 µM Al (+Al) for 5 d, and the root length was measured for the evaluation of Al resistance. Scale bar = 2 cm. B) Root elongation of 0 to 24 h (left panel) and 24 to 48 h (right panel) treatments of different Al concentrations. Five-day-old seedlings of WT, sal1, nrat1, and sal1nrat1 were exposed to a 0.5 mM CaCl2 solution containing 0, 10, 20, 30, or 50 µM Al at pH 4.5 for 48 h. Root elongation during the first and second 24-h treatments were measured and compared. C, D) Al content in cell sap (C) and cell wall (D) of WT, sal1, nrat1, and sal1nrat1. Five-day-old seedlings were exposed to a 0.5 mM CaCl2 solution (pH 4.5) containing 20 µM Al for 6 h, and then root tips (0 to 1 cm) were harvested and fractionated into cell sap and cell wall for Al determination. Values are means ± SD of 10 (A and B) 3 (C and D) biological replicates. In all bar graphs of this figure, means with different letters are significantly different (P < 0.05, analysis of variance test followed by Tukey's test).
Determination of Al content showed that in accordance with previous data (Xia et al. 2010), the nrat1 single mutant accumulated a lower and higher level of Al in the symplasm and cell wall than WT, respectively, which was in contrast to sal1 that had increased Al accumulation in the symplasm (Fig. 9, C and D). The sal1nrat1 double mutant accumulated a similar level of Al in the cell wall and symplasm to WT (Fig. 9, C and D). These data further proved that the Al-hypersensitive phenotype of sal1 is induced by internal Al toxicity and suggested that NRAT1 plays an important role in the sal1-mediated increased Al accumulation in the symplasm. In addition, the sal1 mutation rescued the Al-sensitive phenotype and the increased Al accumulation in the cell wall of nrat1 (Fig. 9, C and D), which suggested that the sal1 mutation also promotes Al accumulation in the symplasm through other unknown Al transporters and consequently attenuates the cell wall Al toxicity in nrat1. Together, these results revealed that the sal1 and nrat1 mutations induce Al toxicity in the symplasm and cell wall, respectively, and suggest that the coordination of Al accumulation in the cell wall and symplam is critical for Al resistance in rice.
Dysfunction of Arabidopsis PP2C.D phosphatases increases Al resistance
To investigate whether Arabidopsis PP2C.D phosphatases play a similar role in Al resistance to SAL1, we ordered T-DNA insertion mutants of AtPP2C.D5, AtPP2C.D6, and AtPP2C.D7, which negatively regulate the PM H+-ATPase activity (Spartz et al. 2014; Ren et al. 2018). Real-time quantitative reverse transcription polymerase chain reaction (RT-qPCR) analyses revealed that the 3 PP2C.D phosphatase-encoding genes were knocked out or knocked down in their respective T-DNA mutants (Supplemental Fig. S7, A and B). We generated the Atpp2c.d5d6d7 triple mutant via hybridization to examine its Al resistance. In contrast to the hypersensitivity of sal1 to Al, the Atpp2c.d5d6d7 triple mutant was more resistant to Al than its WT (Fig. 10A). We then examined the phenotype of aha2-4 mutant, which has reduced the PM H+-ATPase activity (Haruta et al. 2010; Haruta and Sussman 2012). The aha2-4 mutant was more sensitive to Al stress than Col WT (Fig. 10A). Thus, PM H+-ATPase plays a positive role in Al resistance in Arabidopsis. PM H+-ATPase activity has been documented to positively modulate organic acid exudation in soybean (Shen et al. 2005; Liang et al. 2013). To investigate whether organic acid secretion was affected by the altered PM H+-ATPase activity, we compared the level of malate secretion in Col WT, Atpp2c.d5d6d7, and aha2-4. No difference in malate secretion between Col WT, Atpp2c.d5d6d7, and aha2-4 under control conditions was observed. However, the level of Al-triggered malate secretion was higher and lower in Atpp2c.d5d6d7 and aha2-4, respectively, than in Col WT (Fig. 10B). These results suggest that Arabidopsis PP2C.D phosphatases negatively regulate Al resistance by modulating malate secretion in Arabidopsis.
![Mutation of PP2C.D phosphatases increases Al resistance in Arabidopsis and model for the Al resistance regulated by PP2C.D phosphatases. A) Comparison of Al resistance phenotype of Col WT, Atpp2c.d5d6d7, and aha2-4. Seedlings were grown on a soaked gel medium containing 0, 0.75, 1.0, or 1.25 mM Al for 7 d, and root lengths were measured and compared. Values are means ± SD of 10 root lengths. Means with different letters are significantly different (P < 0.05, analysis of variance [ANOVA] test followed by Tukey's test). Scale bar = 2 cm. B) Increased malate secretion in Atpp2c.d5d6d7. Twelve-day-old seedlings were treated with 0 (−Al) or 20 μM Al (+Al) for 12 h, and the root exudates were analyzed for the malate concentrations (pmol plant−1 h−1). Values are means ± SD of three biological replicates. Means with different letters are significantly different (P < 0.05, ANOVA test followed by Tukey's test). C) Model for the Al resistance regulated by rice SAL1 (leaf panel) and Arabidopsis PP2C.D phosphatases (right panel). The plasma membrane-localized SAL1 inhibits PM H+-ATPase activity via dephosphorylation and PM H+-ATPase promotes NRAT1-mediated Al uptake by generating a proton gradient in rice. Thus, dysfunction of SAL1 increases PM H+-ATPase activity and Al uptake, which leads to the increased internal Al toxicity. While knockout of NRAT1 in WT background increases cell wall Al toxicity, knockout of NRAT1 in sal1 background rescues the increased Al sensitivity of both sal1 and nrat1. In contrast, Arabidopsis PP2C.D phosphatases play a negative role in Al resistance by inhibiting PM H+-ATPase-facilitated malate secretion, which increases Al resistance by chelation and exclusion of Al.](https://oup.silverchair-cdn.com/oup/backfile/Content_public/Journal/plphys/192/2/10.1093_plphys_kiad122/2/m_kiad122f10.jpeg?Expires=1747942579&Signature=Rq49XJzuaRTjC1EhMtxj~-sIz74Fql64duoJ~1WH-xOgXpMTVyZrXB2yhoUTEyP48qP7m-Z~B6msgZgyW75~YudGD4LAohdH6WhK488oWKE5~zmYJslgme5i5~tYzS4yoWSxWyoPWsw-lh5EWUnjwjRBisUzRfeGAuCSflIFG6UfWlcNOXOJeAsI6MQDtPOU4~7RDzWcCM8tW5ZzJ9aHto6DTrOPMN~IEvy0k8KzK0V~GF2XviU9BY7fRm2jAVSCluDDxb6PCLKrxcRcfUchLnj9seG4KGcH7zWWK7p2vuej0lXD4W296BVqWKF~rZzzEn6iAzOn0VaVRcnT0ws82g__&Key-Pair-Id=APKAIE5G5CRDK6RD3PGA)
Mutation of PP2C.D phosphatases increases Al resistance in Arabidopsis and model for the Al resistance regulated by PP2C.D phosphatases. A) Comparison of Al resistance phenotype of Col WT, Atpp2c.d5d6d7, and aha2-4. Seedlings were grown on a soaked gel medium containing 0, 0.75, 1.0, or 1.25 mM Al for 7 d, and root lengths were measured and compared. Values are means ± SD of 10 root lengths. Means with different letters are significantly different (P < 0.05, analysis of variance [ANOVA] test followed by Tukey's test). Scale bar = 2 cm. B) Increased malate secretion in Atpp2c.d5d6d7. Twelve-day-old seedlings were treated with 0 (−Al) or 20 μM Al (+Al) for 12 h, and the root exudates were analyzed for the malate concentrations (pmol plant−1 h−1). Values are means ± SD of three biological replicates. Means with different letters are significantly different (P < 0.05, ANOVA test followed by Tukey's test). C) Model for the Al resistance regulated by rice SAL1 (leaf panel) and Arabidopsis PP2C.D phosphatases (right panel). The plasma membrane-localized SAL1 inhibits PM H+-ATPase activity via dephosphorylation and PM H+-ATPase promotes NRAT1-mediated Al uptake by generating a proton gradient in rice. Thus, dysfunction of SAL1 increases PM H+-ATPase activity and Al uptake, which leads to the increased internal Al toxicity. While knockout of NRAT1 in WT background increases cell wall Al toxicity, knockout of NRAT1 in sal1 background rescues the increased Al sensitivity of both sal1 and nrat1. In contrast, Arabidopsis PP2C.D phosphatases play a negative role in Al resistance by inhibiting PM H+-ATPase-facilitated malate secretion, which increases Al resistance by chelation and exclusion of Al.
Discussion
In this study, we identified SAL1 encoding a PP2C.D phosphatase as a key player in regulating Al resistance in rice by a forward genetic screen. We revealed that SAL1 is anchored to the PM via palmitoylation and inhibits the activity of PM H+-ATPase through dephosphorylation. The PM H+-ATPase generates a proton gradient to facilitate NRAT1-mediated Al uptake (Fig. 10C). Mutation of SAL1 increased the PM H+-ATPase activity and consequently promoted Al uptake by NRAT1 and other unknown Al transporters, which elevated internal Al toxicity and root growth inhibition. Knockout of NRAT1 rescued the Al-hypersensitive phenotype of sal1, and the sal1 mutation also enabled the recovery of the increased Al sensitivity phenotype in nrat1. Our results thus reveal that optimal distribution of Al in the cell wall and symplasm is crucial for Al resistance in rice. We further demonstrated that PP2C.D phosphatases play opposing roles in Al resistance in Arabidopsis and rice. Although Arabidopsis and rice PP2C.D phosphatases play a conserved role in the inhibition of PM H+-ATPase activity via dephosphorylation, Arabidopsis PP2C.D phosphatases negatively regulate Al resistance through the inhibition of PM H+-ATPase-facilitated malate secretion, which is a major Al-resistance mechanism in Arabidopsis (Fig. 10C).
Phytotoxic Al3+ is the hardest Lewis acid with a low covalent index and a high ionic index, which renders Al3+ having strong interactions with organic molecules containing oxygen groups (Poschenrieder et al. 2008). Most of Al is bound to the cell wall because the cell wall bears a large number of oxygen groups and a negative charge (Kochian 1995; Ma 2007). By using synchrotron-based low-energy x-ray fluorescence spectromicroscropy and high-resolution secondary ion mass spectroscopy methods, Kopittke et al. (2015) further revealed that most Al localizes in the walls of outer cells. Rapid inhibition of root growth is considered the primary Al toxicity symptom that occurs within hours of exposure to Al (Ma 2007). Recent high-resolution kinematic analyses demonstrated that Al inhibits soybean root growth after only 5 min exposure to a high Al concentration (75 μM) or after 90 min exposure to 10 μM Al (Kopittke et al. 2015). On the basis of high-resolution spatial distribution data of Al and Al-induced root growth inhibition, Kopittke et al. (2015) proposed that the primary lesion of Al is apoplastic. Our results, however, revealed that symplasic Al also plays a critical role in Al toxicity. Interestingly, we found that the sal1 mutant showed greater root growth inhibition than WT after exposure to Al stress for more than 24 h (Fig. 1), which suggests that the internal Al toxicity in the mutant is accumulative and requires a longer term to induce root growth inhibition. Our results also imply that long-term Al toxicity plays an important role in Al-induced root growth inhibition and should not be ignored.
Al stress can increase or decrease the PM H+-ATPase activity in different plant species (Matsumoto 1988; Ahn et al. 2001; Shen et al. 2005; Zhang et al. 2019a). We showed that rice PM H+-ATPase activity increases in response to Al stress, which was attributed to increases in both protein and phosphorylation levels of the PM H+-ATPase (Fig. 7, B and C). In contrast, although Al stress slightly increased the protein level of SAL1 that negatively regulates the PM H+-ATPase activity (Fig. 4C), the phosphatase activity of SAL1 is inhibited significantly by the Al stress (Fig. 3D). Thus, Al stress-triggered phosphorylation and activity of PM H+-ATPase might be partly through inhibiting the phosphatase activity of SAL1. The increased expression of SAL1 under Al stress may rapidly reduce the PM H+-ATPase activity once Al stress is removed, which may be important for maintaining PM H+-ATPase homeostasis. SMALL AUXIN UP RNAs (SAURs) are reported to inhibit PP2C.D phosphatases and thus activate the PM H+-ATPase activity (Ren and Gray 2015). Determining the role of SAURs in Al-triggered the PM H+-ATPase activity is a future research endeavor.
Although PM H+-ATPase activity is triggered by Al stress, reducing the expression of OSA7 encoding the major root PM H+-ATPase did not affect Al resistance significantly (Supplemental Fig. S6), suggesting that the increase of PM H+-ATPase activity in response to Al does not play an important role in Al resistance. Because Al stress interferes with plant uptake and transport of essential nutrients (Singh et al. 2017), the increase of the PM H+-ATPase activity may facilitate nutrient uptake and transport to counteract the negative effect of Al stress. However, a further increase of the PM H+-ATPase activity in sal1 enhances Al uptake and, consequently, the increased accumulation of Al in the symplasm, which results in the hypersensitivity of sal1 to Al. Our results thus revealed that SAL1 plays a crucial role in Al resistance by damping the PM H+-ATPase activity to restrict Al uptake.
In contrast to sal1, mutation of NRAT1 encoding the Al uptake transporter was found to reduce and increase Al accumulation in the symplast and cell wall, respectively (Fig. 9, C and D) (Xia et al. 2010). Nevertheless, like sal1, nrat1 is also more sensitive to Al than WT (Fig. 9, C and D). These observations highlight that Al can target both the cell wall and symplastic components to confer Al toxicity and suggest that Al accumulation in the cell wall and symplasm should be tightly controlled and balanced for Al detoxification in rice. The coordination between cell wall and symplastic Al detoxification has also been suggested previously (Huang et al. 2012; Zhu et al. 2013); nevertheless, the direct genetic evidence supporting the importance of this coordination is lacking. Here, we showed that simultaneous mutations of NRAT1 and SAL1, which regulate cell wall and symplastic Al detoxification, respectively, displayed a similar Al accumulation pattern and Al resistance phenotype to WT, clearly demonstrating the importance and impact of the coordination between cell wall and symplastic Al detoxification. In addition, because the sal1nrat1 double mutant showed reduced and increased Al accumulation in the cell wall and the symplasm, respectively, in comparison with nrat1, the sal1 mutation also facilitates Al uptake to attenuate cell wall Al toxicity in nrat1 through other unknown Al transporters, which remains to be identified and characterized in the future.
Similar to Arabidopsis PP2C.D phosphatases, rice SAL1 is also involved in dephosphorylating and inhibiting PM H+-ATPases. However, they play an opposite role in regulating Al resistance because sal1 is hypersensitive to Al, whereas mutations of Arabidopsis PP2C.D phosphatase-encoded genes AtPP2C.D5 to AtPP2C.D7 increase Al resistance (Fig. 10A). The enhanced Al resistance in the Atpp2c.d5d6d7 triple mutant can be attributed to increased malate secretion triggered by the elevated PM H+-ATPase activity (Fig. 10B). Our results suggest that rice and Arabidopsis adopt different strategies to detoxify Al. Arabidopsis utilizes malate secretion-based Al exclusion as a major mechanism to reduce Al toxicity in the cell wall, and PP2C.D phosphatases play a negative role in regulating malate exudation. In contrast, rice introduces apoplastic Al into the symplasm via Al uptake transporters including NRAT1 to reduce the cell wall Al toxicity (Xia et al. 2010), and SAL1 negatively regulates the activity of the Al transporters to prevent excess Al uptake and increased Al toxicity in the symplasm.
Materials and methods
Plant materials
Indica rice (O. sativa) variety Kasalath was used as the wild type for all the control experiments. The Al-sensitive mutant sal1 was screened previously from an EMS-mutagenized population by a highly efficient method (Liu et al. 2016). To produce knockout mutant alleles of ART1 and NRAT1, the CRISPR/Cas9-based genome editing method was used. Two different 20-bp coding sequences (Supplemental Table S3) of ART1 and NRAT1 were designed and constructed into a sgRNA-Cas9 expression vector (Ma and Liu 2016), and the resultant vector was transformed into both WT and/or sal1 mutant background. Homozygous nrat1 mutants in WT and sal1 background were identified by sequencing the target regions of ART1 and NRAT1. To generate knockdown lines of OSA7 by RNAi technology, a portion of OSA7 coding sequence (504 bp) was amplified by a primer pair (Supplemental Table S3) and cloned into pANDA vector using GATEWAYTM cloning technology. To generate overexpression lines of OSA7, its coding sequence was amplified by a primer pair (Supplemental Table S3) and cloned into pEarlygate101 vector (XbaI and HindIII restriction sites) harboring maize ubiquitin promoter. The resultant vectors were transformed into WT and sal1 calli by Agrobacterium (EHA105 strain)-mediated transformation method, respectively, and homozygous transgenic lines were used for the expression and phenotypic analyses.
Arabidopsis mutants d5 (GABI_330E08), d6 (SALK_202796), d7 (SALK_118712), and aha2-4 (SALK_082786) were obtained from the Nottingham Arabidopsis Stock Centre (NASC) (https://arabidopsis.info/).
Evaluation of Al resistance
For evaluation of Al resistance in rice, seeds were soaked in a 0.5 mM CaCl2 solution at 37 °C for 3 d, and then the germinated seeds were sown on a net floating on a 0.5 mM CaCl2 solution (pH 4.5) containing 0, 10, or 20 µM AlCl3 at 28 °C for 5 d to measure root lengths by a ruler for the assessment of Al sensitivity. For the evaluation of Al sensitivity within 48 h, the soaked seeds were sown on the 0.5 mM CaCl2 solution (pH 4.5) for 2 d and then transferred to the same 0.5 mM CaCl2 solution containing 0, 10, 20, 30, or 50 µM AlCl3 for 48 h at 28 °C. The root lengths were measured at 3 time points: 0, 24, and 48 h treatments. Root elongation calculated from the root lengths at the 3 time points or relative root elongation expressed as root elongation with Al treatment/root elongation without Al were used to evaluate the Al sensitivity.
To examine the effect of vanadate and FC on Al sensitivity, 5-day-old seedlings of WT and sal1 were exposed to a 0.5 mM CaCl2 solution (pH 4.5) containing 0 or 20 µM AlCl3 and with or without 250 µM Na3VO4 or 1 µM FC at 28 °C for 24 and/or 48 h. Root elongation was calculated from the first and/or second 24 h treatment to determine the impact of vanadate and FC on the Al sensitivity of WT and the mutant. Ten seedlings of each line at each treatment were used for the evaluation of Al sensitivity.
For the evaluation of Al resistance in Arabidopsis, a modified soaked gel medium method was used to assess Al resistance, which has been described previously (Larsen et al. 2005; Zhang et al. 2019b). Seeds of Col WT, Atpp2c.d5d6d7, and aha2-4 were sowed on the soaked gel medium for 7 d, and root lengths were measured by Image J software for the evaluation of Al resistance.
To examine whether sal1 is sensitive to Al toxicity in acidic soil, germinated seeds of WT and sal1 were grown on neutral or acidic soils for 7 d, and then root length was compared for the evaluation of Al resistance. Parent material type of the acidic soil is quaternary red clay. Soil pH was measured using a pH meter after mixing dry soils with deionized water (soil/water = 1/2.5) and stewing for half an hour. To determine soluble Al in the soils, 0.3 g soils was mixed with 15 mL of 1 M KCl and shook on a shaker for 1 h. The mixtures were then centrifuged at 5000 rpm for 15 min, and the supernatants were filtered and subjected for the Al measurement. The spectrophotometric pyrocatechol violet method was used to determine the Al concentration, which has been described previously (Kramer et al. 1994).
Evaluation of low pH and other metal tolerance
To evaluate the tolerance to low pH, germinated seeds of WT and sal1 mutant were grown on a 0.5 mM CaCl2 solution at pH 4.5, 4.0, or 3.5 at 28 °C for 5 d, and then the root length was measured for the determination of low pH tolerance. For the evaluation of other metal tolerance, germinated seeds of WT and sal1 were grown on a 0.5 mM CaCl2 solution containing 10 µM CdCl2, 10 µM LaCl3, or 0.5 µM CuCl2 at pH 4.5. After growth for 5 d, the root length was measured and compared.
Determination of Al accumulation in roots
Five-day-old seedlings of WT and sal1 mutant were exposed to a 0.5 mM CaCl2 solution (pH 4.5) containing 20 µM Al at 28 °C for 6 h, and the roots were then stained 0.1% (w/v) Eriochrome Cyanine R for 10 min. After washing with distilled water for 10 min, the roots were photographed by a stereo microscope (SZX12, Olympus) equipped with a camera (DP26, Olympus). For morin staining, the roots were excised and embedded in 5% (w/v) agar. Root tips were transversely sectioned at around 3 mm from apexes by a vibrating blade microtome (Leica VT1200S, Leica) and then stained in 0.2% (w/v) of morin for 10 min. The green fluorescence signal was observed with a laser scanning microscopy (LSM880, ZEISS) using 488 nm excitation radiation and observing 500 to 530 nm of emission.
To measure Al content in different compartments of roots, 5-day-old seedlings were exposed to a 0.5 mM CaCl2 solution (pH 4.5) containing 20 µM Al at 28 °C for 0.5, 3, or 6 h, and then root tips (0 to 1 cm) were harvested. Fractionation of the cell sap and cell wall has been described previously (Xia et al. 2010; Liu et al. 2016). Measurement of Al concentration in a solution was determined by inductively coupled plasma mass spectrometry (ICP-MS, PerkinElmer NexION300D).
Measurement of malate secretion in Arabidopsis roots
Twelve-day-old seedlings of Col-0, Atpp2c.d5d6d7 and aha2-4 grown on 1/2 MS nutrient agar medium containing 1% (w/v) sucrose were pretreated with a 2% (v/v) MGRL solution (Fujiwara et al. 1992) with 1% sucrose for 2 h at pH 4.8 and then exposed to the same solution containing 0 or 20 μM AlCl3 for 12 h in a 12-well plate. Root exudates from 20 seedlings in each of 3 biological replicates were collected and concentrated by lyophilizing (CHRIST ALPHA 1-2 LDplus, Germany). The NAD/NADH enzymatic cycling method (Hampp et al. 1984) was adopted to measure the malate concentration in the root exudates.
Cloning of SAL1
The sal1 mutant was crossed with the WT to generate an F2 population for genetic analysis and mapping-by-sequencing. Pooled DNA from 92 F2 Al-sensitive seedlings and WT DNA were, respectively, subjected for high-throughput DNA sequencing using the Illumina HiSeq4000 system, which was performed by a commercial company (Shanghai Hanyu Biotech lab, Shanghai, China) (accession number in NCBI: SRR11714511 and SRR11714512). The sequencing data of 250-bp paired-end reads had a depth of around 27-fold coverage of the rice genome. Clean reads were mapped to the Kasalath reference sequence database (https://rapdb.dna.affrc.go.jp/download/archive/irgsp1/kasalath_genome.tar.gz) by using bwa (version: 0.7.10) with default parameters. The aligned reads were analyzed by GATK (version: 3.5, Broad institute) for calling single nucleotide polymorphisms (SNPs). The MutMap method (Abe et al. 2012) was used to search the candidate region of sal1. To confirm the candidate region of sal1, 4 dCAPS polymorphic markers (Supplemental Table S3) were developed based on single-nucleotide polymorphisms in the candidate region of sal1, which was used to perform linkage analysis in 58 F2 Al-sensitive seedlings. The dCAPS maker X4 developed from a C-to-T substitution in the coding sequence of LOC_Os06g50380 was completely linked to the Al-sensitive phenotype (Supplemental Table S1), suggesting that the mutation in LOC_Os06g50380 might cause the Al hypersensitivity in sal1.
A complementation test was performed to confirm LOC_Os06g50380 is SAL1. A DNA fragment consisting of a 3.5-kb promoter and the gene of LOC_Os06g50380 without stop codon was amplified by a primer pair (Supplemental Table S3) and inserted in frame with 3 x hemagglutinin (HA) tag into the pCAMBIA3301 vector (XbaI and HindIII restriction sites). The construct was then transformed into sal1 mutant, and 3 independent homozygous transgenic lines were screened and subjected for the complementation analysis.
Phylogenetic analysis
Multiple alignments were performed with ClustalW using default settings in BioEdit software, and the phylogenetic tree was constructed by MEGA6.0 software using the neighbor-joining method with 1,000 bootstrap trials.
RNA extraction and RT-qPCR analysis
To examine the expression pattern of SAL1, different tissues of 90-day-old WT plants including roots, stems, leaf blades and sheathes, and panicles were sampled for RNA isolation. For the expression analysis of SAL1/sal1 and OSA7 in response to Al stress, 5-day-old seedlings were exposed to a 0.5 mM CaCl2 solution (pH 4.5) containing 0 or 20 μM AlCl3 at 30 °C for 6 h, and then root tips (0 to 1 cm) were excised for the RNA isolation. Total RNA of each sample was extracted by the TaKaRa MiniBEST plant RNA Extraction Kit (Cat # 9769), and then 1 μg of the total RNA was used for first-strand cDNA synthesis using the HiScript II Q Select RT SuperMix (Vazyme Biotech Co., Ltd., China). RT-qPCR analysis of each gene was performed by using the cDNA product as a template and the SYBR® Green Master Mix kit (Vazyme Biotech Co., Ltd., China). Histone H3 (Os06g0130900) was used as an internal control. RT-qPCR was run on the CFX96 Touch real-time polymerase chain reaction detection system (Bio-Rad). The sequence information of the primers used for expression analysis is listed in Supplemental Table S3.
Subcellular localization analysis of SAL1
The coding sequence of SAL1/sal1 without stop codon was amplified and cloned into pEZS-NL vector harboring the 35S promoter and eGFP gene to produce 35S:SAL1/sal1-eGFP construct. Mutated versions of SAL1 with different number of mutation sites on the 3 potential sites (Cys12, Cys151, and and Cys152) were generated by site-directed mutagenesis using 35S:SAL1-eGFP as a template and oligonucleotide primers listed in Supplemental Table S3. Each resultant vector was transformed into rice protoplasts for GFP fluorescence observation by a confocal laser scanning microscopy (Zeiss LSM880, CARL ZEISS) using 488 nm excitation radiation and observing 500 to 530 nm of emission. Chlorophyll fluorescence was also observed with the microscopy using 488 nm excitation radiation and observing 650 to 750 nm of emission to assist the determination of eGFP subcellular localization.
Yeast 2-hybrid and complementation assays
To test the interaction between SAL1 and different PM H+-ATPases, the split-ubiquitin yeast 2-hybrid system was used. The coding sequences of SAL1 and OSA6 to OSA10 were cloned into pBT3-STE (SfiI restriction site) and pPR3-N (SfiI restriction site) vectors, respectively. The resultant SAL1 and OSA plasmids were co-transformed into yeast strain NMY51 and selected on SD (-Leu/Trp) medium. Positive yeast clones were grown on SD (-Leu/Trp) and SD (-Leu/Trp/His/Ade) containing 2 mM 3-amino-1,2,4-triazole (3-AT) media for 3 d to determine the protein–protein interaction.
For the yeast complementation assays, Saccharomyces cerevisiae strain RS-72 (Mata; ade1-100his4-519leu2-3,312pPMA1::pGAL1) was used (Fuglsang et al. 2007). The coding sequence of SAL1 was amplified and cloned into pMP1645 vector (NotI restriction site), while the coding sequences of OSA7, OSA8, or OSA9 were cloned into pMP1612 vector (NotI restriction site). The 2 vectors contain the same constitutive PGK promoter for target gene expression (Fuglsang et al. 2007). The constructed vectors or control vectors were transformed into the RS-72 strain. Positive cloned selected on SD (-His/Ade) medium were cultured in SD (-His/Ade) liquid medium containing 2% (w/v) galactose for the amplification and were then spotted on SD (-His/Ade) medium containing 2% (w/v) galactose or glucose for 5 d growth at 30 °C. The primers used for the vector construction are listed in Supplemental Table S3.
Split-LUC complementation and co-IP assays
For the split-LUC complementation analysis, coding sequences of SAL1 and OSA7 were cloned into pCAMBIA1-cLUC (KpnI and PstI restriction sites) and pCAMBIA1-nLUC (KpnI and SalI restriction sites), respectively. The resultant SAL1-cLUC and OSA7-nLUC vectors were co-transformed into 2-week-old Nicotiana benthamiana leaves by Agrobacterium-mediated transformation. The transformed plants were grown in the dark for 1 d and in the long-day conditions for 2 d, and then subjected for LUC signal detection by a chemiluminescent imaging system (Tanon 5200) to determine whether the 2 proteins interacted with each other.
For the co-IP assay, 1 ml of rice protoplasts (∼2 × 106 cells) were co-transfected with 10 μg SAL1-3HA or Rac1-3HA and with 10 μg OSA7-2Flag. After incubation for overnight in the dark, the protoplasts were subjected for total protein extraction by using the lysis buffer (500 μL) containing 50 mM Tris-HCl (pH 8.0), 150 mM NaCl, 5 mM EDTA-2Na, 1% (w/v) n-dodecyl β-D-maltoside, and 1×protease inhibitor cocktail (5892791001; Roche), and 20 μL of protein extract was used as the input control. The rest protein extract was incubated with anti-HA magnetic beads (B26201; Bimake, USA) for 2 h. After the extracts were washed 3 times with protein extraction buffer, the bound proteins were eluted with 1×sodium dodecyl sulfate sample loading buffer for immunoblot analysis. HA- and Flag-fuzed proteins were detected by anti HA-horseradish peroxidase (HRP) (12013819001, Lot 44323100; Roche) and Flag-HRP (A8592; Sigma-Aldrich) antibodies, respectively.
SAL1 protein expression and phosphatase assays
The coding sequences of SAL1 or sal1 were cloned into pET29a (+) vector (BamHI and XhoI restriction sites) to generate SAL1-His or sal1-His recombinant plasmids. The plasmid containing SAL1-His or sal1-His was transformed into E. coli strain BL21 (DE3) for target protein expression. The transformed cells were lysed and purified with Ni-TED sefinose (TM) resin 6 FF (C610030, Songon, China). Purified proteins were quantified using the Quick Start Bradford reagent (5000205, Bio-Rad, USA). The purified SAL1-His and sal1-His proteins were confirmed and detected by the immunoblot analysis using anti-His antibody (GNI4110-HS; GNI, Japan). To analyze the phosphatase activity of purified proteins, 0.5 μg of SAL1-His or sal1-His was incubated in 500 μl of assay buffer consisting of 75 mM Tris (pH 7.6), 0.5 mM EDTA, 100 mM NaCl, 5 mM pNPP, and 10 mM MnCl2, ZnCl2, or MgCl2. Absorbance at 405 nm was recorded after 20 min in a Miomate 3S spectrometer (Thermo Scientific). A standard curve was generated using 4-nitrophenol to calculate the phosphatase activity.
To determine the effect of Al stress on protein accumulation and phosphatase activity of SAL1 in rice, 5-day-old seedlings of a pSAL1:SAL1-HA complementation line were treated with or without 20 μM AlCl3 for 6 h, and then root tips (0 to 1 cm) were sampled for protein extraction. The total protein fraction was extracted by macerating frozen tissue in protein extraction buffer containing 20 mM Tris–HCl (pH 7.5), 300 mM NaCl, 5 mM MgCl2, 0.5% (v/v) NP-40, 5 mM DTT, 50 μM MG132 (A2585, APExBIO, USA), and 1× complete protease inhibitor mixture. The extracted proteins were subjected to the immunoblot analysis of SAL1-HA using the anti HA-HRP antibody for the comparison of protein levels of different samples. For the detection of SAL1-HA phosphatase activity, the extracted proteins were immunoprecipitated with 20 μL anti-HA immunomagnetic beads (B26201; Bimake, USA) for 2 h at room temperature. After washing beads with 1× PBST (phosphate-buffered saline with Tween 20) buffer (136.9 mM NaCl, 2.67 mM KCl, 8.1 mM Na2HPO4, 1.76 mM KH2PO4, 0.5% (v/v) Tween20) for 3 times, the immunoprecipitated proteins were eluted with 100 μL 0.1 M glycine (pH 2.5) for 5 min at 25 °C and then added with 1 M Tris–HCl (pH 9.0) for the neutralization. About 0.5 μg eluted SAL1-HA protein was used for the phosphatase activity assay as described earlier.
PM H+-ATPase abundance, phosphorylation, and activity assays
Five-day-old seedlings of WT and sal1 were treated with 0.5 mM CaCl2 solution (pH 4.5) containing 0 or 20 μM AlCl3 for 6 h and then root tips (0 to 1 cm) were sampled. For comparison of the abundance of PM H+-ATPase, total proteins were extracted and subjected to the immunoblot analysis using anti-PM H+-ATPase rabbit antibody (Hayashi et al. 2010; Toda et al. 2016). The Actin protein was used as the loading control and detected by anti-Actin antibody (CW0096; Cwbio, China). For PM H+-ATPase phosphorylation and activity assays, PM of the root tip samples was isolated according to a previous method (Larsson et al. 1994). Around 10 μg PM proteins were used for the immunoblot analysis of PM H+-ATPase phosphorylation using anti-pThr antibody recognizing the penultimate threonine of PM H+-ATPase (Hayashi et al. 2010; Toda et al. 2016). For the PM H+-ATPase activity assay, 50 μg PM proteins were incubated in 300 μ1 solution (0.33 M sucrose, 50 mM MES-KOH (pH 6.5), 3 mM ATP, 4 mM MgSO4, 50 mM KNO3, 1 mM NaN3, 0.1 mM Na2MoO4, 0.1 mM EDTA, and 1 mM DTT) with or without 0.1 mM Na3VO4. The reaction was initiated by adding ATP and run at 25 °C for 30 min. Inorganic phosphate products determined by absorbance at 860 nm were used for the calculation of the PM H+-ATPase activity.
GUS assay
To generate pSAL1:SAL1-GUS transgenic plants, the 3.5-kb promoter and the gene of SAL1 without stop codon were amplified and fuzed in frame to uidA gene encoding β-glucuronidase (GUS) in the pORE-R2 vector (XhoI and EcoRI restriction sites). The construct was introduced into WT plants by Agrobacterium-mediated transformation. Transgenic plants harboring the homozygous pSAL1:SAL1-GUS transgene were selected for the GUS analysis. Leaf and root tissues of 30-day-old plants grown on half-strength Kimura nutrient solution were collected and stained with a commercialized GUS staining solution (161031, o'Biolab Co., Ltd., Beijing, China) for overnight at 37 °C. Stained roots of pSAL1:SAL1-GUS transgenic lines were imbedded in 5% (w/v) agar for cross sectioning with an ultramicrotome (Lecia EM UC7). Stained tissues were observed with a stereomicroscope (SZX12, Olympus) and were imaged with a camera (DP20, Olympus) fixed on the stereomicroscope.
Statistical analyses
Two-tailed student's t test was used to conduct statistical analysis on 2 lines, while one-way analysis of variance followed by Tukey test was used for the statistical analysis of more than 2 lines. Quantification of immunoblot signals was performed by tracing out the individual band using ImageJ software.
Accession numbers
Sequence data from this article can be found in EMBL/GenBank data libraries under the following accession numbers: SAL1 (LOC_Os06g50380), ART1 (LOC_Os12g07280), NRAT1 (LOC_Os02g03900), OSA1 (LOC_Os03g48310), OSA2 (LOC_Os07g09340), OSA3 (LOC_Os12g44150), OSA4 (LOC_Os05g25550), OSA5 (LOC_Os08g14360), OSA6 (LOC_Os02g55400), OSA7 (LOC_Os04g56160), OSA8 (LOC_Os03g01120), OSA9 (LOC_Os03g08560), OSA10 (LOC_Os06g08310), AtPP2C.D5 (At4g38520), AtPP2C.D6 (At3g51370), and AtPP2C.D7 (At5g66080). Whole-genome sequence data of pooled F2 sal1 mutant plants and WT plants can be found in NCBI under the accession numbers, SRR11714511 and SRR11714512, respectively.
Acknowledgments
We thank Prof. Yan Guo from China Agricultural University for kindly providing yeast strain RS-72.
Supplemental data
The following materials are available in the online version of this article.
Supplemental Figure S1. Sensitivity to other stresses and morphological phenotype in sal1 (supports Fig 1).
Supplemental Figure S2. Cloning of SAL1 (supports Fig. 3).
Supplemental Figure S3. Phylogenetic and multiple alignment analyses of SAL1 and other PP2C.D phosphatases (supports Fig. 3).
Supplemental Figure S4. The SAL1-GFP fusion protein can complement the Al-hypersensitive phenotype of sal1 (supports Fig. 5).
Supplemental Figure S5. Aluminum stress and sal1 mutation increase OSA7 expression (supports Fig. 7).
Supplemental Figure S6. Knockdown of OSA7 by RNAi does not affect Al resistance (supports Fig. 8).
Supplemental Figure S7. Decreased expression of PP2C.D phosphatase-encoding genes in their respective T-DNA lines (supports Fig. 10).
Supplemental Table S1. Mutation sites and linkage analyses of sal1 on rice chromosome 6.
Supplemental Table S2. List of SAL1 interaction proteins by Y2H.
Supplemental Table S3. Primers used in this study.
Funding
This work was supported by the National Natural Science Foundation of China (Grant Nos. 32200212, 31870223, and 32170261), National Key Laboratory of Plant Molecular Genetics, the Open Research Fund of State Key Laboratory of Hybrid Rice (Hunan Hybrid Rice Research Center), and Guangdong Provincial Key Laboratory of New Technology in Rice Breeding (Grant No. 2020B1212060047).
References
Author notes
W.X. and C.-F.H. designed the research. W.X., S.L., H.G., J. W., and D. L. conducted the experiments. T.K. commented on the manuscript. W.X. and C.-F.H. wrote the manuscript.
The author responsible for distribution of materials integral to the findings presented in this article in accordance with the policy described in the Instructions for Authors (https://dbpia.nl.go.kr/plphys/pages/General-Instructions) is Chao-Feng Huang.
Conflict of interest statement. Authors declare that there is no conflict of interest.