-
PDF
- Split View
-
Views
-
Cite
Cite
Peng Zhang, Xiaoding Ma, Lina Liu, Chanjuan Mao, Yongkang Hu, Bingxiao Yan, Jia Guo, Xinyu Liu, Jinxia Shi, Gang-Seob Lee, Xiaowu Pan, Yiwen Deng, Zhengguang Zhang, Zhensheng Kang, Yongli Qiao, MEDIATOR SUBUNIT 16 negatively regulates rice immunity by modulating PATHOGENESIS RELATED 3 activity, Plant Physiology, Volume 192, Issue 2, June 2023, Pages 1132–1150, https://doi.org/10.1093/plphys/kiad120
- Share Icon Share
Abstract
Lesion mimic mutants (LMMs) are valuable genetic resources for unraveling plant defense responses including programmed cell death. Here, we identified a rice (Oryza sativa) LMM, spotted leaf 38 (spl38), and demonstrated that spl38 is essential for the formation of hypersensitive response-like lesions and innate immunity. Map-based cloning revealed that SPL38 encodes MEDIATOR SUBUNIT 16 (OsMED16). The spl38 mutant showed enhanced resistance to rice pathogens Magnaporthe oryzae and Xanthomonas oryzae pv. oryzae (Xoo) and exhibited delayed flowering, while OsMED16-overexpressing plants showed increased rice susceptibility to M. oryzae. The OsMED16-edited rice lines were phenotypically similar to the spl38 mutant but were extremely weak, exhibited growth retardation, and eventually died. The C-terminus of OsMED16 showed interaction with the positive immune regulator PATHOGENESIS RELATED 3 (OsPR3), resulting in the competitive repression of its chitinase and chitin-binding activities. Furthermore, the ospr3 osmed16 double mutants did not exhibit the lesion mimic phenotype of the spl38 mutant. Strikingly, OsMED16 exhibited an opposite function in plant defense relative to that of Arabidopsis (Arabidopsis thaliana) AtMED16, most likely because of 2 amino acid substitutions between the monocot and dicot MED16s tested. Collectively, our findings suggest that OsMED16 negatively regulates cell death and immunity in rice, probably via the OsPR3-mediated chitin signaling pathway.
Introduction
Owing to their sessile nature, plants have evolved sophisticated mechanisms to combat pathogen attacks (Jones and Dangl 2006). Hypersensitive response (HR) is one of the most efficient and prominent immune responses in plants, which triggers rapid programmed cell death (PCD) at the site of pathogen entry, to avert future pathogen invasions or proliferations in host plants (Zebell and Dong 2015). The occurrence of HR is often accompanied by typical physiological processes such as the burst of reactive oxygen species (ROS), accumulation of callose and antimicrobial compounds, expression of defense-related genes, and alterations of the plant cell wall, which subsequently contribute to the induction of pathogen-associated molecular pattern (PAMP)-triggered immunity or effector-triggered immunity (Dangl et al. 1996; Giraldo and Valent 2013; Oliveira-Garcia and Valent 2015). While the HR plays a vital role in host resistance to pathogens, the underlying mechanisms are not fully understood.
Mutagenesis has proven to be a highly valuable approach for dissecting the plant defense response and increasing the genetic variability underlying desired traits. Many mutants show spontaneous disease-like lesions without pathogen infection. Because of the formation of localized cell death-related lesions resembling those caused by the pathogen-induced HR, these mutants have been designated as lesion mimic mutants (LMMs; Lorrain et al. 2003). Over the past 3 decades, LMMs have been widely identified in a range of plant species, including Arabidopsis (Arabidopsis thaliana; Lorrain et al. 2003), barley (Hordeum vulgare; Rostoks et al. 2006), groundnut (Arachis hypogea; Badigannavar et al. 2002), maize (Zea mays; Johal et al. 1995), rice (Oryza sativa; Yin et al. 2000; Cui et al. 2020), soybean (Glycine max; Wang et al. 2020), and wheat (Triticum aestivum; Kamlofski et al. 2007). LMMs often exhibit either accelerated leaf senescence (characterized by lesions that spread uncontrollably) or delayed growth and flowering (characterized by lesions that are discrete and of determinate size; Lorrain et al. 2003; Qiao et al. 2010; Wang et al. 2015c). LMMs exhibit activated immune responses and show substantially enhanced resistance against pathogens (Li et al. 2017). Thus far, more than 60 LMM genes have been isolated from plants (Bruggeman et al. 2015), whose functional alleles encode different proteins involved in numerous disease-associated molecular pathways, such as Ca2+ signal transduction, chloroplast activity and photosynthesis, protein ubiquitination or phosphorylation, ROS production, transcriptional regulation, porphyrin and phenolic compound biosynthesis, and mRNA splicing (Zeng et al. 2004; Chen et al. 2012; Undan et al. 2012; Liu et al. 2015; Ma et al. 2019). These findings suggest that the formation of HR-like lesions in plants is a very complicated process.
Mediator (MED) is an evolutionarily conserved multisubunit complex found in all eukaryotes, ranging from yeast to humans, and plays an essential role in controlling gene expression by acting as a bridge between transcription factors and RNA polymerase II during transcription initiation (Bourbon 2008). MED has multiple functional forms and acts as a transcriptional co-activator or co-repressor (Conaway and Conaway 2011). Despite its importance, relatively few studies have been conducted on the MED complex, mainly because of the lethality of med mutants in most multicellular organisms (Caillaud et al. 2013). To date, MED subunits have been investigated in Arabidopsis, maize, rice, tobacco (Nicotiana tabacum), tomato, and wheat (Mathur et al. 2011; Wang et al. 2011; Dolan and Chapple 2017; Hiebert et al. 2020; Alonso-Nieves et al. 2021), demonstrating their role in biotic and abiotic stress responses, flowering, phytohormone signaling, immune response, growth, and development (Kidd et al. 2009; Elfving et al. 2011; Buendia-Monreal and Gillmor 2016; Liu et al. 2020; He et al. 2021). The AtMED complex has at least 34 subunits, 6 of which are specific to the plant lineage (Mathur et al. 2011). Several AtMED subunits perform specific functions in plant development and immunity. For instance, AtMED12 and AtMED13 play critical roles in regulating early embryo patterning (Gillmor et al. 2010); AtMED18 regulates root morphogenesis by modulating the expression of auxin-responsive genes (Raya-González et al. 2018); AtMED14, AtMED15, and AtMED16 act as critical regulators of the salicylic acid (SA) response, similar to NONEXPRESSOR OF PATHOGENESIS-RELATED GENES 1 (NPR1; Canet et al. 2012; Zhang et al. 2013); AtMED21 controls defense against necrotrophic pathogens by interacting with the plant RING E3 ligase HUB1 (Dhawan et al. 2009); and AtMED25 acts as a positive regulator of jasmonic acid (JA)-dependent activation of defense-related genes (Kidd et al. 2009). However, the biological functions of most MED subunits remain to be determined.
AtMED16, a well-characterized subunit, is involved in regulating cell wall integrity, circadian clock, tissue development, disease resistance, drought and osmotic stress tolerance, flowering, freezing acclimation, and iron homeostasis (Knight et al. 2009; Wathugala et al. 2012; Zhang et al. 2012; Zhang et al. 2013; Yang et al. 2014; Sorek et al. 2015; Liu et al. 2019). AtMED16 also regulates SA signaling and systemic acquired resistance pathways, conferring resistance to fungal pathogens, Botrytis cinerea and Alternaria brassicicola, as well as the bacterial pathogen Pseudomonas syringae pv. tomato (Pst) DC3000 (Wathugala et al. 2012; Zhang et al. 2013; Wang et al. 2015a). More recently, silencing of the GmMED16-1 gene was shown to increase susceptibility to Phytophthora sojae in soybean (Xue et al. 2019). These findings suggest that MED16 homologs act as positive regulators of plant immune responses in dicots; however, little is known about their role in monocots.
PATHOGENESIS-RELATED (PR) families comprise a group of inducing factors and functionally diverse proteins implicated in active plant defense, potentially restricting pathogen development and spread (van Loon et al. 2006). PR proteins have been investigated in Arabidopsis, tomato, and potato (Solanum tuberosum), and often exist as numerous isoforms in various host plants. Currently, at least 17 families of PR proteins, with enzymatic activities, are recognized in most plant species, including β-1,3-glucanases (PR2), chitinases (PR3), thaumatin-like proteins (PR5), proteases (PR6), defensins (PR12), and thionins (PR13; Wanderley-Nogueira et al. 2012; Irigoyen et al. 2020). In rice, a total of 414 PR proteins have been identified to date (Irigoyen et al. 2020); however, their exact mechanisms in plant defense remain largely unknown.
Here, we identified a rice LMM, termed spl38, which shows an HR-like cell death phenotype, chitin-induced ROS generation, and enhanced resistance to both the rice blast and blight pathogens. We further demonstrate that SPL38 encodes MED subunit 16 (OsMED16), which targets OsPR3, an endogenous chitinase that positively regulates immunity in rice. We show that OsMED16 represses the chitinase and chitin-binding activities of OsPR3 through its C-terminal domain. The cell death phenotype caused by the knockout mutation of OsMED16 was blocked in the ospr3-4 mutant. Strikingly, by comparing the amino acid sequences of OsMED16 and AtMED16, we found that 2 residues are very important for regulating the response to pathogen infection in dicots and monocots. Collectively, our data strongly suggest that OsMED16 plays a negative role in the regulation of PCD and immune response via OsPR3-mediated immunity.
Results
Phenotypic characterization of the spl38 mutant
The rice spl38 mutant was isolated from the ethyl methanesulfonate (EMS)-treated M2 population of the japonica cultivar Taichung 65 (TC65). Small, reddish-brown lesions appeared on the top 2 to 3 leaves below the flag leaf of spl38 mutant plants at the point of maximum tillering and gradually expanded to the entire leaf at the flowering stage (Fig. 1, A to C). Unlike the wild type (WT; TC65), which exhibited normal leaves, the spl38 mutant showed fewer and smaller lesions on young leaves, and discrete lesions of determinate size on old leaves. Very few or no lesions appeared on the flag leaf of spl38. Additionally, the growth and flowering of the spl38 mutant became clearly retarded with the increase in the severity of the lesion mimic phenotype, resulting in a marked reduction in all tested agronomic traits, especially productive panicle number, filled grain number, and heading date (Fig. 1D; Supplemental Fig. S1, A to D and Supplemental Table S1). These data indicate that cell death in spl38 is closely associated with growth- and yield-related traits in rice.
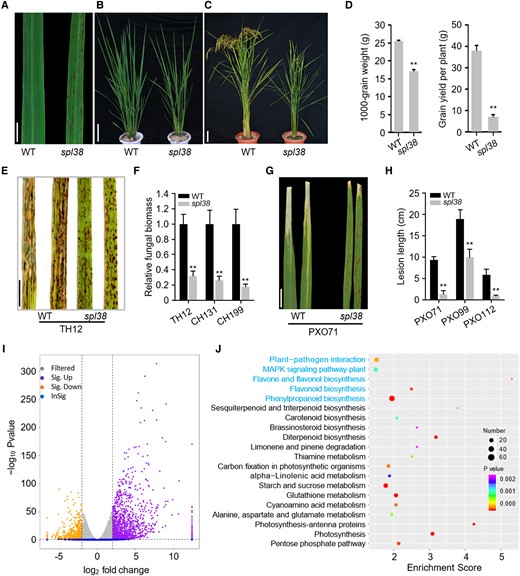
Phenotypic characterization of the LMM, spl38. A) Representative leaves of the spl38 mutant and the WT. Scale bar, 1 cm. B) Plant phenotype at the tillering stage (50 d after germination). Scale bar, 10 cm. C) Whole plant architecture at the ripening stage (120 d after germination). Scale bar, 10 cm. D) Comparison of 1,000-grain weight and average grain yield per plant between spl38 and WT plants grown under the natural paddy field conditions. E) Disease symptoms on spl38 and WT leaves inoculated with the compatible M. oryzae isolate TH12. Leaves were photographed at 120 hpi and are part of a single image. Scale bar, 1 cm. G) Disease symptoms on spl38 and WT leaves infected with the compatible X. oryzae pv. oryzae (Xoo) isolate PXO71. Leaves were examined at 14 dpi. Scale bar, 2 cm. F and H) Fungal biomass (F) and lesion length (H) on the inoculated leaves of spl38 and WT plants. I) Volcano plot showing the genes differentially expressed between the WT and spl38 mutant plants. The x-axis represents normalized RNA-seq log2 gene expression counts. Gray and blue colors represent nonsignificant DEGs; purple and orange colors represent significant DEGs (upregulated and downregulated, respectively). J) KEGG pathway enrichment analysis of top 20 KEGG DEGs (P ≤ 0.05). The x-axis represents the enrichment score. The color of the dot represents the P-value, and the size of the dot represents the number of DEGs mapped to the reference pathways. Data represent mean ± SE of 3 independent replicates. Asterisks represent significant differences between spl38 mutant and WT plants (**P < 0.01; Student's t-test).
To elucidate the possible biochemical mechanisms underlying the development of HR-like lesions in spl38, we investigated the levels of ROS, specifically and H2O2, using nitro blue tetrazolium (NBT) and 3,3′-diaminobenzidine (DAB) staining, respectively. The results of NBT staining strongly corresponded with lesion formation in the cleared spl38 mutant leaves but not in cleared WT leaves. Similar results were obtained with DAB staining (Supplemental Fig. S1, E and F). Moreover, the highest levels of ROS in spl38 were obtained at approximately 15 min after chitin application and were approximately 2- to 3.5-fold than those in the WT (Supplemental Fig. S1G), indicating that the ROS signaling pathway is triggered in spl38.
Spl38 exhibits enhanced resistance against bacterial and fungal pathogens
LMMs frequently exhibit enhanced pathogen resistance (Li et al. 2017). To evaluate the pathogen resistance ability of spl38, we inoculated the mutant and WT plants with 3 virulent isolates each of Magnaporthe oryzae (a causal agent of rice blast) and Xanthomonas oryzae pv. oryzae (Xoo; a causal agent of bacterial blight). In the spray inoculation assays of M. oryzae, both lesion area and fungal biomass were substantially lower in spl38 mutant plants than in the WT (Fig. 1, E and F; Supplemental Fig. S2A). Similar results were obtained when fully expanded flag leaves of the WT and spl38 inoculated with Xoo isolates were examined at 15-d postinoculation (dpi; Fig. 1, G and H; Supplemental Fig. S2B). Thus, spl38 plants displayed markedly increased resistance to bacterial and fungal rice pathogens.
To further understand the mechanism of enhanced resistance in spl38, we performed RNA sequencing (RNA-seq) analysis of WT and spl38 leaves at the early tillering stage (Supplemental Table S2). A total of 2,936 genes were upregulated, while 2,295 genes were downregulated in spl38 leaves compared with WT leaves (Fig. 1I; Supplemental Table S3). Gene Ontology (GO) analysis indicated that these differentially expressed genes (DEGs) enriched in the biological process category could be involved in photosystem stoichiometry adjustment, JA- and ethylene (ET)-dependent systemic resistance, and Ca2+ homeostasis (Supplemental Fig. S3). Kyoto Encyclopedia of Genes and Genomes (KEGG) pathway analysis revealed that DEGs representing distinct biological pathways were significantly enriched in plant–pathogen interaction, peroxisome, mitogen-activated protein kinase (MAPK) signaling pathway, and phenylpropanoid and flavonoid biosynthesis (Fig. 1J). Interestingly, we found that many chitinase and defense response-related genes were significantly upregulated in spl38. To verify the RNA-seq data, we confirmed the enhanced transcript levels of 6 defense-related genes involved in SA and JA signaling pathways and 7 of 8 chitinase-encoding genes in spl38 relative to that in WT by real-time quantitative reverse transcription PCR (RT-qPCR; Supplemental Fig. S2, C and D; Li et al. 2017). Moreover, transcription of 3 PR genes with similar expression patterns (PR1b, PR10, and AOS2) was induced upon M. oryzae infection in both the WT and spl38 mutant plants, but the induced expression of these genes was earlier and higher in spl38 than in the WT (Supplemental Fig. S2E). Together, these results suggest that spl38 confers enhanced resistance, possibly by activating the chitinase-related defense response in rice.
Positional cloning and expression analysis of the SPL38 gene
To isolate the causal gene underlying the lesion mimic phenotype of spl38, we employed a map-based cloning strategy. Genetic analysis indicated that the spl38 phenotype was governed by a single nuclear recessive gene (Supplemental Table S4). SPL38 locus showed linkage with 2 insertion/deletion (InDel) markers, IN28 and IN8, on the long arm of chromosome 10 and cosegregated with the spl38 phenotype of 90 recessive F2 individuals. To fine map the SPL38 locus, approximately 1,652 homozygous genotypes showing the spl38 phenotype were selected from the segregating F2 population, and the SPL38 locus was further delimited to a 40.9-kb region flanked by the sequence-tagged site (STS) markers M8 and M9 (Fig. 2A).
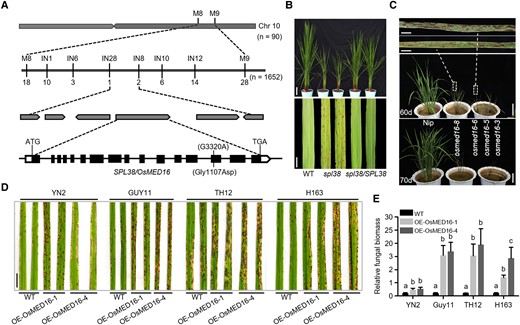
Positional cloning and functional characterization of SPL38. A) Schematic representation showing the location of SPL38/OSMED16 on rice chromosome 10. The SPL38 locus was preliminarily mapped to the long arm of chromosome 10 and then narrowed down to a 40.9-kb interval. The numbers under the linkage map indicate the number of recombinants. The predicted SPL38 gene structure is shown. Black rectangles and lines indicate exons and introns, respectively. The start codon (ATG) and stop codon (TGA) are also indicated. B)SPL38 complementation assay in rice. Functional complementation of the SPL38 gene completely rescued plant appearance (upper panel) and restored leaf phenotype (lower panel) in the paddy field. Representative plants were photographed at the tillering stage. Scale bar: 10 cm in the upper panel, 1 cm in the lower panel. C) Phenotypes of OsMED16-edited lines (generated by CRISPR-Cas9) and WT plants. Photographs were taken at 60 d (upper panel) and 70 d (lower panel) after transplanting. Scale bar: 5 mm in enlarged photographs and 10 cm in photographs of 60 and 70 d. D) Disease symptoms on WT and OsMED16-overexpressing leaves inoculated with 4 compatible M. oryzae isolates. Leaves were photographed at 120 hpi and are part of a single image. Scale bar, 1 cm. E) Fungal biomass on the inoculated leaves of OsMED16-overexpressing and WT plants. Data were normalized relative to the expression of OsACTIN1 and M. oryzae 28S genes. Data represent mean ± SE of 3 independent replicates. Different letters indicate statistically significant differences (P < 0.01), as determined by Duncan's multiple range test.
To examine the spl38 mutation, 6 open reading frames (ORFs) within the 40.9-kb region were amplified from the genomic DNA of spl38 and WT plants by PCR (Supplemental Table S5). Sequencing of these ORFs in both genotypes uncovered a point mutation (G → A) at 3,320 nt (relative to the transcription start site) in the 14th exon of OsMED16 (LOC_Os10g35560) in spl38, which resulted in an amino acid substitution from Gly to Asp. The 3,906 bp coding sequence (CDS) of OsMED16 contains 16 exons and is predicted to encode OsMED16 (Fig. 2A). Intriguingly, we found that not only the substituted amino acid residue (Gly) but also the full-length amino acid sequence of OsMED16 was highly conserved among dicots and monocots, with 66.2% sequence similarity between OsMED16 and AtMED16 (Supplemental Fig. S4, A and B).
To confirm whether the point mutation identified in OsMED16 corresponded to the spl38 phenotype, we performed the genetic complementation assay and CRISPR/Cas9-targeted mutagenesis. All transgenic lines harboring the full-length CDS of OsMED16 showed complete rescue of lesion mimic phenotype; no lesions were observed on the leaves of the spl38 complementation line (spl38/SPL38; Fig. 2B; Supplemental Fig. S5A). Four OsMED16-edited plants were confirmed by PCR-based sequencing. Three of these 4 plants were heterozygous; only osmed16-5 was homozygous. As expected, osmed16 mutant plants exhibited the lesion mimic phenotype, but these plants were extremely weak and exhibited dwarfism during early vegetative development, and eventually died after 70 d of growth in soil. Additionally, the osmed16-5 homozygous plant showed more severe phenotypes and earlier death than the 3 heterozygous plants (Fig. 2C; Supplemental Fig. S5, B and C).
To further elucidate the function of OsMED16 in rice, the spatiotemporal expression pattern of OsMED16 was investigated by RT-qPCR. OsMED16 was ubiquitously expressed in all plant organs tested, with the highest expression level in roots, and relatively low expression in the stem (Supplemental Fig. S6A). These results were confirmed by GUS staining assays using OsMED16::GUS transgenic plants generated in this study (Supplemental Fig. S6B). Together, these data suggest that OsMED16 is universally expressed in all organs of rice plants.
It was previously reported that AtMED16 regulates SA-triggered immunity in Arabidopsis (Zhang et al. 2012). To test whether OsMED16 is involved in the immune response regulation in rice, transcript levels of OsMED16 were investigated in WT plants treated with PAMP (chitin) and M. oryzae. The results showed that OsMED16 expression was substantially induced after chitin treatment, with maximal expression at 1-h postinoculation (hpi; Supplemental Fig. S6C). In addition, transcript levels of OsMED16 were also upregulated upon M. oryzae infection (Supplemental Fig. S6C). Moreover, we also confirmed the susceptibility of OsMED16-overexpressing plants; 2 OsMED16-overexpressing plants showed a drastic enhancement of susceptibility when challenged with 4 compatible M. oryzae isolates (Fig. 2, D and E). Consistently, 5 of 7 Chitinase genes were markedly downregulated in OsMED16-overexpressing plants (Supplemental Fig. S6E), suggesting that OsMED16 represses the expression of Chitinase genes. Together, these experiments strongly suggest a negative role of OsMED16 in regulating rice immunity.
OsMED16 interacts with OsPR3 in vitro and in vivo
To understand how OsMED16 regulates rice immunity, we first examined the subcellular localization of OsMED16. Full-length CDSs of OsMED16 and OsMED16M (which encodes a mutant protein with Gly1107Asp substitution) were fused to the N-terminus of the yellow fluorescent protein (YFP) gene. The recombinant plasmids were transiently expressed in rice protoplasts. The fluorescence signal of the OsMED16-YFP fusion protein was detected in the nucleus, whereas that of OsMED16M-YFP was detected in the cytosol and nucleus of rice protoplasts. By contrast, the YFP (positive control) signal showed ubiquitous distribution (Fig. 3A). These results indicate that mutations in OsMED16 affect the localization of the encoded protein.
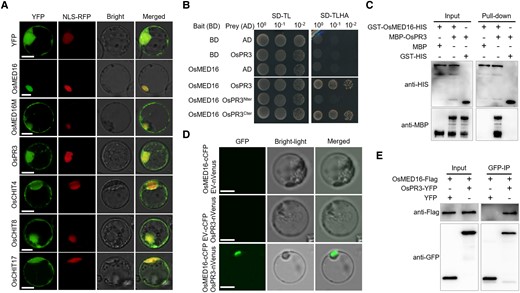
OsMED16 physically interacts with OsPR3. A) Subcellular localization of OsMED16, OsMED16M, OsPR3, and 3 chitinases in rice mesophyll protoplasts. The 35S::YFP (yellow fluorescent protein) protein was used as a positive control. NLS-RFP (red fluorescent protein) was a nucleus marker. Scale bars, 10 μm. B) Y2H assay showing the interaction between OsMED16 and OsPR3. The yeast strain AH109 was transformed and selected on minimal medium. -TL and -TLHA indicate SD/-Trp-Leu and SD/-Trp-Leu-His-Ade dropout plates, respectively. The ability of yeast colonies to grow on -TLHA plates indicates an interaction between the 2 proteins. C) Pull-down assay. GST-OsMED16-HIS and MBP–OsPR3 were expressed in E. coli. Co-precipitation of OsMED16 and OsPR3 was determined by western blotting before (input) and after affinity purification (pull-down) using glutathione agarose beads. D) BiFC assay verifying the interaction between OsMED16 and OsPR3 in rice protoplasts. Fluorescence was detected by confocal microscopy at 16 hpi. Scale bars, 20 μm. E) Co-IP assay confirming the OsMED16–OsPR3 interaction. Total proteins were extracted from rice protoplasts expressing OsMED16-Flag and OsPR3-YFP constructs. The immune complexes were pulled down using anti-GFP magnetic beads, and the co-precipitation of OsPR3-YFP or YFP was examined by western blotting. The experiment was repeated twice with similar results.
We next performed a yeast two-hybrid (Y2H) assay. Full-length OsMED16 CDS was used to identify potential interacting partners in a leaf cDNA library of M. oryzae-inoculated rice plants. Interestingly, the positive clone harboring the CDS of OsPR3 (LOC_Os06g51050), which encodes a chitinase precursor protein, was detected repeatedly (Fig. 3B).
To verify the OsMED16–OsPR3 interaction and its biological relevance, we carried out in vitro pull-down assays by expressing glutathione S-transferase (GST) fused to HIS-tagged OsMED16 and maltose-binding protein (MBP)-tagged OsPR3 in Escherichia coli. Immunoblot assays showed that MBP-OsPR3 was specifically enriched in the GST-OsMED16-HIS-bound glutathione resins (Fig. 3C). Next, we confirmed the interaction between OsMED16 with OsPR3 using bimolecular fluorescence complementation (BiFC) assay in rice protoplasts. A plasmid containing OsMED16 fused to the N-terminal half of Venus (OsMED16-nVenus) was co-expressed with the OsPR3-cCFP fusion in rice protoplasts. Strong fluorescence restricted to the nucleus was observed (Fig. 3D), suggesting that the OsMED16–OSPR3 complex localizes to the nucleus. We further validated the OsMED16–OsPR3 interaction in vivo using co-immunoprecipitation (Co-IP) assays. The OsMED16-FLAG and OSPR3-YFP fusions driven by the cauliflower mosaic virus (CaMV) 35S promoter were co-expressed in rice protoplasts. Total proteins were extracted and incubated with anti-GFP resin. The results showed that OsPR3-YFP, but not YFP, was considerably enriched in the OsMED16-YFP precipitates (Fig. 3E). Collectively, these results strongly suggested that OsMED16 associates with OsPR3 in vitro and in vivo.
The OsPR3 protein is predicted to contain N-terminal signal peptides, a chitin-binding domain, and a C-terminal catalytic domain. To identify the region of OsPR3 that binds to OsMED16, we performed the Y2H assay using 2 truncated fragments of OsPR3 (OsPR3Nter and OsPR3Cter). Dissection of their interactive domains indicated that the chitinase activity domain (OsPR3Nter), not the chitin-binding domain (OsPR3Cter), of OsPR3 is essential for binding to OsMED16 (Fig. 3B). Because rice chitinases are classified into 7 classes (Xu et al. 2007), we investigated the interaction between OsMED16 and 14 other rice chitinases. Y2H assays indicated that besides OsPR3, OsMED16 interacts with only CHIT4 in Class I, and no other chitinases (Supplemental Fig. S7). Intriguingly, the subcellular localization assay indicated that 4 YFP-fused chitinases showed similar localization patterns, with detection mainly in the cytoplasm and nucleus (Fig. 3A).
OsPR3 is a positive regulator of rice innate immunity
To elucidate the regulatory role of OsPR3 in rice immunity, we generated 2 OsPR3-edited plants (ospr3-4 and ospr3-6) in the japonica cultivar Zhonghua 11 (ZH11) using the CRISPR-Cas9 technology (Fig. 4A; Supplemental Fig. S8). Both OsPR3-edited plants showed markedly reduced transcript levels of OsPR3 compared with WT ZH11 plants but no phenotypic abnormalities (Fig. 4B). The OsPR3-edited plants were then challenged with 6 M. oryzae isolates. Compared with WT ZH11 plants, the OsPR3-edited plants showed remarkably reduced resistance, with increased fungal biomass (Fig. 4, C and D; Supplemental Fig. S9). Similar results were obtained when inoculated with 2 Xoo isolates; OsPR3-edited plants showed enhanced susceptibility to both isolates, with larger lesions in Xoo-inoculated OsPR3-edited plants compared with that in Xoo-inoculated ZH11 plants (Fig. 4, E and F; Supplemental Fig. S9A). Moreover, many defense-associated genes were downregulated in OsPR3-edited plants (Supplemental Fig. S9B) in comparison with WT ZH11 plants. Overall, these results indicate that OsPR3 positively regulates immune responses in rice.
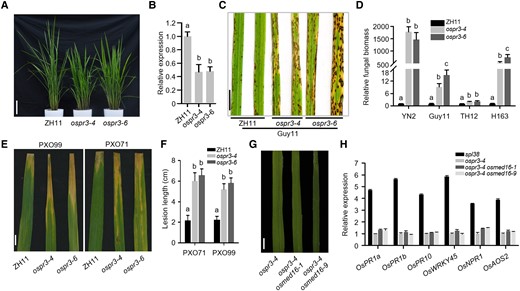
OsPR3 acts as a positive regulator of rice immunity. A) Phenotypes of OsPR3-edited lines (generated by CRISPR-Cas9) and WT plants. Photographs were taken at 50 d after germination. Scale bar, 10 cm. B) Transcript abundance of OsPR3 in OsPR3-edited lines and WT plants determined by RT-qPCR. OsACTIN1 was used as the internal standard. C) Disease symptoms on OsPR3-edited and WT leaves inoculated with a compatible M. oryzae isolate, Guy11. Leaves were photographed at 120 hpi and are part of a single image. Scale bar, 1 cm. D) Fungal biomass on the inoculated leaves of OsPR3-edited and WT plants. E) Disease symptoms on OsPR3-edited and WT leaves inoculated with 2 compatible Xoo isolates, PXO99 and PXO71. Leaves were examined at 14 dpi. Scale bar, 1 cm. F) Length of lesions formed on the inoculated leaves of OsPR3-edited and WT plants. G) Phenotypic analysis of the leaves of the ospr3-4 mutant and 2 ospr3-4 osmed16 double mutant lines. No lesions were visible on the leaves. Scale bar, 1 cm. H) Relative expression levels of 6 PR genes in single and double mutant plants by RT-qPCR. Total RNA was extracted from the leaves of 40-d-old rice seedlings. Data were normalized relative to the expression of the OsACTIN1 gene. Data represent mean ± SE of 3 independent replicates. Letters in B, D, and F represent statistically significant differences (P < 0.01; Duncan's multiple range test). The experiment was repeated twice with similar results.
To determine whether the lesion mimic phenotype of the spl38 mutant resulted from an increased accumulation of OsPR3, we mutated the OsMED16 gene in the ospr3-4 mutant background using the CRISPR/Cas9 technology and generated 2 ospr3-4/osmed16 double mutant lines (ospr3-4 osmed16-1 and ospr3-4 osmed16-9; Supplemental Fig. S10A). Two OsMED16-edited double mutants were confirmed by PCR-based sequencing (Supplemental Fig. S10B). Phenotypic analysis revealed that both double mutant lines were dwarf and highly sterile, and the OsMED6 mutations did not generate the lesion mimic phenotype in the ospr3-4 mutant (Fig. 4G; Supplemental Fig. S10C). Furthermore, the expression of 6 PR genes was repressed in ospr3-4 osmed16-1 and ospr3-4 osmed16-9 double mutants, unlike in spl38 (Fig. 4H). Collectively, these results suggest that OsMED16 negatively regulates cell death in an OsPR3-dependent manner.
OsMED16 represses the chitin-binding and catalytic activities of OsPR3 in rice
Given that OsPR3 is a putative GH19 chitinase (Fig. 5A), we purified OsPR3 and its truncated or mutated derivatives, and investigated their enzymatic activity using colloidal chitin as the substrate. The recombinant MBP-OsPR3 protein could effectively hydrolyze chitin into N-Acetyl-D-Glucosamine (GlcNAc) monomers (Fig. 5B), indicating that OsPR3 is an active chitinase. Amino acid sequence alignment showed that Glu141 and Glu163 residues are conserved among GH19 family chitinases, consistent with their requirement for chitinase activity reported previously (Balu et al. 2020). Amino acid substitutions (E141A and E163A) in OsPR3 (OsPR3E141A/E163A) abolished its catalytic activity (Fig. 5B). Intriguingly, the chitinase activity was dramatically inhibited in the presence of OsMED16, but not OsMED16M, indicating that OsMED16 hinders the enzymatic activity of OsPR3.
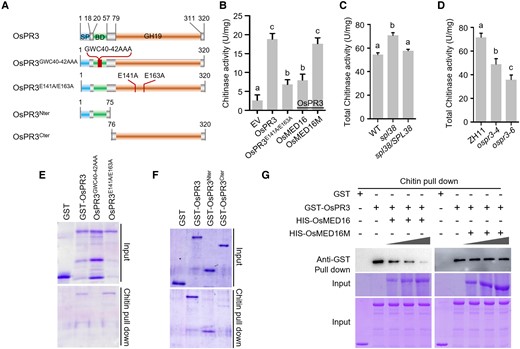
OsMED16 represses the chitin-binding and catalytic activity of OsPR3. A) Schematic representation of domain architecture of OsPR3 and its mutant proteins. SP, signal peptide; BD, chitin-binding domain; GH19, glycoside hydrolase 19. B) OsPR3 is a functional chitinase. The enzymatic activities of OsPR3 were inhibited by OsMED16 but not by OsMED16M. C) Chitinase activity in spl38, spl38/SPL38, and WT plants. D) Chitinase activity in OsPR3-edited lines and WT plants. The chitinase enzyme activity of the recombinant MBP-OsPR3 protein was analyzed using colloid chitin as the substrate. Data represent mean ± standard deviation (SD; n = 3). Letters represent statistically significant differences (P < 0.01; Duncan's multiple range test). Data represent mean ± SE (n = 3). E–G) Chitin pull-down assays showing that chitinase activity is dispensable for binding to chitin (E); the N-terminal region of OsPR3 is essential for binding to chitin (F); and OsMED16, but not mutated OsMED16, competes with chitin to bind to OsPR3 (G). Colloid chitin and purified recombinant proteins were used for chitin pull-down assays. Each 50-mL reaction contained 50 mg of colloid chitin and 2 mg of GST-OsPR3. GST-OsMED16 or mutated OsMED16 protein (0, 1, 2, and 3 μg) was added to the mixture, and the reaction was incubated for 1 h with constant shaking. The chitin-associated MBP-OsPR3 fusion protein was detected using immunoblotting. The experiment was repeated in triplicate with similar results.
These results prompted us to compare the chitinase activity of the protein extracts of WT, spl38, and spl38/SPL38 plants. Enzymatic activity assays revealed that the spl38 mutant showed markedly higher catalytic activity than the WT, whereas the spl38/SPL38 complementation line exhibited similar catalytic activity as the WT (Fig. 5C). Similar results were obtained using OsPR3-edited lines; the catalytic activity of the protein extract of OsPR3-edited lines was significantly lower than that of WT ZH11 plants (Fig. 5D), suggesting that the expression of OsMED16 and OsPR3 is closely associated with chitinase activity.
Given that OSPR3 contains a chitin-binding domain, we examined the chitin-binding property of OsPR3 by in vitro chitin pull-down assays. The results showed that OsPR3, OsPR3E141A/E163A, and OsPR3Nter (which contained an intact chitin-binding motif), but not OsPR3GWC40–42AAA (which lost the chitin-binding activity) and OsPR3Cter (which had an intact catalytic motif), were able to bind chitin in vitro (Fig. 5, E and F), indicating that the chitin-binding activity of OsPR3 is irrelevant to its catalytic activity.
Since OsMED16 can bind to OsPR3, we examined whether it competes with chitin to bind to OsPR3. The results of the chitin pull-down assay showed that the enrichment of OsPR3 decreased with the addition of increasing amounts of OsMED16, whereas the enrichment of OsPR3 did not alter followed by the increasing amounts of OsMED16M (Fig. 5G). Taken together, our results suggest that OsMED16 competitively inhibits chitin to bind to OsPR3 in vitro.
MED16 putative orthologs showed opposite pathogen-response phenotypes in monocot and dicot plants
Previous studies revealed that AtMED16 (also known as AtSFR6) positively regulates the response to pathogen attacks in Arabidopsis (Wathugala et al. 2012; Zhang et al. 2012). This led us to test whether MED16 homologs in monocots perform a similar function. Phylogenetic analysis revealed 3 potential MED16 homologs in the wheat genome (Supplemental Fig. S4). We, therefore, knocked down the expression of all 3 TaMED16s in wheat via virus-induced gene silencing (VIGS) using the barley stripe mosaic virus (BSMV). Two constructs were designed to simultaneously target and silence the 3 TaMED16 homologs in wheat. Consistent with the results of the spl38 mutant obtained in rice, the TaMED16 knockdown lines of wheat exhibited enhanced resistance to Puccinia striiformis f. sp. tritici, with less uredinia and reduced fungal biomass compared with the WT (Fig. 6, A to D). This result indicates that monocot MED16 homologs (OsMED16 and TaMED16s) potentially perform a similar role in negatively regulating the immune response.
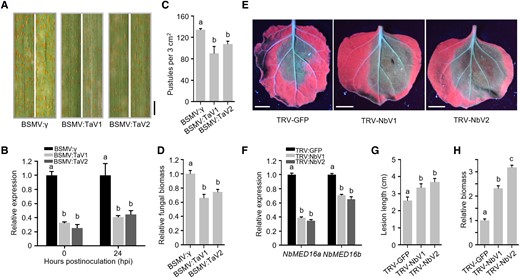
Wheat TaMED16s and N. benthamiana NbMED16s exhibit an opposite role in response to pathogen invasion. A) Disease symptoms on TaMED16-silenced leaves treated with P. striiformis f. sp. tritici (Pst). Leaves were photographed at 14 dpi. Scale bar, 3 mm. B) Transcript abundance of TaMED16 homologous genes in wheat determined by RT-qPCR analysis. C) The number of uredinium pustules formed by P. striiformis f. sp. tritici (Pst) on the fourth leaf of wheat plants treated with labeled BSMV. The number of pustules was counted within a 3.0 cm2 leaf area at 14 dpi. D) Relative fungal biomass on wheat leaves following Pst infection, as determined by RT-qPCR. TaEF-1α and PstEF-1α were used to normalize the RNA level of wheat leaves and Pst, respectively. E) Disease symptoms on NbMED16a/b-silenced leaves infected with P. parasitica. NbMED16a/b-silenced leaves were inoculated with 1,000-zoospore suspension, and disease symptoms were examined at 2 dpi. Scale bar, 1 cm. F) Analysis of the transcript abundance of NbMED16a/b in N. benthamiana leaves by RT-qPCR following the inoculation with Agrobacterium harboring either the TRV2–LIC empty VIGS vector (EV) or TRV2–LIC carrying the NbMED16a or NbMED16b silencing construct. The 18S rRNA was used as the internal standard. G) Size of lesions formed by P. parasitica on NbMED16a/b-silenced leaves. H) Quantification of the relative biomass of P. parasitica by RT-qPCR. The infected leaves (n = 15) were collected at 48 hpi. Data represent mean ± SE. Letters represent statistically significant differences (P < 0.01; Duncan's multiple range test). These experiments were repeated twice with similar results.
Next, we examined the function of MED16 in another dicot, Nicotiana benthamiana, using the VIGS assay. Phylogenetic analysis revealed 2 potential MED16 homologs (NbMED16a and NbMED16b) in N. benthamiana (Supplemental Fig. S4). Two DNA fragments designed to target both NbMED16a and NbMED16b were cloned into the tobacco rattle virus (TRV)-based VIGS vector to knock down their expression in N. benthamiana (Fig. 6E). Expression of these constructs in N. benthamiana successfully silenced both genes with high silencing efficiency (Fig. 6F). The NbMED16a and NbMED16b knockdown N. benthamiana lines showed reduced resistance to Phytophthora parasitica, with increased fungal biomass (Fig. 6, G and H), suggesting that the role of MED16 in positively contributing to plant immunity is conserved among dicots. Intriguingly, data showed that overexpression of OsMED16 in the atsfr6 mutant background was unable to rescue the susceptibility of the Arabidopsis mutant to Pst DC3000, and overexpression of AtMED16 in the spl38 mutant (OsMED16 mutation) background was unable to complement the lesion mimic phenotype in rice (Fig. 7). Taken together, these results suggest that MED16 genes of dicots and monocots may perform opposite roles in plant immunity against pathogens.
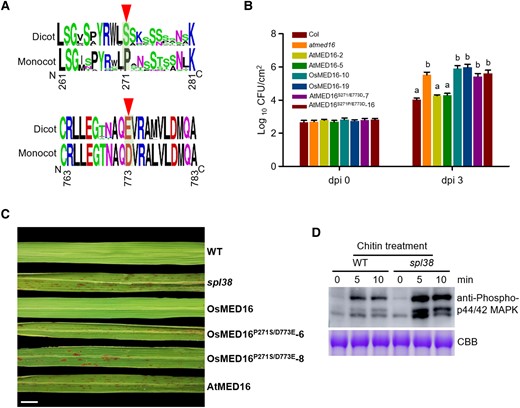
Two evolutionarily conserved residues of MED16 in 2 subclasses of angiosperms are important for pathogen response in Arabidopsis and rice. A) Frequency logos of partial amino acid sequences of MED16 in 33 different monocot and dicot species. The most prominent difference (PD signature in monocots vs. SE signature in dicots) is shown in Supplemental Fig. S4. PD signature: the proline (P) and aspartic acid (D) residues. SE signature: the serine (S) and glutamic acid (E) residues. B) Bacterial growth in atmed16, overexpressing 3 mutated AtMED16 transgenic lines. Bacterial titers were evaluated at 3 dpi. Data represent mean ± SE of 3 independent experiments, each consisting of 3 plants (n = 10). Letters represent statistically significant differences (P < 0.01; Duncan's multiple range test). These experiments were repeated twice with similar results. C) Leaf phenotypes of WT, spl38 mutant, and OsMED16M- or AtMED16M-overexpressing plants. The OsMED16M and AtMED16M cDNAs were introduced into spl38 plants, and T2 transgenic plants were generated. Leaves were collected and photographed at 60 d after germination. Scale bar, 1 cm. D) Chitin-induced MAPK activation in the leaves of 14-d-old WT and spl38 mutant seedlings. Leaves were treated with 10 μg mL−1 chitin. Samples were collected at 0, 5, and 10 min, and analyzed by immunoblotting with anti-phospho-p44/42 MAPK antibody. Bands indicate phosphorylated MPK3 and MPK6 proteins. Coomassie brilliant blue staining of Rubisco served as a loading control. The experiments were repeated 3 times with similar results.
Two evolutionarily conserved residues in monocot and dicot MED16 proteins play an important role in the pathogen response of Arabidopsis and rice
The above data indicated that MED16 proteins potentially harbor one or more evolutionarily conserved residues that contribute to the inverse pathogen response between monocots and dicots. To test this possibility, we further analyzed the amino acid sequences of 36 MED16s. The MED16s of monocots and dicots could be clearly distinguished based on 2 amino acid residues at positions 271 and 773: serine (S) and glutamic acid (E), respectively, in dicots such as N. benthamiana and Arabidopsis, and proline (P) and aspartic acid (D), respectively, in monocots such as wheat and rice (Fig. 7A; Supplemental Fig. S4), suggesting that these 2 amino acid residues are important for the antagonistic action of MED16 between monocots and dicots in response to pathogen attack. To verify this possibility, we performed a genetic complementation test in Arabidopsis by expressing the AtMED16S271P/E773D construct in the atsfr6 mutant background and challenging the resulting transgenic lines with Pst DC3000. The results showed that the expression of AtMED16S271P/E773D did not rescue the susceptibility of transgenic Arabidopsis plants to Pst DC3000 (Fig. 7B). Similar results were obtained, and transgenic plants expressing OsMED16P271S/D773E in the spl38 mutant were not able to eliminate the lesion mimic phenotype (Fig. 7C). Taken together, these genetic and molecular data indicate that the P and D residues at amino acid positions 271 and 773 likely determine the action of MED16 in response to pathogen infection.
To further explore whether the opposite actions of AtMED16 and OsMED16 are caused by only the difference in these 2 residues (S271P, E773D), we tested the commutative interaction between PR3 and MED16 in rice and Arabidopsis via 2 assays. The results showed that AtPR3 did not interact with both AtMED16 and OsMED16, and OsPR3 was unable to bind to AtMED16 in Y2H assays (Supplemental Fig. S11, A and B). We then generated 3 OsMED16 mutant sequences (OsMED16P271S, OsMED16D773E, and OsMED16P271S, D773E), in which the proline (P) and aspartic acid (D) residues of OsMED16 were individually or simultaneously substituted with the serine (S) and glutamic acid (E) of AtMED16, respectively. The results of protein–protein interaction analysis demonstrated that the substitution of amino acid residues in OsMED16 did not affect its interaction with OsPR3 in both Y2H and BiFC assays, suggesting that P and D residues are unessential for the interaction of OsMED16 with OsPR3. Moreover, we also determined the transcript levels of 8 other mediator subunits in spl38 and WT plants and found that their expression between spl38 and WT plants did not show substantial differences (Supplemental Fig. S11C), indicating that OsMED16-mediated defense phenotype is not likely associated with other mediator subunits. Given that OsMED16 interacts with OsPR3 in the nucleus (Fig. 3D), we explored whether OsMED16 regulates rice immunity via chitin signaling. The spl38 mutant and WT plants were treated with chitin, and their responses to chitin elicitation were examined. Compared with the WT, the spl38 mutant showed elevated chitin-triggered immune responses, as evident from the measurement of ROS levels and the immunoblot analysis of MAPKs with α-pMAPK antibody (Fig. 7D; Supplemental Fig. S1G). In summary, our results suggest that the nuclear-localized OsMED16 protein plays a negative role during chitin signaling, perhaps by dampening ROS production and MAPK activity.
Discussion
In this study, we characterized a rice mutant, spl38, which showed typical lesion mimic phenotypes, with elevated ROS accumulation and enhanced pathogen resistance (Fig. 1). SPL38 was identified as LOC_Os10g35560, which encodes mediator complex subunit 16, OsMED16. Functional complementation with the WT OsMED16 rescued the spl38 phenotype (Fig. 2B). We further showed that OsMED16-edited rice lines generated a similar mutant phenotype, but these lines also showed an extremely weak and dwarf phenotype and eventually died. It is likely that the site and type of mutations in OsMED16 in these plants led to the formation of truncated proteins, while 1 amino acid substitution was a weak mutation and resulted in cell death and growth retardation in the spl38 mutant. These data indicate that OsMED16 is an important gene for maintaining normal plant growth and development.
LMMs are often associated with early leaf senescence and damaged cellular organelles (Li et al. 2017; Ruan et al. 2019; Cui et al. 2020). For instance, mutation of OsSPL28, which encodes clathrin-associated adaptor protein complex 1 medium subunit μ1 (AP1M1), promotes leaf senescence in rice (Qiao et al. 2010). Similarly, a loss-of-function mutation in SPL29, which encodes UDP-N-acetylglucosamine pyrophosphorylase 1, exhibits degraded chloroplast membranes and abnormally assembled grana (Xiao et al. 2018). Nevertheless, spl38 did not exhibit early leaf senescence but showed retarded development and delayed flowering, similar to the osspl3 mutant, which displays delayed abscisic acid-mediated leaf senescence (Wang et al. 2015b).
Steady-state ROS levels in plants are determined by a tightly controlled balance between ROS production and scavenging. However, ROS equilibrium can be disturbed by different biotic and abiotic stresses, resulting in augmented ROS levels and consequently the destruction of cell structures (Mittler 2017). Interestingly, a number of studies have shown that elevated ROS levels trigger cell death and lesion formation in rice. For instance, mutation of the UDPG gene and disruption of the cytochrome P450 monooxygenase induce ROS production and cell death in rice (Xiao et al. 2018; Cui et al. 2020). Consistent with previous studies, massive amounts of and H2O2 were generated in spl38 leaves, potentially resulting in cell death and lesion formation.
LMMs in plants often originate as a result of a disturbance in PCD pathways, which induces the expression of PR genes and spontaneous appearance of HR-like lesions, both of which may contribute to enhanced disease resistance against pathogens (Yin et al. 2000; Manosalva et al. 2011; Chandrashekar et al. 2018; Ma et al. 2019). Consistently, in the present study, spl38 plants displayed markedly increased resistance to multiple M. oryzae and Xoo isolates, and showed a quick induction of 6 defense-related genes (Supplemental Fig. S2C), suggesting that the activated resistance was broad-spectrum and possibly nonspecific. Thus, the OsMED16 gene may be a promising target for genome editing to enhance rice disease resistance by improving other yield traits. Intriguingly, transcriptional analysis revealed that a large number of defense-related and chitinase genes are dramatically upregulated in spl38 compared with the WT. Plant chitinases are generated as PR proteins during self-defense against invading phytopathogens (Hamid et al. 2013). Consistent with the results of RNA-seq, RT-qPCR analysis showed that the expression of 7 chitinase and 6 PR genes was remarkably enhanced in spl38 compared with the WT (Supplemental Fig. S2). Moreover, we found that OsMED16 overexpression substantially enhanced the susceptibility of rice plants to virulent isolates of M. oryzae. Collectively, the loss- and gain-of-function assays conducted in this study strongly suggest that OsMED16 negatively regulates rice immunity. However, AtMED16 did not regulate cell death-related pathways, indicating its positive role in plant immunity. Mutations in AtMED16 blocked the induction of several JA/ET-responsive genes; consequently, the atmed16 mutants were more susceptible to necrotrophic fungal pathogens B. cinerea, Sclerotinia sclerotiorum, and A. brassicicola, and the bacterial P. syringae (Wathugala et al. 2012; Zhang et al. 2012; Wang et al. 2015a), indicating that MED16 homologs in rice and Arabidopsis play an antagonistic role in response to various pathogens.
In further seeking a mechanistic explanation for the OsMED16 ability in regulating rice immunity, we identified OsPR3 as an OsMED16-interacting protein in rice. OsPR3 encodes a chitinase precursor, which shares 64.8% amino acid sequence identity with AtPR3 (AT3G12500), indicating that OsPR3 is a potential PR protein (Chandrashekar et al. 2018). Our data showed that OsMED16 interacts with OsPR3 in the nucleus and negatively regulates rice immunity, probably via the chitin signaling pathway. By contrast, AtMED16 does not associate with AtPR3 and plays dual roles in mediating both SA and JA/ET signaling pathways as a positive immune regulator (Zhang et al. 2012). Recent studies showed that the B. cinerea effector Crh1 could be secreted into apoplastic spaces when Crh1 was fused to the AtPR3 secretion signal, indicating that AtPR3 is likely localized to the apoplastic space (Bi et al. 2021), while OsPR3 is mainly detected in nucleus and cytoplasm, which is reminiscent of the localization of 4 chitinases. Therefore, it is possible that rice and Arabidopsis MED16 proteins perform diverse functions in regulating plant immunity. However, we could not infer whether the mutated OsMED16-mediated plant immunity by OsPR3, in combination with other genes encoding antifungal products, or a direct activation chitin signaling, will improve resistance against pathogen infection, its exact mechanism remains to be explored. Moreover, we also found that OsMED16 associates with another rice Class I chitinase, CHIT4. Chitinases are widely distributed in prokaryotes and eukaryotes, and perform diverse roles (Hamid et al. 2013). For instance, chitinase secreted by the bacterial pathogen Xylella fastidiosa hydrolyzes chitin present in the cell wall and cuticle of its host fungi and insects, respectively, for use as a carbon source (Labroussaa et al. 2017). Chitinase of the fungal pathogen M. oryzae (MoChia1) was competitively bound by the rice tetratricopeptide repeat protein, resulting in the accumulation of free chitin for reestablishing the host immune response (Yang et al. 2019). Overexpression of genes encoding plant chitinases has been shown to effectively increase the resistance to Fusarium spp. in wheat, Rhizoctonia solani in rice, and B. cinerea in tomato (Cletus et al. 2013; Richa et al. 2017). Exogenous chitinases catalyze the hydrolysis of chitin present in the cell wall of fungi and green algae, and in the exoskeleton of many crustaceans and insects, and are well known for their antifungal activity (Santos et al. 2007). However, it remains unclear how plant endogenous chitinases, especially nuclear- and cytoplasm-localized chitinases, regulate immunity, and the precise molecular mechanism underlying these defense responses against pathogens needs to be addressed in the future.
The most exciting finding of this study was the identification of an SE signature in dicot and a DP signature in monocot MED16 amino acid sequences, which clearly differentiate the 2 evolutionary distinct plant lineages (Fig. 7). Analysis of the roles of these signature sequences in monocot and dicot MED16s using site-directed mutagenesis coupled with genetic complementation test revealed that P and D residues at amino acid positions 271 and 773 of MED16 are very important for plant immunity between monocots and dicots upon pathogen recognition, but not for the interaction of OsMED16 with OsPR3. MED is a multisubunit complex that functions as a transcriptional co-activator in all eukaryotes. In the present study, MED16, one of the MED subunits, was found in most sister lineage of land plants including mosses (Physcomitrium patens and Ceratodon purpureus) and liverwort (Marchantia polymorpha) but not in other lower land plants. The absence of MED16 in lower land plants might be compensated by the evolution of other subunits because MED complexes are variable at the evolutionary, compositional, and conformational levels, and missing subunits can be substituted by other subunits under different conditions (Allen and Taatjes 2015; Robinson et al. 2015). Intriguingly, in these 3 MED16s (PpMED16 in P. patens, CpMED16 in C. purpureus, and MpMED16 in M. polymorpha), we found that 2 amino acid residues (PD, TD, and SD, respectively) were reminiscent of the PD signature found in monocot MED16s. However, why and how other diverse residues at the corresponding amino acid positions of MED16 in lower land plants contribute to the evolutionary success of monocots remain to be investigated.
Taken together, it is plausible that the OsMED16 gene mutation is responsible for lesion mimic phenotypes, cell death, and enhanced disease resistance in rice. The nuclear-localized OsMED16 protein regulates rice immunity, probably by inhibiting chitin signaling. However, we could not rule out the possibility that the OsMED16 mutation results in the release of OsPR3, thereby being secreted into the apoplastic spaces. The precise mechanism of action of OsMED16 and OsPR3 is still elusive and warrants further investigation.
Materials and methods
Plant materials and growth conditions
The rice (O. sativa) mutant spl38 was originally isolated from an EMS-mutagenized population of the japonica rice cultivar TC65. To identify the gene responsible for the spl38 mutant phenotype, an F2 population was generated from the cross between spl38 and the indica rice cultivar Dular. Plants of all genotypes were grown at the experimental farms in Shanghai and Sanya (China) during the normal rice growing season. Two independent mutant lines of OsPR3 (ospr3-4 and ospr3-6) and 4 independent mutant lines of OsMED16 (osmed16-3, osmed16-5, osmed16-6, and osmed16-8) were generated via CRISPR/Cas9-mediated targeted mutagenesis in the background Zhonghua 11 “ZH11.” The ospr3-4 osmed16-1 and ospr3-4 osmed16-9 double mutant lines were generated via CRISPR/Cas9-mediated targeted mutagenesis in the background ospr3-4. To conduct pathogen infection assays, all plants were grown in a greenhouse under standard growth conditions at Shanghai Normal University. Arabidopsis (A. thaliana) and N. benthamiana plants were grown in a greenhouse at 22 °C under 16-h light/8-h dark photoperiod. Primers used in this study are listed in Supplemental Table S6.
Histochemical staining and ROS burst assays
The histochemical staining assays were performed using fresh leaves of spl38 and WT plants. NBT and DAB staining (for monitoring and H2O2 accumulation, respectively) and GUS staining (for monitoring tissue-specific expression) assays were performed as described previously (Yin et al. 2000; Qiao et al. 2010; Ma et al. 2019).
ROS production was determined as described previously (Liu et al. 2020). Briefly, leaf discs were excised from 5- to 7-wk-old plants using an ear-hole puncher and incubated overnight in sterile water using a 96-well plate. Then, 3 leaf discs were immersed in a tube containing either reaction buffer (100 μL of luminol [substrate; Thermo Scientific], 10 μg mL−1 peroxidase [Solarbio], and 10 μg mL−1 chitin) or sterile distilled water (control). The tube was immediately placed in a Promage GloMax Navigator Microplate Luminometer, and luminescence was recorded at 15 s intervals for 30 min.
Pathogen infection and biomass determination assays
To evaluate rice blast resistance, 2-wk-old spl38 and WT seedlings were spray-inoculated with the spore suspension (approximately 1 × 105 spores/mL) of 7 M. oryzae isolates (Deng et al. 2017), which was obtained by in vitro culture. The inoculated seedlings were incubated in a growth chamber at 28 °C in the dark for 24 h and then transferred to a greenhouse, where they were allowed to grow under normal growth conditions. Photographs of plants were taken at 7 dpi, and disease resistance was scored based on the fungal biomass determined by RT-qPCR (Kawano et al. 2010).
To evaluate bacterial blight resistance, 3 Xoo isolates were separately cultured on nutrient agar medium. The bacterial suspensions were adjusted to an optical density (OD600) of 1.0 using sterilized water. Fully expanded leaves of spl38 and WT plants at the booting stage were inoculated with the Xoo isolates using the scissors-dipping method, as previously reported (Yang et al. 2008). Lesion length was measured at 14 dpi.
Genetic mapping
To map the SPL38 gene, an F2 population was constructed by crossing spl38 with Dular, and recessive class analysis was performed using approximately 260 polymorphic simple sequence repeat and STS markers evenly distributed across all rice chromosomes. To further fine map the Spl38 gene, additional InDel and SNP markers were developed and used to narrow down the spl38-spanning region associated with the HR-like lesion phenotype of F2 plants (Qiao et al. 2010; Chen et al. 2020).
Plasmid construction and rice transformation
All plasmid constructs were generated via recombination using the ClonExpress II One Step Cloning Kit (Vazyme) or LR reaction using the Gateway LR Clonase II enzyme mix (Invitrogen). To generate the CRISPR/Cas9 knockout mutant lines, sgRNAs targeting the exon of OsMED16 and OsPR3 were designed using CRISPR-P and then cloned into the CRISPR plasmid BGK032 (Liu et al. 2017; Lu et al. 2017). To generate OsMED16 overexpression transgenic plants, the full-length CDS of OsMED16 was amplified and recombined into the pCAMBIA1390 vector, which placed the OsMED16 CDS fragment downstream of the maize (Z. mays) ubiquitin promoter. To generate OsMED16pro::GUS transgenic plants, the 2,000 bp promoter region upstream of OsMED16 was amplified and recombined into the pCAMBIA1391 vector. To conduct the complementation test, full-length OsMED16 CDS was cloned into the pCUbi1390 binary vector under the control of the maize Ubi promoter. The resulting constructs were introduced into A. tumefaciens strain EHA105, respectively, and the transformed Agrobacterium cells were used to transfect immature rice embryos, as described previously (Hiei and Komari 2008).
Protoplast isolation and transformation
For isolation of rice protoplasts, Nipponbare seeds were planted on 1/2 MS medium in darkness to generate 2-wk-old etiolated seedlings. The stem and sheath of etiolated rice seedlings were cut into ∼0.5 mm strips, and incubated in an enzyme solution containing 1.5% (w/v) Cellulase and 0.75% (w/v) Macerozyme R-10 for 4 to 5 h in the dark with gentle shaking (50 rpm) at 25 °C. The digested product was transferred to an equal volume of W5 solution (154 mM NaCl, 125 mM CaCl2, 5 mM KCl, and 2 mM MES at pH 5.7), and the protoplasts were collected by filtering through 70 μm nylon meshes. After centrifugation at 200×g for 10 min, the W5 solution was discarded and the protoplasts were resuspended in MMG solution (0.4 M mannitol, 15 mM MgCl2, and 4 mM MES at pH 5.7). Then rice protoplasts were transformed with the corresponding plasmids via polyethylene glycol (PEG)-mediated transformation as previously described (Yang et al. 2019).
Subcellular localization
Full-length CDS of OsMED16, OsMED16M, OsPR3, OsCHIT4, OsCHIT8, and OsCHIT17, without the stop codon, were individually amplified and cloned into the PA7 vector to generate C-terminal YFP fusions. An NLS-RFP fusion was used as a nuclear marker. The recombinant plasmids were introduced into rice protoplasts via PEG-mediated transformation, and the transformed protoplasts were incubated at 28 °C in the dark for 16 h, as previously described (Yang et al. 2019). The resulting construct was individually transformed into rice protoplast, and the fluorescence of YFP fusion proteins was detected at 16 to 18 hpi using a confocal microscope (Olympus Fluoview FV1000). YFP fluorescence was observed after excitation using a 488-nm laser and detected using the bandpass 505 to 530 nm emission filter setting. RFP was observed after excitation using a 561-nm laser and detected using the bandpass 605 to 630 nm emission filter setting.
Y2H assay
Y2H assay was carried out as previously described (Gui et al. 2022). Briefly, full-length OsMED16 CDS was cloned into the pGBKT7 bait vector for screening the rice cDNA library. The entire ORF of potential target genes was cloned into the pGADT7 prey vector. The resultant bait and prey constructs were co-transformed into yeast (Saccharomyces cerevisiae) strain AH109. The transformed cells were plated on SD/-Trp/-Leu medium and incubated at 30 °C for 2 d. Then, the cells were transferred to the stringent medium (SD/-Trp/-Leu/-His/-Ade; Zhang et al. 2019) and incubated at 30 °C for another 3 to 6 d before evaluation. The transformants that grew on the most stringent selective medium were considered to show protein–protein interactions.
GST and chitin pull-down assays
The CDSs of OsMED16 and OsPR3 were individually cloned into pGEX4T-2 and pMAL-c2x vectors to generate the GST-OsMED16 and MBP-OsPR3 constructs. These resulting constructs and empty vectors were expressed in E. coli strain Rosetta. The GST pull-down assay was carried out as described previously (Ma et al. 2019). Briefly, GST or GST-OsMED16 fusion protein coupled to beads was incubated with MBP or MBP-OsPR3. After washing the beads 3 to 5 times with 1× phosphate buffered salt solution, the co-precipitation of OsMED16 with OsPR3 was examined by western blotting using the corresponding antibodies.
The chitin pull-down assay was performed as described previously (Yang et al. 2019). Briefly, 2 mg of colloidal chitin was incubated with 2 μg of various purified recombinant proteins (GST-OsPR3, GST-OsPR3Nter, GST-OsPR3Cter, GST-OsPR3 GWC40–42AAA, or GST-OsPR3 E141A/E163A) separately in binding buffer (20 mM Tris-HCl [pH 7.5], 100 mM NaCl, 0.1 mM EDTA, 0.1 mM PMSF, and 0.1% (v/v) Triton X-100) at 4 °C for 3 h, with gentle shaking. The glycan was collected by centrifugation at 6,000×g at 4 °C for 2 min. The pellet was washed 5 times with washing buffer (20 mM Tris-HCl [pH 7.5], 200 mM NaCl, 0.1 mM EDTA, 0.1 mM PMSF, and 0.2% Nonidet P-40). The precipitate was separated by SDS-PAGE. Chitin-associated GST-tagged proteins were detected by immunoblotting using anti-GST antibody.
BiFC and Co-IP assays
To perform the BiFC assay, full-length cDNA sequences of OsMED16 and OsPR3 were cloned into pSAT1-cCFP and pSAT1-nVenus vectors, respectively. The recombinant plasmids were co-expressed in rice leaf sheath protoplasts by PEG-mediated transformation, and the transformed protoplasts were incubated at 28 °C in the dark for 16 h. The fluorescence signal of recombinant proteins was detected at 48 hpi using a confocal microscope (Olympus Fluoview FV1000). YFP fluorescence was observed after excitation using a 488-nm laser and detected using the bandpass 505 to 530 nm emission filter setting.
To perform the Co-IP assay in rice protoplast, PCR products were cloned into the PA7-Flag and PA7-YFP vectors to generate constructs (OsMED16-Flag and OsPR3-YFP). The PA7-YFP plasmid was used as a negative control. The recombinant plasmids were introduced into rice protoplasts via PEG-mediated transformation, and the transformed protoplasts were incubated at 28 °C in the dark for 16 h, as previously described (Yang et al. 2019). Total proteins were extracted from the transformed protoplasts using the extraction buffer (10 mM HEPES, 100 mM NaCl, 1 mM EDTA, 2 mM NaF, 2 mM Na3VO4, 1 mM DTT, 0.5% (v/v) Nonidet P-40, 1% (v/v) protease inhibitor cocktail [Sigma-Aldrich]), and then incubated with Anti-GFP mAb Magnetic Beads (MBL) at 4 °C for 4 h. The co-precipitate of OsMED16 was analyzed using anti-Flag or anti-GFP antibody.
Chitinase activity assay
Chitinase activity was determined using colloidal chitin as the substrate, according to the protocol described previously (Yang et al. 2019). Briefly, 0.25 mg of colloidal chitin was incubated with 1 μg of the recombinant protein in 100 μL of 50 mM sodium acetate buffer (pH 7.0) at 37 °C for 1 h. The reaction was terminated by boiling the sample in water at 100 °C for 5 min. The undigested chitin was removed by centrifugation and then added 100 μL of 3,5-dinitrosalicylic acid (DNS) to the supernatant. The absorbance of the supernatant was measured at 540 nm using a spectrophotometer (Eppendorf). The GST protein was used as a negative control. One unit (U) of chitinase was defined as the amount of enzyme required to produce 1 μM N-acetylglucosamine per mg chitin per hour under the standard conditions described above.
MAPK assay
Leaves and stems of O. sativa seedlings were cut to 3-mm strips. The materials were floated in water overnight to recover from wounding stress. The materials were then treated with 10 μg mL−1 chitin for 15 min followed by freezing in liquid nitrogen. Materials were ground in liquid nitrogen and homogenized in extraction buffer containing 10 mM HEPES, 100 mM NaCl, 1 mM EDTA, 2 mM NaF, 2 mM Na3VO4, 1 mM DTT, 0.5% (v/v) Nonidet P-40, 1% (v/v) protease inhibitor cocktail (Sigma-Aldrich), 1× PhosStop phosphatase inhibitor cocktail (Roche). Phosphorylation of MAPK proteins was detected by western blot with anti-phospho-p44/42 MAPK antibody (Cell Signaling Technology, #4370s).
VIGS assays in wheat (Triticum aestivum) and N. benthamiana
To silence TaMED16s, 2 cDNA fragments derived from the CDS were amplified by PCR and cloned into the BSMV vector. The recombinant constructs and the empty vector (containing the tripartite viral genome) were linearized and transcribed to produce RNA, which was then used to inoculate the second leaf of wheat plants (BSMV-γ, BSMV-TaPDS, BSMV-TaMED16-1, and BSMV:TaMED16-2), as previously described (Xu et al. 2019). Plants infected with the BSMV were then grown at 25 to 27 °C. After 10 dpi, the fourth leaves of seedlings were further treated with fresh urediniospores of Pst CYR31 on Suwon 11 wheat cultivar. Then, 15 to 20 infected leaves were used to analyze the disease symptoms and to count the urediniospores. RNA was isolated from the infected leaves to assess the silencing efficiency. DNA was extracted from Pst-infected leaves to determine the fungal biomass. Finally, leaves showing disease symptoms were photographed at 14 dpi.
The OsMED16 homologous genes in N. benthamiana, NbMED16a and NbMED16b, were silenced using the antisense fragments, NbMED16i-1 and NbMED16i-2, respectively, with the TRV-based VIGS system, as described previously (Dong et al. 2007). The TRV-GFP vector was used as a negative control.
RNA isolation, RT-PCR, and RT-qPCR
Total RNA was extracted from various rice tissues (root, stem, leave, leaf sheath, and young panicle) or leaves treated with chitin or Xoo using Trizol reagent (Invitrogen) according to the manufacturer's procedures. Total RNA of 2 μg was used as a template to synthesize first strand cDNA in a 20-μL reaction volume based on the HiScript II 1st Strand cDNA Synthesis Kit (Vazyme). RT-qPCR was performed on a Roche LightCycler480 system as previously described (Zhang et al. 2019), and the ChamQ Universal SYBR qPCR Master Mix (Vazyme) was used to detect the tissue- or gene-specific expression. The rice Actin gene was used as an internal control.
RNA-seq analysis
RNA-seq libraries were constructed from the spl38 and WT leaves of 4-wk-old seedlings following the manufacturer's procedure. Deep sequencing was conducted on an Illumina HiSeqTM 2500 genome analyzer. Raw reads were assessed for quality control by the software NGS QC Toolkit. Clean reads were mapped to the Nipponbare cultivar reference genome by hisat2 with default parameters. The Fragments Per Kilobase of exon model per Million mapped fragments (FPKM) value was utilized to calculate the normalized expression data of each library. DEGs were determined by the DESeq R package with the criteria of absolute fold change > 2 and false discovery rate < 0.05. GO functional enrichment analysis and KEGG analysis were performed as described previously (Wang et al. 2017).
Protein expression and western blot analysis
For the prokaryotic system, the resulting constructs and empty vectors were expressed in E. coli strain Rosetta, and the induction and purification of fused proteins were carried out as previously described (Yang et al. 2019). Western blot analysis was performed as previously described (Zhang et al. 2019). In brief, proteins were separated by SDS-PAGE and transferred electrophoretically to polyvinylidene fluoride membranes. The membrane was then incubated with specific antibodies and envisioned using Supersignal Chemiluminescent substrates reagent (Thermo Fisher Scientific).
Phylogenetic analyses
The amino acid sequences of OsMED16 in various plants were downloaded by the NCBI Blastp search program. Sequence alignment was performed with Clustal Omega. The phylogenetic tree was generated using the Neighbor-Joining method in MEGA X. The bootstrap values indicated at the nodes in the phylogenetic tree are based on 1,000 bootstrap replications.
Statistical analysis
Statistical analyses for multiple comparisons were performed using a one-way ANOVA or two-tailed Student's t-test with SPSS 18.0.
Accession numbers
The raw data of RNA-seq are archived at NCBI PRJNA748105. Sequence data from this article can be found in the GenBank/EMBL libraries under the following accession numbers: OsMED16 (LOC_Os10g35560), OsPR3 (LOC_Os06g51050), AtMED16 (AT4G04920), AtPR3 (AT3G12500), OsCHIT1 (LOC_Os02g39330), OsCHIT2 (LOC_Os04g41620), OsCHIT3 (Os04g41680), OsCHIT4 (LOC_Os03g30470), OsCHIT6 (LOC_Os05g33150), OsCHIT8 (LOC_Os06g51060), OsCHIT9 (LOC_Os12g13610), OsCHIT10 (LOC_Os01g18400), OsCHIT11 (LOC_Os05g04690), OsCHIT12 (LOC_Os08g41100), OsCHIT13 (LOC_Os09g32080), OsCHIT14 (LOC_Os10g39680), OsCHIT17 (LOC_Os05g33130), OsCHITC1 (LOC_Os01g43220), OsCHITC2 (LOC_Os10g28050), OsPAL1 (LOC_Os02g41630), OsPAL4 (LOC_Os02g41680), OsPR1a (LOC_Os07g03710), OsPR1b (LOC_Os01g28450), OsPR10 (LOC_Os12g36880), OsWRKY45 (LOC_ Os05g25770), OsNPR1 (LOC_Os01g09800), OsAOS2 (LOC_Os03g12500), OsMED11 (LOC_Os02g09600), OsMED14 (LOC_Os09g10960), OsMED15 (LOC_Os08g45080), OsMED18 (LOC_Os02g10050), OsMED21 (LOC_Os08g04150), OsMED23 (LOC_Os02g49992), OsMED28 (LOC_Os05g06290), OsMED31 (LOC_Os07g07020), and OsActin (LOC_Os03g50885).
Acknowledgments
We thank Prof. Heather Knight (Durham University, UK) for providing seeds of sfr6 mutant, 35S::AtSFR6 and 35S::OsSFR6 transgenic lines, and Prof. Ertao Wang (Shanghai Institute of Plant Physiology & Ecology, CAS) for helpful discussions.
Author contributions
Y.Q. conceived and designed the experiments; P.Z., X.M., L.L., C.M., Y.H., B.Y., X.L., G.S.L., J.S., X.P., and J.G. performed the experiment; P.Z. and X.M. analyzed the data; Y.D., Z.Z., and Z.K. provided the suggestion for this research; Y.Q. and P.Z. wrote the manuscript. All authors discussed the results and commented on the manuscript.
Supplemental data
The following materials are available in the online version of this article.
Supplemental Figure S1. Phenotype of the lesion mimic mutant spl38.
Supplemental Figure S2. Analysis of pathogen resistance in the spl38 mutant (related to Fig. 2).
Supplemental Figure S3. KEGG pathway enrichment analysis of top 20 KEGG DEGs between WT and spl38 mutant based on transcriptome data (P ≤ 0.05).
Supplemental Figure S4. Phylogenetic analysis of MED16 homologs in 33 plants.
Supplemental Figure S5. Analysis of targeting sequences in OsMED16-complementation and edited rice plants.
Supplemental Figure S6. Analysis of expressions of OsMED16 and Chitinase genes.
Supplemental Figure S7. Y2H assay verifying interaction between OsMED16 and 15 chitinases, respectively.
Supplemental Figure S8. Characteristics of OsPR3-edited plants generated by CRISPR/Cas9.
Supplemental Figure S9. Analysis of OsPR3-edited lines by pathogen inoculation assay and RT-qPCR.
Supplemental Figure S10. Characteristics of ospr3/osmed double mutant plants generated by CRISPR/Cas9.
Supplemental Figure S11. Analysis of interaction between MED16 and PR3 in rice and Arabidopsis.
Supplemental Table S1. Comparison of agricultural traits between spl38 and the wild-type cultivar, Taichung 65.
Supplemental Table S2. Summary of RNA-seq read mapping results.
Supplemental Table S3. Transcriptome data of the wild type and spl38 mutant.
Supplemental Table S4. Genetic analysis of the spl38 mutant in F2 populations.
Supplemental Table S5. List of 6 predicted genes present in candidate region on chromosome 10.
Supplemental Table S6. List of primers used in this study.
Funding
This work was supported by grants from the National Natural Science Foundation of China (32072502, 31801321, and 32172359), the Science and Technology Commission of Shanghai Municipality (18DZ2260500), the Next-Generation BioGreen 21 Program (PJ015782), Rural Development Administration, Republic of Korea, and the Special Program of China National Tobacco Corporation (110202102034).
References
Author notes
Peng Zhang, Xiaoding Ma, Lina Liu and Chanjuan Mao contributed equally to this work.
The author responsible for distribution of materials integral to the findings presented in this article in accordance with the policy described in the Instructions for Authors (https://dbpia.nl.go.kr/plphys/pages/General-Instructions) is: Yongli Qiao.
Conflict of interest statement. The authors declare no potential conflict of interest.