-
PDF
- Split View
-
Views
-
Cite
Cite
Jianghai Xu, Zhijia Yang, Xiaohong Fei, Meiling Zhang, Yang Cui, Xiangbo Zhang, Kaiwen Tan, Lizhu E, Haiming Zhao, Jinsheng Lai, Qian Zhao, Weibin Song, HEAT SHOCK PROTEIN 90.6 interacts with carbon and nitrogen metabolism components during seed development, Plant Physiology, Volume 191, Issue 4, April 2023, Pages 2316–2333, https://doi.org/10.1093/plphys/kiad019
- Share Icon Share
Abstract
Carbon and nitrogen are the two main nutrients in maize (Zea mays L.) kernels, and kernel filling and metabolism determine seed formation and germination. However, the molecular mechanisms underlying the relationship between kernel filling and corresponding carbon and nitrogen metabolism remain largely unknown. Here, we found that HEAT SHOCK PROTEIN 90.6 (HSP90.6) is involved in both seed filling and the metabolism processes of carbon and nitrogen. A single-amino acid mutation within the HATPase_c domain of HSP90.6 led to small kernels. Transcriptome profiling showed that the expression of amino acid biosynthesis- and carbon metabolism-related genes was significantly downregulated in the hsp90.6 mutant. Further molecular evidence showed strong interactions between HSP90.6 and the 26S proteasome subunits REGULATORY PARTICLE NON-ATPASE6 (RPN6) and PROTEASOME BETA SUBUNITD2 (PBD2). The mutation of hsp90.6 significantly reduced the activity of the 26S proteasome, resulting in the accumulation of ubiquitinated proteins and defects in nitrogen recycling. Moreover, we verified that HSP90.6 is involved in carbon metabolism through interacting with the 14-3-3 protein GENERAL REGULATORY FACTOR14-4 (GF14-4). Collectively, our findings revealed that HSP90.6 is involved in seed filling and development by interacting with the components controlling carbon and nitrogen metabolism.
Introduction
Carbon (C) and nitrogen (N) are two essential macronutrients required for plant development and seed formation (Gao et al., 2020). C/N metabolism is inseparable from seed filling, and the seeds are the main harvest organs. Understanding the relationship between seed filling and C/N metabolism is helpful for cultivating high-quality and high-yield crops. In the past few years, the regulatory network of seed filling has been explored and many genes that control seed filling have been cloned, such as Choline Transporter-like Protein 1 (CTLP1), Opaque Endosperm and Small Germ 1 (OS1), and Miniature Seed 6 (MN6) (Song et al., 2019; Hu et al., 2021; Yi et al., 2021). However, the mechanism between seed filling and C/N metabolism remains largely unknown. It has been reported that some small-molecule heat shock proteins (sHSPs) are involved in C/N metabolism in the endosperm (Ishimaru et al., 2019), but it remains unclear whether members of the HEAT SHOCK PROTEIN 90 (HSP90) subfamily are also involved in this process.
The HSP90 subfamily is a highly conserved chaperone protein subfamily (Schopf et al., 2017), belonging to the gyrases, HSP90, histidine kinase and MutL (GHKL) superfamily of ATPases (Dutta and Inouye, 2000). HSP90s contain three domains: an N-terminal ATPase domain responsible for hydrolyzing ATP to release energy, an intermediate domain responsible for client protein binding, and a C-terminal dimerization domain (Pearl and Prodromou, 2006; Prodromou, 2016). HSP90s play important roles in stress-treated tissues, including cell signal transduction, the cell cycle, and maintaining protein integrity (Picard et al., 1990; Pratt, 1997; Young et al., 2003; Jackson et al., 2004; McClellan et al., 2005; Taipale et al., 2010). HSP90s have also been found to be important parts of the plant development process under normal physiological conditions (Rutherford and Lindquist, 1998; Krishna and Gloor, 2001); a loss-function of HSP90 affects embryogenesis, seed germination, and pollen development (Prasinos, 2005; Cha et al., 2013; Feng et al., 2014; Luo et al., 2019).
Generally, HSPs function as molecular chaperones by interacting with multiple types of proteins (Prodromou, 2016; Hoter et al., 2018). HSP90 and HSP70 could interact with the 26S proteasome in yeast and participate in the degradation of ubiquitinated proteins (Verma et al., 2000; Park et al., 2007). In addition, ScHSP110 interacts with the 19S regulatory particles (RPs) of the 26S proteasome and then recruits the substrate-HSP70 complex to participate in protein degradation (Kandasamy and Andréasson, 2018). The 26S proteasome is the key complex responsible for regulating protein degradation in eukaryotic cells and controlling many cellular processes, including the cell cycle, DNA replication, and signal transduction (Goldberg, 2007; Finley, 2009; Bhattacharyya et al., 2014; Collins and Goldberg, 2017). The 26S proteasomal degradation pathway is a recycler of proteins and thus a contributor to N recycling (Tornkvist et al., 2019). Research shows that proteasome inhibition could lead to amino acid shortages. In yeast (Saccharomyces cerevisiae), human (Homo sapiens), and Drosophila (Drosophila melanogaster), the deleterious consequences of proteasome inhibition can be rescued by extra amino acid supplementation (Suraweera et al., 2012).
In addition to being molecular chaperones, HSPs also play regulatory roles through posttranslational modification, including phosphorylation, acetylation, and methylation (Prodromou, 2016; Sima and Richter, 2018). Phosphorylation is the most common posttranslational modification of HSPs involved in regulation (Hornbeck et al., 2012; Muller et al., 2013; Nguyen et al., 2017). It has been reported that the 14-3-3 protein BRAIN MODULOSIGNALIN HOMOLOGUE 1 (Bmh1) recruits the phosphatase GLYCOGEN SYNTHASE 7 (Glc7) by interacting with HSP70 to participate in the regulation of sugar metabolism in Saccharomyces cerevisiae (Hübscher et al., 2016; Chen et al., 2019). And 14-3-3 proteins are highly conserved dimeric proteins that bind to Ser/Thr phosphorylated sites on substrate proteins (Morrison, 2009; Madeira et al., 2015). In Arabidopsis (Arabidopsis thaliana) and maize (Zea mays L.), there are 13 and 12 14-3-3 proteins, respectively. The 14-3-3 isoforms chi and epsilon in Arabidopsis (Swatek et al., 2011) and GF14-4 and GF14-6 in maize are substantially expressed in the seeds (Dou et al., 2015). Protein–protein interactions between the 14-3-3 proteins and substrate proteins show that the 14-3-3 proteins are involved in glycolysis, fatty acid synthesis, and protein storage (Swatek et al., 2011; Dou et al., 2015; Ma et al., 2016). This is in line with the findings of previous studies based on several specific targets of 14-3-3s: 14-3-3s mainly regulate C/N metabolism in plants (Fu et al., 2000; Fulgosi et al., 2002; Comparot et al., 2003; Diaz et al., 2011).
Here, we cloned HSP90.6 and proved that its product is involved in maize seed filling via carbon and nitrogen metabolism. Evidence showed that a mutation in the HATPase_c domain of HSP90.6 resulted in lower ATPase activity in the hsp90.6 mutant, which ultimately led to smaller seeds. We also demonstrated that HSP90.6 is involved in the 26S proteasome degradation pathway, which affects nitrogen recycling. Furthermore, HSP90.6 was found to be involved in carbon metabolism by interacting with the 14-3-3 protein GF14-4.
Results
Cloning and identification of HSP90.6
To identify genes involved in seed filling and development in maize, ethyl methanesulfonate (EMS) was used to induce mutations. And a mutant with abnormal kernel development, ehsp90.6, was isolated by bulked segregant RNA-sequencing (BSR-seq). A single-nucleotide mutation (G380A) was identified, which resulted in a single-amino acid change from Arg to Gln (Figure 1D; Supplemental Table 1). Compared with wild-type (WT) seeds, mature seeds of ehsp90.6 were smaller and slightly shrunken (Figure 1, A and B; Supplemental Figure 1, A–C). ehsp90.6 could germinate normally, but the seedlings showed dwarfism (Figure 1C; Supplemental Figure 1, D and E). The segregation ratio of normal seeds and smaller seeds was approximately 3:1, indicating that the mutation in ehsp90.6 is a single recessive gene. To further determine the candidate gene, three independent knockout plants were obtained, all of which were heterozygous mutants with an insertion or deletion at the target site leading to frameshift mutations (Supplemental Figure 2A). When T1-generation heterozygous hsp90.6 plants were selfed, some seeds on the mature ears showed obvious shrinkage, almost no filling, and could not germinate (Supplemental Figure 2B); the segregation ratios were close to 3:1 (246:74, 352:127, 298:112). The progeny resulting from the hybridization of plants with the different transformation plants also showed the same results (Supplemental Figure 2D).
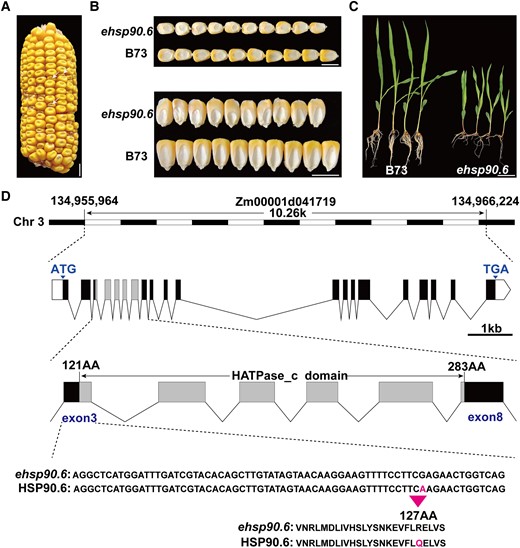
Cloning and phenotypic characteristics of ehsp90.6. A, Ears of heterozygous ehsp90.6 F2 mutants; the white arrow represents homozygous ehsp90.6. Scale bar = 1 cm. B, Mature seeds of B73 and ehsp90.6. Scale bar = 1 cm. C, Seedlings of B73 and ehsp90.6 at 14 day after germination (DAG). Scale bar = 5 cm. D, Genomic location, structure, and mutation site of ehsp90.6. ehsp90.6, EMS mutant of hsp90.6.
The hsp90.6 mutant could be distinguished from the WT as early as 10 days after pollination (DAP), and the seeds were light yellow (WT) and light white (hsp90.6) (Supplemental Figure 2C). The WT and hsp90.6 grains were separated from the same ear for paraffin embedding. The results showed that embryo and endosperm development occurred significantly later in hsp90.6 seeds than in WT seeds, and subsequent seed filling was significantly hindered in hsp90.6 (Supplemental Figure 2E). Therefore, HSP90.6 is involved in the regulation of maize seed development, as well as seed filling.
HSP90.6 is a heat shock protein located in the cytoplasm and nucleus
HSP90.6 is a member of the HSP90 subfamily and comprises 831 amino acids, with an HATPase_c domain at amino acids 121–283 (Figure 1D). RT-qPCR analysis showed that HSP90.6 was expressed in the roots, stems, leaves, tassels, and female ears. The transcript level of HSP90.6 was highest in the early stage of grain development (Supplemental Figure 3B). Then, the expression of HSP90.6 in kernel subregions was detected by in situ hybridization of grains at different stages. HSP90.6 was highly and evenly distributed in kernels at 5 DAP. The expression in the embryo and endosperm was still strong at 12 DAP, but it was almost undetectable in the seed coat. By 20 DAP, HSP90.6 transcripts accumulated at low levels except in the scutellum (Figure 2B). These results showed that HSP90.6 was maintained at a high transcript level in the early stage of seed development. Furthermore, a subcellular localization assay showed that HSP90.6 is localized to the nucleus and cytoplasm (Figure 2C).
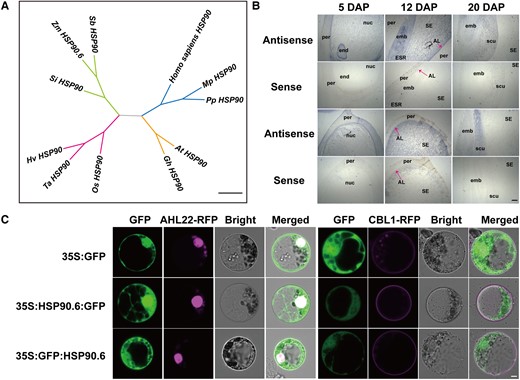
HSP90.6 is a heat shock protein located in the cytoplasm and nucleus. A, Phylogenetic relationship of HSP90.6 and its homologs. Scale bar = 0.2 amino acid replacement rate. (B) In situ hybridization analysis of the expression of HSP90.6 in LH244 seeds at different stages. Scale bar = 200 μm. AL, aleurone layer; emb, embryo; end, endosperm; ESR, embryo-surrounding region; nuc, nucellus; per, pericarp; scu, scutellum; SE, starchy endosperm. C, Subcellular localization of HSP90.6 and its colocalization with organelle markers in maize protoplasts. AtAHL22-RFP, nuclear marker; AtCBL1-RFP, cell membrane marker. Scale bar = 5 μm.
To understand the evolution of HSP90.6, a phylogenetic tree was constructed based on the HSP90.6 protein sequences of maize and its homologs (Supplemental Table 2). The results showed that HSP90.6 was especially similar to its orthologues in sorghum (Sorghum bicolor L.) and millet (Setaria italica) (Figure 2A). Moreover, we found that there are additional HSPs in maize. In maize, the HSP90 subfamily has seven members (Supplemental Figure 3A), but their function is unknown.
A single-amino acid mutation of HATPase_c affects the function of HSP90.6
The ATPase domain is necessary for HSP90 to function. This domain has ATPase activity and promotes HSP90 to assist protein folding by consuming ATP (Sima and Richter, 2018; Donato and Geisler, 2019). In animals, whether HSP90 mutation or drug-based inhibition, a reduction in HSP90 activity leads to various abnormal phenotypes (Zuehlke and Johnson, 2010). It has been reported that a single-amino acid mutation in the ATPase domain affects its ATPase activity (Rehn et al., 2020). Therefore, we speculated that the smaller seeds may be caused by a decreased ATPase activity of ehsp90.6. To verify this hypothesis, the protein structures of HSP90.6 and ehsp90.6 were predicted via I-TASSER (https://zhanglab.ccmb.med.umich.edu/I-TASSER/). A structural comparison was conducted by PyMOL (https://pymol.org/2/), which revealed that the protein structures were not substantially different (Figure 3A; Supplemental Figure 4C). Next, Swiss-Pdb Viewer was used to search for hydrogen bonds between the amino acids, and the results indicated that, unlike Arg127 and Glu123, which formed a hydrogen bond, a hydrogen bond was formed between Gln127 and Val124 of ehsp90.6 (Supplemental Figure 4, A and B). In addition, the ligand binding sites and the enzyme active sites were changed in ehsp90.6 (Supplemental Figures 5 and 6). Furthermore, we measured the ATPase activity of HSP90.6 and ehsp90.6 purified in E. coli (BL21), which showed that the ATPase activity of ehsp90.6 was significantly lower than that of HSP90.6 (Figure 3B). These results suggested that the single-amino acid mutation in ehsp90.6 influenced its ATPase activity.
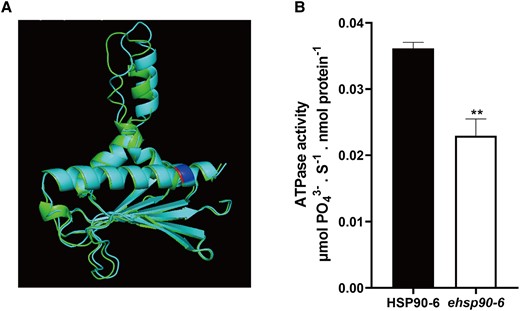
Comparison of HATPase_c structure and verification of its activity in WT and ehsp90.6. A, Comparison of predicted WT and ehsp90.6 HATPase_c domain structures. Green represents HSP90.6, cyan represents ehsp90.6, and red and blue represent amino acid mutation sites. B, ATPase activity of HSP90.6 and ehsp90.6. Molybdenum blue method was used to determine the concentration of , and the reaction rates were calculated between 0 and 10 s. For each sample, three independent biological replicates were analyzed. Values are averages and error bars are SDs. n = 3. **, P < 0.01 (Student's t test).
The metabolism of carbon and nitrogen is disrupted in hsp90.6
The HATPase_c domain is necessary for HSP90, and changes in its activity directly affect the regulatory mechanism in which HSP90 participates (Pearl and Prodromou, 2006). To explore the effect of the hsp90.6 mutation on seed development, embryos and endosperm of wild-type and mutant plants were isolated from segregated ears at 10 DAP, and total RNA was extracted to construct a library for next-generation sequencing. The correlation coefficient of the data was greater than 0.9, indicating that the sequencing quality could be used for further analysis (Supplemental Figure 7, A and B). Through comparison with the WT data, 6,226 differentially expressed genes (DEGs) were identified (screening criteria of |log2(fold-change)| > 1 and Q-value < 0.05), among which 3,510 genes were upregulated and 2,716 genes were downregulated (Supplemental Figure 7C and Table 3).
To understand the functions of DEGs, Gene Ontology (GO) functional enrichment analysis was conducted, which revealed that HSP90.6 was widely involved in intracellular processes, including protein homeostasis, signal transduction, transcriptional regulation, and nutrient metabolism. As expected, genes related to protein metabolism were highly enriched (Figure 4A; Supplemental Table 4), because HSP90 mediates protein assembly and helps polypeptides fold and be transported (Schopf et al., 2017). Regarding signal transduction and transcriptional regulation, related studies have reported that HSP90 carries out these functions by activating steroid hormone receptors, protein kinases, and transcription factors (Picard et al., 1990; Pratt, 1997; Jackson et al., 2004).
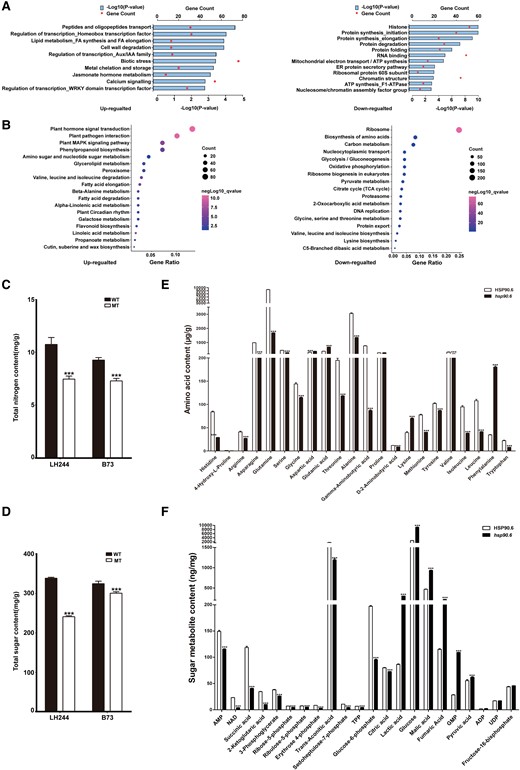
Effects of hsp90.6 on seed development. A, Functional classification of DEGs between hsp90.6 and WT. B, KEGG pathway enrichment analysis of the DEGs. C, Total nitrogen content in wild-type and hsp90.6/ehsp90.6 samples. D, Total sugar content in wild-type and hsp90.6/ehsp90.6 samples. The sugar and nitrogen contents in LH244 and hsp90.6, B73 and ehsp90.6 were measured, respectively. E, The amino acid contents in HSP90.6 and hsp90.6. F, The sugar metabolite contents in HSP90.6 and hsp90.6. For each sample, three independent biological replicates were analyzed. Values are averages and error bars are SDs. n = 3. ***, P < 0.001 (Student's t test).
For nutrient metabolism, Kyoto Encyclopedia of Genes and Genomes (KEGG) pathway analysis was performed on the DEGs to determine the metabolic processes in which they participated. Pathways involving biosynthesis of amino acids and carbon metabolism were highly enriched in downregulated genes (Figure 4B; Supplemental Table 5). Therefore, we measured the sugar and nitrogen contents in WT and hsp90.6/ehsp90.6 samples, and the results showed that they were significantly reduced in the MT samples (Figure 4, C and D). In addition, we also measured the contents of each amino acid and the contents of some sugar metabolites in WT and hsp90.6 samples. The contents of most amino acids decreased, and more than half of the sugar metabolites decreased in the hsp90.6 mutant (Figure 4, E and F). These results indicate that the seed filling of the hsp90.6 mutant was severely affected. Therefore, the DEGs affecting seed filling were analyzed. The transcript levels of Opaque 2 (O2), O11, Opaque Endosperm and Small Germ 1 (OS1), Miniature Seed 6 (MN6), Brittle 2 (Bt2), Prolamin-box factor 1 (PBF1), Naked endosperm 2 (NKD2), and NAM, ATAF, and CUC TFs 130 (NAC130) decreased significantly in the hsp90.6 mutant. OS1 plays roles in nutrients uptake and allocation during maize seed filling and development, as well as regulating MN6 (Song et al., 2019; Yi et al., 2021). O11 is an endosperm-specific transcription factor that can transcriptionally activate NKD2, and NKD2 directly regulates the expression of O2. O2 is the central regulator of seed filling, which, through interaction with PBF1, could transcriptionally activate sucrose cleavage, starch biosynthesis, and protein storage-related genes (Zhang et al., 2015, 2016; Gontarek et al., 2016; Yang et al., 2016, 2021; Feng et al., 2018; Deng et al., 2020). NAC130 regulates the expression of Bt2 and 16-kDa γ-zein. The starch and protein contents of nac130 mutant seeds are dramatically reduced (Zhang et al., 2019).
HSP90.6 affects nutrient metabolism by interacting with RPN6, PBD2 (PRC2), and GF14-4
To explore how HSP90.6 affects C/N metabolism, an antibody against HSP90.6 was prepared (Supplemental Figure 8), and total proteins of WT and hsp90.6 embryos and endosperm at 10 DAP were extracted for immunoprecipitation (IP). The immunoprecipitated proteins were identified by mass spectrometry (MS), and their sequences were queried via BLAST in the MaizeGDB. To screen the interacting proteins of HSP90.6 more accurately, we performed the experiment using three replicates of the wild-type, and obtained 638 proteins by overlapping the data. Then, removing the non-specific binding proteins by overlapping the data of the WT and hsp90.6 mutant, we obtained a total of 52 specific proteins, and the corresponding protein functions and studies were accessed in Ensembl (https://ensembl.gramene.org/Zea_mays/Location/View?r=1:8001-18000;db=core) (Figure 5, A and B; Supplemental Table 6).
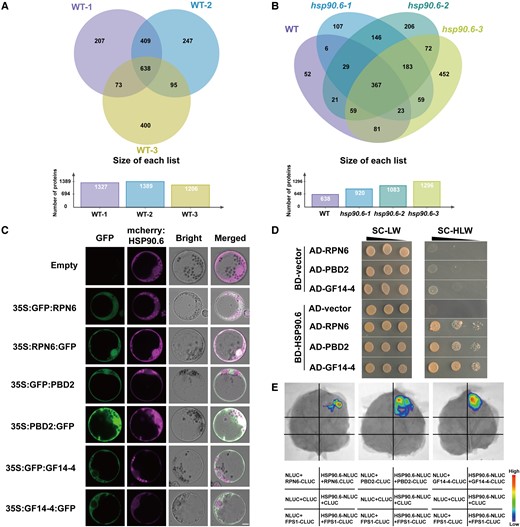
Screening, colocalization, and interaction of HSP90.6-interacting proteins. A, Venn diagram of IP-MS results of three biological repeats of WT. The proteins obtained in three biological repeats of WT overlapped, and 638 proteins were obtained. B, Venn diagram of IP-MS results of three biological repeats of WT overlapping with three biological repeats of hsp90.6. Non-specific binding proteins were removed from the 638 proteins through overlapping with the proteins obtained in hsp90.6, and 52 interacting proteins were finally obtained. Three candidate proteins RPN6, PBD2, and GF14-4 were screened by the results of KEGG and related studies of 52 interacting proteins. The IP was performed with anti-HSP90.6 in WT (LH244) and hsp90.6, with WT as the experimental group and hsp90.6 as the negative control. C, Subcellular localization showing that HSP90.6 colocalizes with RPN6 and PBD2 and overlaps with GF14-4. Scale bar = 5 μm. D, Yeast two-hybrid assays showing that HSP90.6 interacts with RPN6, PBD2, and GF14-4. AD, activating domain; BD, binding domain. E, LCI showing that HSP90.6 interacts with RPN6, PBD2, and GF14-4. The fluorescent signal intensities represent their interaction activities. FPS1 (Farnesyl Pyrophosphate Synthetase 1), negative control.
Considering the connection between nitrogen recycling and the ubiquitin–proteasome system, the 26S proteasome subunits REGULATORY PARTICLE NON-ATPASE 6 (RPN6) and PROTEASOME BETA SUBUNIT D2 (PBD2) (PROTEASOME COMPONENT 2 [PRC2]) were selected for verification. The interaction between HSPs and the 26S proteasome has been reported in animals and yeasts (Verma et al., 2000; Park et al., 2007; Kandasamy and Andréasson, 2018). However, there are no reports of embryonic lethality caused by the loss-function of the 26S proteasome (Wang et al., 2019). GENERAL REGULATORY FACTOR 14-4 (GF14-4), another protein identified via IP-MS, attracted our attention. The GF14-4 protein mainly regulates C/N metabolism (Diaz et al., 2011), and there is evidence in maize seeds that ZmGF14-4 is involved in glycolysis and post-glycolysis metabolism (Dou et al., 2015). Since we found that genes involved in C metabolism were downregulated in the hsp90.6 mutant, and the GF14-4 protein is likely to function in C metabolism, we decided to further study this protein. To verify the relationships between HSP90.6 and these proteins, the subcellular localizations of RPN6, PBD2 (PRC2), and GF14-4 were predicted through CELLO (http://cello.life.nctu.edu.tw/cello2go/). RPN6 and PBD2 (PRC2) were located in the nucleus and cytoplasm, which is consistent with the location of HSP90.6. GF14-4 was mainly distributed in the cytoplasm, which overlapped with the location of HSP90.6. Subsequently, subcellular localization was performed in maize protoplasts, and RPN6 and PBD2 (PRC2) completely overlapped with HSP90.6. The cytoplasmic location of GF14-4 also overlapped with that of HSP90.6 (Figure 5C). In summary, HSP90.6 colocalizes with RPN6, PBD2 (PRC2), and GF14-4. In addition, yeast two-hybrid and luciferase complementation imaging (LCI) assays were conducted to prove the interactions between HSP90.6 and RPN6, PBD2 (PRC2), and GF14-4 (Figure 5, D and E). These results revealed that HSP90.6 interacted with RPN6, PBD2 (PRC2), and GF14-4 both in vivo and in vitro.
Loss of function of HSP90.6 reduces 26S proteasome activity
In eukaryotes, the ubiquitin–proteasome system (UPS) degrades short-lived regulatory proteins and soluble misfolded proteins through the 26S proteasome (Schubert et al., 2000; Vierstra, 2009; Finley et al., 2012; Samant et al., 2018; Marshall and Vierstra, 2019). And the complex structure of the 26S proteasome requires the assistance of a variety of chaperone proteins (Budenholzer et al., 2017; Bard et al., 2018). HSP90 is usually part of the final step to promote protein folding, assembly of multiprotein complexes, and binding of ligands to substrates. It has a large substrate-binding interface, which preferentially binds to late-folding intermediates that contain tertiary structures (Jakob et al., 1995; Karagöz et al., 2014; Abildgaard et al., 2020). As a subunit of RP, RPN6 is an important component of the 26S proteasome and has been identified as a molecular tweezer that fixes CP and RP together to form a fully activated 26S proteasome (Santamaria et al., 2003; Isono et al., 2005; Pathare et al., 2012; Estrin et al., 2013; Cho et al., 2015; Tian and Trader, 2020). Specifically, RPN6 interacts with RPT6 of RP and the α2 subunit of CP (Chen et al., 2016; Tian and Trader, 2020). PBD2 (PRC2) is a subunit of CP and is responsible for endowing active sites with chymotrypsin cleavage properties and maintaining the degradation activity of the 26S proteasome (Arendt and Hochstrasser, 1997; Heinemeyer et al., 1997; Dick et al., 1998; Kisselev et al., 1999, 2003; Kumar Deshmukh et al., 2019; Marshall and Vierstra, 2019). We speculated that the interactions of HSP90.6 with RPN6 and PBD2 (PRC2) would affect the activity of the 26S proteasome.
To verify that the activity of the 26S proteasome was impaired, the 26S proteasomes were separated via molecular sieves from WT and hsp90.6 embryos and endosperm at 18 DAP. A total of 20 fractions were collected and detected by Western blotting with anti-HSP90.6/PSMD13/PSMA3. The results showed that 26S proteasomes were distributed in fractions 2–7 (Figure 6A). Then, fractions 2–7 were mixed, and a specific fluorescent matrix was used to detect the hydrolytic activity of the 26S proteasome in WT and hsp90.6 on Suc-LLVY-AMC. Compared with that of the WT, the hydrolysis activity of the 26S proteasome of hsp90.6 was reduced by 74.9% (Figure 6B). At the same time, native PAGE was used to test the activity of the 26S proteasome, and consistent results were obtained (Figure 6C). Therefore, hsp90.6 presented reduced 26S proteasome activity.
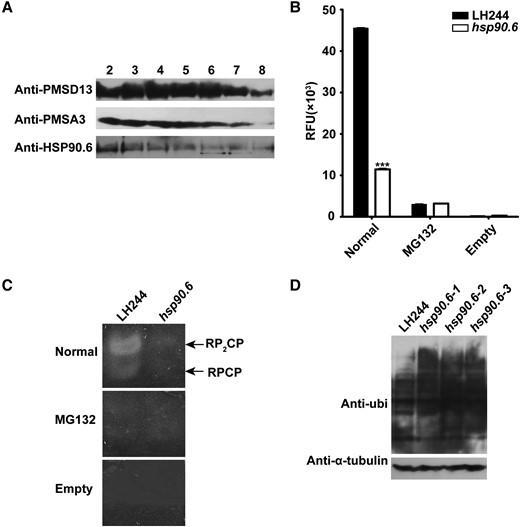
Hsp90.6 reduces 26S proteasome activity. A, Total protein extracted from the embryos and endosperm at 18 DAP were separated by a molecular sieve and then analyzed by Western blotting in conjunction with anti-HSP90.6, anti-PSMD13, and anti-PMSA3. PSMD13 encodes a non-ATPase subunit of the 19S regulator. PMSA3 encodes a 20S core α subunit. B, 26S proteasome activity analysis of embryos and endosperm at 18 DAP in solution. RFU, relative fluorescence units; Normal, 26S proteasome and Suc-LLVY-AMC; MG132, 26S proteasome, Suc-LLVY-AMC and MG132; Empty, Suc-LLVY-AMC. For each sample, three independent biological replicates were analyzed. Values are averages and error bars are SDs. n = 3. ***, P < 0.001 (Student's t test); (C) 26S proteasome activity in-gel analysis of embryos and endosperm at 18 DAP; (D) Ubiquitinated proteins of embryos and endosperm at 18 DAP were analyzed by Western blotting in conjunction with anti-ubi.
As proteasome damage increases the level of ubiquitinated substrate, anti-ubi was used to analyze the level of ubiquitinated proteins in the embryo and endosperm of the WT and hsp90.6 mutant. The Western blotting results showed that the content of ubiquitinated proteins of hsp90.6 obviously increased (Figure 6D). Next, IP-MS was used to analyze ubiquitinated proteins in the embryo and endosperm at 18 DAP. Through the comparison of WT and hsp90.6, a total of 271 accurately quantified proteins were identified. Under the conditions of |log2(fold-change)|≥1 and Q-value < 0.05, 26 differentially ubiquitinated proteins were screened. The accumulation of 20 proteins was increased, which included mainly 60S ribosomal proteins and enzymes. 60S ribosomal proteins are related to translation, and the enzymes are involved in different processes. For example, HISTONE DEACETYLASE 102 (Zm00001d011139) regulates histone acetylation, and its accumulation disrupts gene expression (Shahbazian and Grunstein, 2007). The increase in proteins further indicated that the activity of the 26S proteasome decreased. However, the contents of six proteins were downregulated, which may be because of the failure of the transcriptional and translational regulation pathway involved in HSP90.6, as the transcription levels of five of these proteins were reduced (Supplemental Table 7). The results implied that HSP90.6 is essential for maintaining 26S proteasome activity.
GF14-4 interacts with GPC1 and PGK3 to influence carbon metabolism
Among the DEGs, those related to carbon metabolism were significantly downregulated in hsp90.6, which could have an impact on seed filling. GF14-4 is a subtype of 14-3-3 proteins in maize. 14-3-3 proteins are typical cytoplasmic proteins and participate in various pathways such as sugar metabolism and lipid metabolism (Aducci et al., 2002). 14-3-3 proteins usually combine with phosphorylated proteins, and PTM Viewer (https://www.psb.ugent.be/webtools/ptm-viewer) showed that both Ser677 and Ser688 of HSP90.6 are phosphorylated (Supplemental Figure 9).
To understand the regulatory mechanism of GF14-4, IP-MS was performed. A 35S:GF14-4:GFP vector was constructed and transformed into maize protoplasts. After 24 h of culture, the protoplasts were collected and the total proteins were extracted. Anti-GFP was used for IP-MS, and 25 candidate proteins were obtained according to the description of screening HSP90.6 interacting proteins (Figure 7, A and B). Next, we predicted the subcellular localization of these 25 proteins in CELLO and finally identified 9 proteins localized in the cytoplasm (Supplemental Table 8). We selected the proteins related to glucose metabolism, including PHOSPHOGLYCERATE KINASE 3 (PGK3; Zm00001d049641), PHOSPHOENOLPYRUVATE CARBOXYLASE 1 (PEP1; Zm00001d046170) and GLYCERALDEHYDE-3-PHOSPHATE DEHYDROGENASE 1 (GPC1; Zm00001d015376) for verification. Moreover, we found that ADENOSYL HOMOCYSTEINE HYDROLASE 1 (AHH1; Zm00001d049239) was the only protein overlapped with the IP-MS results of HSP90.6; this protein catalyzes the hydrolysis of S-adenosyl-l-homocysteine (SAH) and enables smooth methyl transfer (Min et al., 2014). The interaction between GF14-4 and these four proteins was verified with LCI. The results confirmed that GF14-4 interacts with GPC1, PGK3, and AHH1 (Figure 7C), which demonstrated that GF14-4 participated in carbon metabolism through interaction with GPC1 and PGK3.
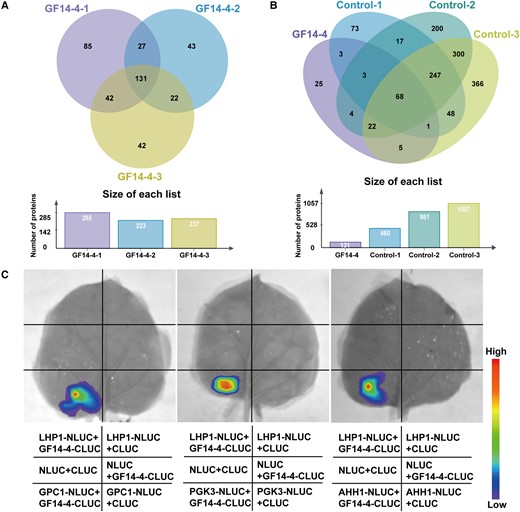
Identification and verification of GF14-4 interaction proteins. A, Venn diagram of IP-MS results of three biological repeats of GF14-4. The proteins obtained in three biological repeats of GF14-4 overlapped, and 131 proteins were obtained. B, Venn diagram of IP-MS results of three biological repeats of GF14-4 overlapping with three biological repeats of control. Non-specific binding proteins were removed from the 131 proteins through overlapping with the proteins obtained in control, and 25 interacting proteins were finally obtained. C, LCI showing that GF14-4 interacts with GPC1, PGK3, and AHH1. The IP was performed with anti-GFP in LH244 protoplasts, with GF14-4 as the experimental group and control as the negative control. GF14-4, GF14-4-GFP fusion protein was expressed in LH244 protoplasts by 35S:GF14-4:GFP; Control, GFP protein was expressed in LH244 protoplasts by 35S:GFP. C, LCI showing that GF14-4 interacted with GPC1, PGK3, and AHH1. LHP1 (Like Heterochromatin Protein 1), negative control.
In conclusion, we depicted a working model of HSP90.6 based on our results. HSP90.6 interacts with RPN6/PBD2(PRC2) to affect the activity of 26S proteasome, which is responsible for the degradation of ubiquitinated proteins, and provides amino acids for nitrogen recycling. Additionally, HSP90.6 interacts with GF14-4 and thus is indirectly involved in carbohydrate metabolism (Figure 8).
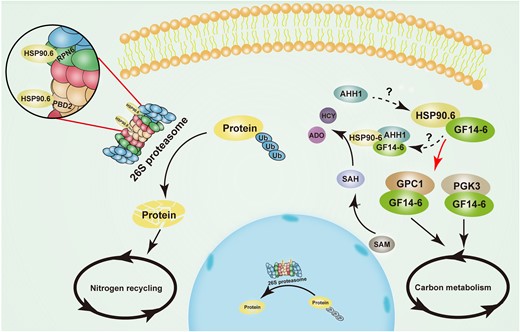
Model of ZmHSP90.6 regulating nutrient metabolism in maize seed development. HSP90.6 interacts with RPN6/PBD2 (PRC2) to regulate 26S proteasome activity and nitrogen recycling. In addition, HSP90.6 interacts with GF14-4 to regulate PGK3 and GPC1 to participate in carbon metabolism. HSP90.6 may form a trimer with GF14-4 and AHH1 to participate in SAH hydrolysis. The red arrow indicates the molecular mechanism revealed in the work. ADO, Adenosine; HCY, l-homocysteine; SAM, S-adenosyl-methionine; SAH, S-adenosyl-l-homocysteine; Ub, Ubiquitin.
Discussion
Carbon and nitrogen are essential nutrients for seed filling and development, and their metabolism determines the morphogenesis of seeds (Goel et al., 2016). In this work, we found that HSP90.6 was involved in C/N metabolism, suggesting that it functions in maintaining nutrient metabolism in maize kernels. The hsp90.6 mutant showed severe seed development defects (Supplemental Figure 2, B–D), which indicated that HSP90.6 participates in the regulation of maize seed development. There are reports that the loss of AtHSP90.6 and AtHSP90.5 in Arabidopsis causes embryonic death (Feng et al., 2014; Luo et al., 2019). However, the underlying molecular mechanism remains unknown. Here, we revealed that HSP90.6 interacted with RPN6/PBD2 (PRC2) to regulate nitrogen recycling by influencing 26S proteasome activity. It has been reported that AtHSP101 works synergistically with the 26S proteasome to promote the clearance of ubiquitinated proteins by refolding rather than proteasomal degradation, and AtHSP101 deletion has no effect on the 26S proteasome (McLoughlin et al., 2019). Therefore, the regulatory mechanisms of HSP90.6 and AtHSP101 are different. Moreover, we verified that HSP90.6 was indirectly involved in carbon metabolism through interacting with the 14-3-3 protein GF14-4 (Figure 8).
HSP90.6 is required for 26S proteasome-mediated nitrogen recycling
Nitrogen recycling is inseparable from the ubiquitin proteasome system, and the core of the ubiquitin proteasome system is the 26S proteasome. The assembly of the 26S proteasome requires a series of proteasome-specific chaperones to ensure that each subunit finds its correct position in the newly synthesized proteasome (Budenholzer et al., 2017). Many related chaperones have been reported in animals, such as PROTEASOME ASSEMBLY CHAPERONES 1 (PAC1)–PAC2 and PAC3–PAC4 heterodimers to promote the assembly of α loops (Kusmierczyk et al., 2008, 2011; Yashiroda et al., 2008); UB-MEDIATED PROTEOLYSIS 1 (Ump1) plays a role in the binding of β subunits and half-dimers (Ramos et al., 1998). Here, we proved that the mutation of hsp90.6 resulted in decreased 26S proteasome activity and the accumulation of ubiquitinated proteins that affected nitrogen recycling (Figure 6, B–D; Supplemental Table 7). We speculated that HSP90.6 may promote the function of RPN6 as a molecular tweezer, and cause PBD2 (PRC2) to lose its chymotrypsin cleavage activity, which directly affects the function of the 26S proteasome.
HSP90.6 plays an important role in carbon metabolism
In a study on Defective Kernel 40 (ZmDEK40), the lack of DEK40 substantially affected the biogenesis of 20S CP, resulting in a decrease in 26S proteasome activity (Wang et al., 2019). However, the dek40 mutant produced smaller kernels only, suggesting that the interaction of HSP90.6 with RPN6 and PBD2 (PRC2) was insufficient to lead to the lethal phenotype of hsp90.6. RNA-sequencing (RNA-seq) analysis showed that the loss of HSP90.6 caused the downregulation of carbon metabolism-related genes (Figure 4B), and the 14-3-3 protein GF14-4 was identified by IP-MS (Supplemental Table 6).
In plants, 14-3-3 proteins regulate protein interactions and enzyme activity in a phosphorylation-dependent manner, including transcription factors, ion channel proteins, and kinases (Kong and Ma, 2018). Phosphorylation of HSP90 has been studied in animals. A specific phosphorylation site of human HSP90β, Ser365, has been shown to affect the interaction of HSP90 and CELL DIVISION CYCLE 37 (CDC37) (Nguyen et al., 2017). In addition, a large number of phosphorylation sites on HSP90α and HSP90β have been predicted by the PhosphositePlus database (Hornbeck et al., 2012), which also implied the possibility of interactions between HSP90 and 14-3-3s. We found that the 14-3-3 protein GF14-4 interacted with the carbon metabolism-related proteins GPC1 and PGK3 (Figure 7, A–C). Therefore, we speculated that the mutation of hsp90.6 led to impaired carbon metabolism and severely inhibited seed filling. But this effect was indirect, and HSP90.6 may be phosphorylated first, and then combined with GF14-4. Their combination may cause conformation changes in GF14-4, which would become active and affect GPC1 and PGK3. Notably, through IP-MS, we found that HSP90.6 and GF14-4 had an overlapping protein (AHH1), which is responsible for catalyzing the decomposition of adenosine homocysteine to ensure the normal supply of methyl compounds (nucleic acid, protein). Therefore, we speculated that the process may be carried out by the formation of a complex comprised of HSP90.6, GF14-4, and AHH1.
The genes encoding the transcription factors O2, O11, NKD2, and NAC130, which have been reported to regulate seed filling, were all downregulated in hsp90.6, which meant that HSP90.6 may play an upstream regulatory role. However, HSP90.6 had no transcriptional activation activity (Supplemental Figure 10); therefore, we hypothesized that bHLH95 (Zm00001d003677) and putative MYB protein (Zm00001d049495), among the 52 interacting proteins identified with IP-MS (Supplemental Table 6), might regulate downstream genes under the action of HSP90.6. In addition, we also detected other proteins, such as DULL ENDOSPERM1 (DU1; Zm00001d000002), HISTONE DEACETYLASE 102 (HD102; Zm00001d011139), GLYCOSYLTRANSFERASE 6 (GT6; Zm00001d051681), which may interact with HSP90.6 to have different functions and regulate seed development. Therefore, only a portion of the mechanism of HSP90.6 was resolved in our study, and the rest needs to be further studied.
Materials and methods
Plant material
The ehsp90.6 mutation in maize (Zea mays L.) was induced by EMS. For this, mature pollen of maize B73 plants was soaked in 0.015% (v/v) EMS solution (volumetric ratio of 1:10) for 10–15 min, and then selfed to obtain T1 seeds. The T1 seeds were planted and selfed to obtain the T2 ears for phenotypic identification.
The maize hsp90.6 mutant was obtained by clustered, regularly interspaced, short palindromic repeats (CRISPR)/CRISPR-associated 9 (Cas9) and transformed by the maize genetic transformation platform of the National Maize Improvement Center of China Agricultural University. The approximate operation method was as follows: two gRNA sequences were inserted into the binary vector pXUE411C-BG according to a previous report (Xing et al., 2014), and gRNAs from CRISPR-P (http://crispr.hzau.edu.cn/CRISPR/), CCCGGAGCAGCTCATTCCTA and TCAATGAGTACTTGGGCCCT, were selected. The Agrobacterium cells containing the target vector were infiltrated into LH244 embryos (1.5–1.8 mm) at 12 DAP for Agrobacterium-mediated transformation (Lagrimini, 2018). Herbicides were used to select positive transformants, which were verified by DNA sequencing at the seedling stage. In this work, the embryos and endosperm for RNA-seq, IP-MS, and 26S proteasome experiments were purified using CRISPR/Cas9 materials.
BSR-seq
T2 seeds of the maize WT (B73) and ehsp90.6 embryos and endosperm at 12 DAP were taken and total RNA was extracted with TRIzol™ reagent (15596018, Thermo Fisher Scientific, USA).
cDNA libraries were constructed using an AHTS Stranded mRNA-seq Library Prep Kit for Illumina V2 (NR612, Vazyme, China). The sequences were obtained by an Illumina X-10 instrument (Illumina, USA). The raw data were compared with the B73_V4 reference genome data by HISAT2 v2.0.4 (http://daehwankimlab.github.io/hisat2/), with the default parameters (HISAT is a fast splice aligner with low memory requirements); the BAM file was output; and SAMtools v0.1.19 (http://samtools.sourceforge.net/) (in the sequence alignment/map format) was used for sorting. Single-nucleotide polymorphism (SNP) calling was performed with unique reads through SAMtools v0.1.19 and BCFtools. The screening criteria for high-confidence SNPs were as follows: (1) SNPs supported at least five reads and (2) SNPs in the WT pool corresponding to the mutant pool were detected. SnpEff v4.2 (https://pcingola.github.io/SnpEff/), which is a program for annotating and predicting the effects of SNPs, was used to annotate the SNPs.
Identification of candidate genes
The degree of linkage between the frequency of each SNP and the phenotype was calculated, and the SNP-corresponding genes with the highest linkage degree were screened. Then, SNPs on the genes of 300 segregating populations of the T3 generation were detected, and candidate genes that co-segregated with the phenotype were identified.
Reverse transcription quantitative PCR
Five micrograms of total RNA was collected, and a Hifair® II 1st Strand cDNA Synthesis Kit (with gDNA Digester Plus) (11121ES60, YEASEN, China) was used for reverse transcription. RT-qPCR was performed in conjunction with Takara TB Green® Premix Ex Taq™ (Tli RNase-H Plus), Bulk (RR420L, Takara, Japan).
Phylogenetic analysis
The protein sequence of HSP90.6 was searched using BLASTP in NCBI database (https://www.ncbi.nlm.nih.gov/) and a phylogenetic tree was constructed with MEGA v7.0 (https://www.megasoftware.net/download_form). The muscle algorithm was used for multiple sequence alignment. The statistical method used was the adjacency matrix method (neighbor-joining [NJ] method). The bootstrap method was used for the phylogenetic tree tests, in which the number of tests was 1,000 and the replacement model was the Poisson model. The phylogenetic tree was visualized by iTOL (https://itol.embl.de/).
Subcellular localization
The coding sequence (CDS) of HSP90.6 was cloned into the N-terminal region and the C-terminal region of pUC:35S:GFP, and the expression vectors were transformed into maize protoplasts using PEG-Ca2+ as described by Yoo et al. (2007). Then, the protoplasts were cultivated at 28°C in the dark for 18–24 h. GFP signal was imaged under 488 nm with excitation, and the emission signal was collected at 493–586 nm. RFP signal was imaged under 561 nm with excitation, and the emission signal was collected at 562–688 nm. Laser intensity is 22% and gain is between 480 and 630 dB.
ATPase activity
The CDSs of the HSP90.6 and ehsp90.6 were ligated into pET-32a, which were subsequently transformed into BL21 (DE3) competent cells for protein expression and purification. ATPase activity was measured by the molybdenum blue method with minor modifications (Rodriguez et al., 1994; Norgan et al., 2013; Yamane et al., 2019). We incubated 75 μl of reaction solution (30 mM Tris at pH 7.5, 8 mM MgCl2, 100 mM NaCl, 1 mM dithiothreitol [DTT], 4% (w/v) sucrose, 30 μg/ml bovine serum albumin [BSA], 1 mM phenylmethylsulfonyl fluoride [PMSF], 0.2 mM ATP, and 100 nM of prokaryotic purified HSP90.6/ehsp90.6) at 30°C for 0/10 s and then immediately added 175 μl of color developing solution (0.35% (m/v) (NH4)2MoO4·4H2O, 0.86 M H2SO4, and 1.4% (w/v) ascorbic acid). Then, they were incubated at 42°C for 30 min, and the absorbance (A660) was measured with a micro plate reader (Molecular Devices i3x, USA).
RNA-seq analysis
A cDNA library was constructed with a Hieff NGS MaxUpTM Dual-mode mRNA Library Prep Kit for Illumina (H9001360, Vazyme, China) and sequenced on an Illumina Nova-seq platform (Illumina, USA), generating 150-nucleotide paired-end reads. FASTP v0.20.1 (http://github.com/OpenGene/fastp) was used to remove low-quality reads. HISAT2 v2.2.1 (http://daehwankimlab.github.io/hisat2/) with the default parameters were used to map the original reads with the sequence information of the B73_V4 reference genome. Then the gene expression levels were measured via fragments per kilobase of exon model per million mapped fragments (FPKM) using Cufflinks (V2.2.1). The differential gene screening criteria were |log2(fold-change) |≥1 and Q-value <0.05.
Total sugar content measurement
Mature WT and mutant seeds were used for the experiment, and a total sugar content assay kit was used (AKSU003C, BOXBIO, China). First, we mixed 0.1 g of sample, 1 ml of extraction buffer A (27.6 mM 3,5-dinitrosalicylic acid) and 1.5 ml of ddH2O at 100°C for 30 min. Then, 1 ml of extraction buffer B (524 mM NaOH, 0.86 M seignette salt) was added, and afterwards the mixture was cooled to room temperature, diluted to 10 ml with ddH2O and centrifuged at 8000×g for 10 min. The absorbance (A540) was measured with a microplate reader (Molecular Devices i3x, USA) by mixing 150 μl of supernatant, 150 μl of chromogenic (40 mM sodium sulfite, 53 mM phenol) solution, and 900 μl of ddH2O.
Nitrogen content measurement
Mature WT and mutant seeds were used for the experiment. We mixed 0.1 g of sample, 0.5 ml of ddH2O and 1 g of catalyst (K2SO4:CuSO4 = 10:1), and 5 ml concentrated sulfuric acid was added. The mixture was heated at 200°C for 1 h and 380°C for 2 h until it turned peacock green. After cooling, the mixture was diluted 50 times and filtered with a 0.22 μm membrane. Finally, a 40 μl diluted sample was mixed with 160 μl buffer C (0.95 M sodium potassium tartrate, 0.59 M Nessler reagent [N874804, MACKLIN, China]). The absorbance (A420) was measured with a microplate reader (Molecular Devices i3x, USA) after 10 min.
Detection of sugar metabolites
Approximately 100 mg of frozen sample was extracted in 1 ml of ice-cold methanol/acetonitrile/water (2:2:1, v/v/v). The sample was sonicated for 30 min twice for 30 min on ice. Samples were then incubated at −20°C for 1 h to precipitate proteins. After centrifugation (4°C, 12,000 rpm, 10 min), the supernatant was dried under vacuum. For UHPLC-ESI-MS/MS analysis, the sample was dissolved in 200 μl of 50% ACN (v/v) and analyzed using a UPLC-Orbitrap-MS system (UPLC, Vanquish; MS, QE). The analytical conditions were as follows: UPLC column, Waters BEH Amide (100*2.1 mm, 1.8 μm); column temperature, 40°C; flow rate, 0.3 ml/min; injection volume, 2 μl; solvent system, 10 mM NH4Ac: acetonitrile; gradient program, 10:90 V/V at 0 min, 60:40 V/V at 9.0 min, 10:90 V/V at 9.1 min, 90:10 V/V at 12.0 min. HRMS data were recorded on a Q-Exactive hybrid Q-Orbitrap mass spectrometer equipped with a heated ESI source (Thermo Fisher Scientific, USA) utilizing the Full scan-ddms2 MS acquisition methods. The ESI source parameters were set as follows: spray voltage, 2.8 kV; sheath gas pressure, 40 arb; aux gas pressure, 10 arb; sweep gas pressure, 0 arb; capillary temperature, 320°C; and aux gas heater temperature, 350°C. Data were acquired on the Q-Exactive using Xcalibur 4.1 (Thermo Scientific, USA), and processed using TraceFinder™4.1 Clinical (Thermo Scientific, USA). Quantified data were output into excel format.
Amino acid content detection
Approximately 100 mg of frozen sample was extracted with 0.6 ml 0.1 M hydrochloric acid for 1 h at room temperature. Each sample was filtered through a 0.22 μm membrane filter. Then 10 μl of the sample was taken into a UHPLC vial and added 70 μl borate buffer (0.2 M boric acid, 0.05 M borax) and 20 μl AccQ•Tag reagent (0.2 M 6-aminoquinolyl-N-hydroxysccinimidyl carbamate). The reaction mixture was kept at room temperature for 1 min, heated at 55°C for 10 and 1 μl was analyzed using a UPLC-Orbitrap-MS system (UPLC, Vanquish; MS, QE). The analytical conditions were as follows: UPLC column, Waters ACQUITY UPLC BEH C18 (1.7 μm, 50*2.1 mm); column temperature, 55°C; flow rate, 0.5 ml/min; injection volume, 1μl; solvent system, water (0.1% (v/v) formic acid): acetonitrile (0.1% (v/v) formic acid); gradient program, 95:5 V/V at 0 min, 90:10 V/V at 5.5 min, 75:25 V/V at 7.5 min, 40:60 V/V at 8 min, 95:5 V/V at 8.5 min, and 95:5 V/V at 13 min. The data were analyzed as described previously for the detection of sugar metabolites.
IP assays
Polyclonal anti-HSP90.6 antibodies were produced in rabbits by HuaBio (China, http://www.huabio.cn/). The peptide sequence used as an antigen was ASIPPRPASNGAPGC.
The embryos and endosperm of WT (LH244) and hsp90.6 at 12 DAP were taken (approximately 0.5 g), and total protein was extracted according to the manufacturer's instructions of a plant protein extraction kit (KGP750, KeyGEN BioTECH, China). The reagents used in IP were from a BEAVER BeaverBeads™ Protein A (or A/G) Immunoprecipitation Kit (22202-20, Beaverbio Medical Engineering, China). The antibody was diluted to 10 μg/μl with IP binding buffer (1.1% (v/v) Triton X-100, 1.2 nM EDTA at pH 8.0, 16.7 mM Tris-HCl at pH 7.5, 200 mM NaCl, 0.5% (w/v) sodium deoxycholate), and 200 μl was added to the extracted protein and incubated for 8 h at 4°C by rotation. After that, 50 μl of protein A/G magnetic beads was added, followed by incubation at 4°C for 1 h. Magnetic separation was performed, followed by washing twice with IP washing buffer (1.3 M NaCl, 30 mM Na2HPO4, 70 mM NaH2PO4). Then, 50 μl of 1 × SDS-PAGE loading buffer (50 mM Tris-HCl at pH 6.8, 2% (w/v) SDS, 10% (v/v) glycerol) was added and mixed evenly, and the proteins were eluted at 95°C for 10 min. Finally, 1 μg protein was digested and loaded onto an LTQ ORBITRAP Velos mass spectrometer (Thermo Fisher Scientific, USA) in buffer A (2% (v/v) acetonitrile + 0.1% (v/v) formic acid) and separated with a linear gradient of buffer B (80% (v/v) acetonitrile + 0.1% (v/v) formic acid) at a flow rate of 250 nl/min controlled by IntelliFlow technology over 120 min. The most abundant precursors in the measured scans (300–1800 m/z) were dynamically selected for HCD fragmentation. The target value was determined based on predictive automatic gain control.
The MS data were analyzed by staff at the Biomass Spectrometry Laboratory of China Agricultural University through MaxQuant v1.3.0.5 (https://www.maxquant.org/) and searched against the MaizeGDB database. The initial search used a 6 ppm precursor mass window. The study followed the trypsin/P digestion rules and allowed the omission of up to two cleavage sites with a mass tolerance of 20 ppm for fragment ions. The matched peptides were screened with a significance threshold of 0.05. The mascot percolator scores were used for all peptides. The gene set enrichment analysis was performed according to Li et al. (2015a).
Yeast two-hybrid assays
The CDS of HSP90.6 was linked downstream of the GAL4 BD domain of pGBKT7. RPN6, PBD2, and GF14-4 were inserted into pGADT7. An EX-Yeast Transformation Kit and related media were used (ZC135, ZOMANBIO, China).
LCI assays
The CDSs of HSP90.6, RPN6, PBD2, and GF14-4 were inserted in pCAMBIA1300-nLUC and pCAMBIA1300-cLUC. Then, the resulting plasmids were transformed into Agrobacterium EHA105 competent cells, and the transformants were injected into leaves of 3-week-old Nicotiana benthamiana seedlings. After incubation at 28°C for 48–72 h, the leaves were removed, and a 1 mM solution of D luciferin (40902ES01, YEASEN, China) was sprayed onto the leaves. The fluorescence signal was captured by a NightShade imaging system (Berthold LB985, Germany).
In-gel peptidase activity assays of the 26S proteasome
Embryos and endosperm of WT (LH244) and hsp90.6 at 18 DAP were used for 26S proteasome purification (Book et al., 2010; Li et al., 2015b; Wang et al., 2019).
Total protein was extracted and subjected to fast-performance liquid chromatography (FPLC) using a Superdex Increase 200 10/300GL column (10246670, GE Healthcare, Sweden) to collect 1 ml fractions per sample. Twenty samples from the fraction collector were placed on ice as soon as the run was completed to detect the proteasome complexes by Western blotting with anti-HSP90.6, anti-PSMD13, and anti-PSMA3 (A6956 and A1245, ABclonal, USA).
The proteasome complexes (2–7, ≥ 670 kD) of the WT and hsp90.6 at 18 DAP were mixed, and 100 nM was taken. Afterward, 9.5 μl of buffer A (50 mM Tris-HCl at pH 7.5, 10% (v/v) glycerol, 5 mM ATP, 5 mM MgCl2, 1 mM DTT, and 1 mg/ml creatine phosphokinase) and 2.5 μl of 5 × native gel loading buffer (250 mM Tris-HCl at pH 7.5, 50% (v/v) glycerol, 5 mM ATP, 25 mM MgCl2, and 1.5 μg/l xylene cyanol FF) were added. A 4% native gel that included 1 mM ATP was used to separate 26S proteasome proteins at 4°C and 100 V for 3.5 h. Next, the gel was rinsed with water, after which 10 ml of development buffer (50 mM Tris-HCl at pH 7.5, 150 mM NaCl, 5 mM MgCl2, 1 mM ATP, and 100 μM Suc-LLVY-AMC) was added, followed by incubation at 30°C at 30 rpm for 30 min. The gel was subsequently scanned at 365 nm by a UV irradiator (Bio-Red, USA).
In-solution peptidase activity assay for 26S proteasomes
Buffer A was replaced with 100 μl of development buffer (50 mM Tris at pH 7.5, 150 mM NaCl, 5 mM MgCl2, 1 mM ATP, and 100 μM Suc-LLVY-AMC), and 35 pM of 26S proteasome protein was added, followed by incubation at 30°C for 30 min. One milliliter of 1% (v/v) SDS was used to stop the reaction. The fluorescence was measured at 380 nm excitation light and 460 nm emission light by a multifunctional microplate reader (Tecan Spark, Switzerland).
Ubiquitylome analysis
The ubiquitylome experiment was carried out for two independent biological replicates, and each replicate consisted of a mixture of embryos and endosperm from different transformants at 18 DAP. A Ubiquitin Detection Kit (SMQ-SKT-131-20, StressMarq, Canada) was used for IP. One milliliter of lysis buffer (50 mM Tris-HCl at pH 7.5, 150 mM NaCl, 0.5% (v/v) NP40, and 1 mM DTT, 0.1% (v/v) protease inhibitor cocktail) was added to 0.5 g of a liquid nitrogen ground sample, incubated at 4°C for 1 h, centrifuged at 12,000 rpm for 15 min at 4°C; then, the supernatant was collected. Protein quantification was performed by Bradford method and the concentration was adjusted to 5 mg/ml StressXpress Ubiquitin matrix was added to 200 μl of protein solution and incubate at 4°C for 1 h, followed by removal of the supernatant. The precipitate was washed twice with 500 μl of wash buffer (50 mM Tris-HCl at pH 7.5, 150 mM NaCl, 0.5% (v/v) NP40, 1 mM DTT) and finally eluted with 50 μl of 0.1% (v/v) formic acid and quantified by the Bradford method. The instrument and instrumental settings were used for MS, and the data were analyzed as described previously for the IP assays. The data were normalized prior to differential accumulation analysis. Proteins were considered to exhibit differences under |log2(fold-change) |≥1 and Q-value <0.05.
Protoplast transformation and IP-MS
The CDS of GF14-4 was cloned into the C-terminal region of 35S:GFP for protoplast transformation using PEG-Ca2+ as described by Yoo et al. (2007).
Yellowing leaves of seedlings at 10 day after germination (DAG) were crushed and combined with 20 ml enzymolysis solution, which was prepared by mixing 400 mM mannitol, 20 mM KCl, 20 mM MES, 1.5% (w/v) cellulase, and 0.4% (w/v) macerozyme, incubating at 55°C for 10 min, and then adding 1 mM CaCl2, 0.1% (w/v) BSA, and 7 μl of 2-hydroxy-1-ethanethiol. Then, the mixture was vacuumed for 30 min. Enzymatic hydrolysis was performed at 28°C and 40 rpm for 3 h. After that, the protoplasts were filtered and washed in W5 solution (125 mM CaCl2, 5 mM KCl, 144 mM NaCl, 2 mM MES). The protoplasts were centrifuged at 100×g for 3 min and resuspended in Mmg solution (400 mM mannitol, 15 mM MgCl2, 4 mM MES). Plasmids (20 µg) were added to protoplasts (200 µl) and mixed gently. Then, 220 µl of PEG solution (200 mM Mannitol, 100 mM CaCl2, 40% (w/v) PEG4000) was added, mixed gently and incubated at room temperature for 18 min. The transfection mixture was diluted with 880 μl W5 solution and mixed gently. After transfection, the mixture was centrifuged at 100×g for 2 min and the protoplasts were resuspended gently with 1 ml W5. Finally, the protoplasts were cultured at 28°C in the dark for 18–24 h and collected.
The IP-MS was performed and the data were analyzed as described previously for the IP assays. The anti-GFP was purchased from Sigma (SAB4200681, USA).
Accession numbers
HSP90.6 (Zm0000d041719); O2 (Zm00001d018971); O11 (Zm00001d003677); OS1 (Zm00001d002661); MN6 (Zm00001d037926); Bt2 (Zm00001d050032); PBF1 (Zm00001d005100); NKD2 (Zm00001d026113); NAC130 (Zm00001d008403); RPN6 (Zm00001d041456); PBD2 (Zm00001d013505); GF14-4 (Zm00001d031688); FPS1 (Zm00001d009431); LHP1 (Zm0000d014449); AtAHL22 (At2g45430); AtCBL1 (At4g17615). The bioproject accession number of raw RNA-seq data is PRJNA873864 in NCBI. The mass spectrometry proteomics data have been deposited in the ProteomeXchange Consortium via the PRIDE partner repository with the dataset identifier PXD036357, and the data are available via ProteomeXchange with the identifier PXD036357.
Supplemental data
The following materials are available in the online version of this article.
Supplemental Figure S1. Phenotypic statistics of ehsp90.6.
Supplemental Figure S2. Phenotypic tests of the ears of the CRISPR/Cas9-generated mutant hsp90.6.
Supplemental Figure S3. Characteristics of HSP90.6.
Supplemental Figure S4. Prediction and comparison of protein structure.
Supplemental Figure S5. Structural prediction of HSP90.6.
Supplemental Figure S6. Structural prediction of ehsp90.6.
Supplemental Figure S7. Analysis of DEGs.
Supplemental Figure S8. Western blot analysis of the specificity of anti-HSP90.6.
Supplemental Figure S9. PTM Viewer results of HSP90.6.
Supplemental Figure S10. Analysis of transcriptional activation activity of HSP90.6.
Supplemental Table S1. Candidate genes identified in the EMS mutants.
Supplemental Table S2. Phylogenetic tree-related gene information.
Supplemental Table S3. Genes differentially expressed between hsp90.6 and the WT.
Supplemental Table S4. Functional classification of DEGs between hsp90.6 and the WT.
Supplemental Table S5. KEGG pathway enrichment analysis of the DEGs.
Supplemental Table S6. IP-MS data of HSP90.6.
Supplemental Table S7. Quantitative analysis of ubiquitinated proteins.
Supplemental Table S8. IP-MS data of GF14-4.
Supplemental Table S9. Primers used in this research.
Acknowledgments
We are grateful to the Mass Spectrometry Laboratory of China Agriculture University for its help in the IP-MS experiment.
Funding
This work was supported by the National Natural Science Foundation of China (31971957; 91935303) and the National Key Research and Development Program of China (2021YFD1200700).
References
Author notes
These authors contributed equally to this article.
J.X., Z.Y., and M.Z. designed the experiments. J.X., Z.Y., and X.F. performed the experiments. J.X., W.S., Q.Z., and J.L. wrote the manuscript. Y.C. and X.Z. screened the mutant hsp90.6. K.T. analyzed the RNA-seq data. L.E. and H.Z. revised the manuscript. The authors declare that there are no conflicts of interest.
The author responsible for distribution of materials integral to the findings presented in this article in accordance with the policy described in the Instructions for Authors (https://dbpia.nl.go.kr/plphys/pages/General-Instructions) is Weibin Song ([email protected]).
Conflict of interest statement. There are no conflicts of interest in the conclusions, implications and opinions of our work and article.