-
PDF
- Split View
-
Views
-
Cite
Cite
Ihsan Muhammad, Li Yang, Shakeel Ahmad, Saqib Farooq, Ahmad Khan, Nisar Muhammad, Saif Ullah, Muhammad Adnan, Shamsher Ali, Qing Ping Liang, Xun Bo Zhou, Melatonin-priming enhances maize seedling drought tolerance by regulating the antioxidant defense system, Plant Physiology, Volume 191, Issue 4, April 2023, Pages 2301–2315, https://doi.org/10.1093/plphys/kiad027
- Share Icon Share
Abstract
Drought stress (DS) challenges sustainable agriculture production by limiting crop growth and development. The objective of the study was to evaluate the effect of melatonin-priming on enzymatic and non-enzymatic antioxidant defense mechanisms and its relation with leaf ultrastructure and stomatal traits in maize (Zea mays L) seedlings under DS (PEG-6000). DS drastically decreased seed germination, plant growth, and leaf chlorophyll content due to excessive reactive oxygen species (ROS) production. Melatonin-priming significantly (P < 0.05) increased seed germination, root length, shoot length, fresh seedling weight, proline content, total soluble protein content, sugar content, chlorophyll content, and stomatal aperture size by 101%, 30%, 133%, 51%, 22%, 59%, 54%, 20%, and 424%, compared to no priming (NP) under DS, respectively. Similarly, priming improved leaf ultrastructure and reduced the amount of chlorophyll loss and oxidative damage in maize seedlings. Melatonin seed priming with 500 µM melatonin (M2) greatly increased superoxide dismutase (SOD), peroxidase (POD), catalase (CAT), glutathione, and ascorbate (AsA) activity, by 65%, 63%, 94%, 41%, and 55% compared to NP under DS and by 0.26%, 8%, 33%, 42%, and 15% under no-stress (NS), respectively. Melatonin-priming also reduced malondialdehyde content, electrolyte leakage, hydrogen peroxide (H2O2) content, and superoxide anion () content by 26%, 31%, 31%, and 33% compared to NP under DS and by 8%, 18%, 10%, and 11% under NS, respectively. In response to DS, melatonin-priming also stabilized the chloroplast structure, sustained cell expansion, protected cell walls, and greatly improved stomatal traits, including stomatal number, length, and width. Our results suggest that melatonin-priming improves drought tolerance in maize seedlings by alleviating the negative effect of ROS.
Introduction
Maize (Zea mays L.) is one of the major cereal crops in China, with a large planting area and high yield (Daryanto et al., 2016). It is a staple food in many countries around the World and is grown under different climatic conditions (Al-Tawaha et al., 2018). At the same time, it is a versatile crop that is used as feed for poultry and cattle, food for humans, and raw material (biofuel) in industries (Chen et al., 2018). Maize is of great interest because it can persist in harsh environmental conditions. However, adverse environmental and climatic conditions could affect the growth, development, and productivity of the maize crop (Lv et al., 2015; Ma et al., 2021). As a severe environmental setback, drought is linked to changes in surface hydrological processes and climate (Leng et al., 2015; Khan et al., 2019). Drought is a naturally occurring phenomenon in all regions of the World; over the last decade, the average grain yield decreased by drought stress (DS) was about 14.70% in China (Khan et al., 2019; Guo et al., 2020).
DS is one of the most important environmental variables limiting crop development and productivity (Muhammad et al., 2022b). It is a major abiotic stress that has had a substantial detrimental impact on maize productivity and decreased global maize production by 40% over the last 25 years (Zhang et al., 2015a, 2015b). DS has a detrimental impact on plant physiological and biochemical metabolic processes, for example, it can cause early stomatal closure, photosynthetic damage, degradation of cell membrane integrity, and, eventually, decreased crop production (Campos et al., 2014; Wang et al., 2021a, 2021b). Photosynthesis is the most susceptible physiological function to DS because it relies on particular components for electron transport (Ahmad et al., 2021a, 2021b). In addition, Wang et al. (2021a, 2021b) reported that stomatal and non-stomatal restrictions under DS reduced plant photosynthetic rates. Abscisic acid is generated in the roots during DS and sends signals to the shoots, causing stomatal closure. This may reduce the intracellular carbon dioxide concentration, which in turn may reduce the photosynthetic rate. DS affects chloroplast structure, the electron transport chain, and carbon assimilation-related enzyme activity, which decreases photosynthetic efficiency and causes an excess of reactive oxygen species (ROS) to accumulate (Campos et al., 2014; Meng et al., 2014). Antioxidant defense mechanisms in plants include enzymatic and non-enzymatic activities that scavenge ROS from cells (Deng et al., 2017; Guo et al., 2020). However, under severe DS, the inherent defense capacity is reduced. Therefore, an effective management strategy is needed to improve the plant antioxidant defense system, increase drought resistance, and decrease ROS production. Using plant growth regulators, such as auxins, gibberellins, cytokinins, abscisic acid, and melatonin, is the most successful and effective method.
Pre-sowing practices such as seed priming involve exposing seeds to an environment with a lower water potential (Khan et al., 2019). It has been demonstrated that seed priming improves the plant capacity to endure stress during subsequent growth and development phases (Khan et al., 2019). Despite the difficulty of studying the germination process, priming is assumed to start metabolic activities required for embryonic growth and radical projection (Khan et al., 2019). Previous research showed that primed seeds outperformed non-primed seeds in germination ability, uniform seedling emergence, and stress tolerance under various adverse environmental conditions (Abid et al., 2018; Habiba et al., 2019). During DS conditions, the rate of CO2 acclimatization reduces; as a result, the decreasing power rate in the Calvin cycle becomes greater than the consumption rate. As reductant molecules are assimilated during the formation of the electron transport chain in photosystems, a large number of ROS are produced (Khan et al., 2019). The high ROS production under DS conditions significantly (P < 0.0) damages the cell membrane, lipids, nucleic acids, and proteins, resulting in lipid peroxidation, protein denaturation, and DNA alteration (Abid et al., 2018; Khan et al., 2019). Plant cells have evolved protective mechanisms that include both enzymatic and non-enzymatic activities (Muhammad et al., 2022b). The accumulation of metabolites such as glycine betaine, soluble sugars, soluble proteins, and proline promotes plant tolerance to DS (Khan et al., 2019). Hormonal treatment is often used to alleviate salt, drought, chilling, and other abiotic stressors (Ahmad et al., 2021a, 2021b; Muhammad et al., 2022b).
Plant growth regulators such as salicylic acid (Farhadi and Ghassemi-Golezani, 2020), proline (Youssef et al., 2020), and melatonin (Muhammad et al., 2022b) have been recently utilized in order to alleviate the detrimental effects of abiotic stress on plants. Chemically, melatonin is acknowledged as N-acetyl-5-methoxy-tryptamine, which is one of the neurohormones of living organisms and was discovered and quantified in plants in 1995 (Khan et al., 2019). Melatonin has critical regulatory roles in abiotic stressors such as drought, salinity, low and high ambient temperatures, UV radiation, and toxic compounds (Okant and Kaya, 2019; Bawa et al., 2020; Ahmad et al., 2021a, 2021b; Sezer et al., 2021). Melatonin protects plants from oxidative stress by promoting their growth, photosynthetic activity, and chlorophyll content while decreasing ROS formation (Kul et al., 2019). The role of melatonin in plants is still unknown, and researchers are debating whether it acts as an antioxidant or a growth regulator (Arnao and Hernández-Ruiz, 2014). Melatonin appears to have auxin activity as a growth regulator, regulating both root and shoot development (Fleta-Soriano et al., 2017). Melatonin also plays a role in fruit development and senescence retardation (Gao et al., 2016; Fleta-Soriano et al., 2017). In addition, seed priming with melatonin has been shown to improve drought tolerance and crop yield and melatonin has been shown to have a higher antioxidant capacity than vitamins E, C, and K in some systems (Tan et al., 2007). These functions make it extremely useful in agronomic applications aimed at increasing crop tolerance to cold, drought, heat, chemical pollutants, and other abiotic stressors and ultimately increasing crop productivity (Alharby and Fahad, 2020; Li et al., 2021). Moreover, seed priming with melatonin improves seed germination, stimulates root growth and development, and protects plant photosynthetic rate under stress conditions (Jiang et al., 2016; Abid et al., 2018).
While volumetric studies suggest that melatonin can improve drought resistance, the exact implementation and mechanisms involved in crop drought tolerance remain unknown. Many environmental stresses can substantially impact the germination of seeds and the survival of seedlings. A variety of substances have been found to have beneficial effects in research studies. Previous studies used melatonin as a foliar application, as a soil drench, and/or mixed it with other nutrient solutions. However, there is a lack of studies examining the seed priming effect on polyethylene glycol (PEG)-induced DS in a controlled environment (growth chamber). We hypothesized that seed priming with exogenous melatonin might be an appropriate, cost-effective, and realistic approach under biotic and abiotic stress conditions. The objectives of this study were to investigate the optimum melatonin level for maize development and its influence on seed germination; physiological, morphological, and biological activities; and stomatal and cellular ultrastructure traits in maize seedlings.
Results
Melatonin-priming enhances germination rate, characteristics, and seedling growth
In the current study, melatonin and hydro-priming (HP) were utilized as pre-sowing treatments. Three different melatonin concentrations were used to simulate the proposed treatments (250, 500, and 1,000 µM). Seed priming significantly (P < 0.05) affected maize germination potential and rate (Figure 1). DS induced by PEG-6000 (DS) significantly (P < 0.05) reduced the seed germination potential and rate of NP seeds compared with that of NP + NS seeds, which had a germination rate of 99%. The seed germination rate was 47%, 79%, 101%, and 61% greater in HP + DS, M1 + DS, M2 + DS, and M3 + DS compared to in NP + DS, respectively (Figure 1). Melatonin-priming (M2) significantly increased root length (23.30 cm) in normal growing conditions (NS) compared with NP (Figure 2A). Furthermore, the root length was 107%, 101%, 117%, 169%, and 118% greater in NP + DS, HP + DS, M1 + DS, M2 + DS, and M3 + DS, respectively, compared to in NP + NS. The M2 + DS treatment increased root length by 30% and 34% compared to the NP + DS and HP + DS treatments, respectively, demonstrating that melatonin-priming is significantly and highly effective in DS environments (Figure 2A).
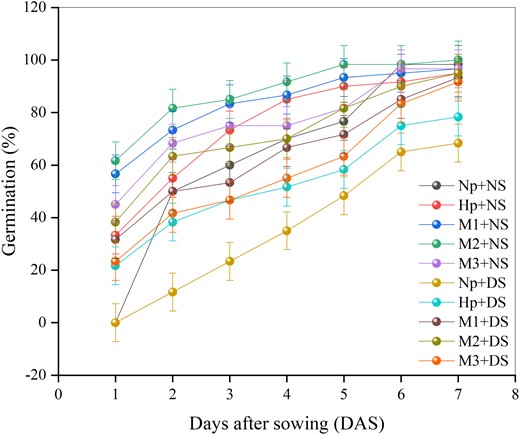
Effects of seed priming with different melatonin concentrations on maize seed germination under DS conditions. No priming (NP): unprimed seeds; hydro-priming (HP): primed in water only; no-stress (NS): no PEG-6000 was added; DS: 15% PEG-6000 was added; melatonin-priming (M1): 250 µM melatonin; melatonin-priming (M2): 500 µM melatonin; melatonin-priming (M3): 1000 µM melatonin. Data represent means of all replicates, and the vertical bars are the LSD values based on 0.05% probability values.

Effects of seed priming with different melatonin concentrations on root length, shoot length, root-shoot length ratio, and fresh seedling weight under DS conditions. (A) Root length, (B) shoot length, (C) root-shoot length ratio, and (D) fresh seedling weight. No priming (NP): unprimed seeds; hydro-priming (HP): primed in water only; no-stress (NS): no PEG-6000 was added; DS: 15% PEG-6000 was added; melatonin-priming (M1): 250 µM melatonin; melatonin-priming (M2): 500 µM melatonin; melatonin-priming (M3): 1000 µM melatonin. Data represent means ± Se of three replicates. Different letters indicate significant differences according to the LSD test (P < 0.05).
Maize shoot growth was significantly affected by melatonin treatments under stress conditions (Figure 2B). The shoot growth in the NP + DS treatment was reduced by 56% and 59% compared to that in the NP + NS and HP + NS treatments, respectively. Melatonin-priming reduced the negative effects of DS on shoot growth in maize compared with NP and HP (Figure 2B). In addition, the M2 + DS treatment resulted in 133% and 63% higher shoot lengths than the NP + DS and HP + DS treatments, respectively. Similar to root length, the root to shoot ratio was also significantly higher (P < 0.05) under DS conditions than that of the NS conditions (Figure 2C). Melatonin-priming significantly (P < 0.05) increased the root-shoot ratio under DS conditions compared to that under no-stress. However, the M1 + DS and M2 + DS treatments markedly decreased the root to shoot ratio compared to NP + DS and HP + DS. Seeds primed with melatonin had significantly greater fresh seedling weights compared to seeds with no priming or HP under both no-stress and DS conditions (P < 0.05). Moreover, the M1 + NS, M2 + NS, and M3 + NS treatments under no-stress conditions increased fresh seedling weight by 12%, 26%, and 24% compared to NP + NS treatment, respectively (Figure 2D). The M2 + DS treatment had a significantly higher fresh seedling weight (71 mg), and NP + DS had a significantly lower fresh seedling weight (47 mg) compared to other treatments. Averaged across all the treatments (NP, HP, and melatonin-priming), the NS condition resulted in a significantly (P < 0.05) higher fresh seedling weight compared to the DS condition (Figure 2D).
Melatonin-priming reduces the oxidative damage in maize seedlings under DS
DS-induced oxidative damage was estimated by measuring the contents of H2O2, , MDA, and proline. Hydrogen peroxide is an ROS that is formed by plant cells in response to stressful environmental conditions. The HP + NS, M1 + NS, M2 + NS, and M3 + NS treatments reduced the H2O2 concentration by 4.65%, 5.44%, 10.31%, and 6.50%, and the concentration by 3.14%, 6.68%, 11.13%, and 10.10%, respectively, compared to the NP + NS treatment (Figure 3, A and B). Hydrogen peroxide and content increased significantly in the NP + DS treatment compared to other treatments. Although HP + DS, M1 + DS, M2 + DS, and M3 + DS treatments significantly decreased the quantity of H2O2 and compared to NP + DS, the lower ROS levels in water deficit areas might be due to melatonin pretreatment. The M2 + DS treatment decreased the H2O2 content by 10.31% and 5.93% and by 11.13% and 8.25% compared to NP + DS and HP + DS, respectively.
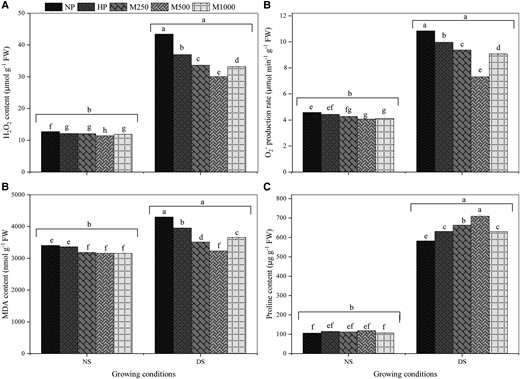
Effects of seed priming with different melatonin concentrations on H2O2, O2− production, MDA content, and proline content under DS conditions. (A) H2O2, (B) O2− production, (C) MDA content, and (D) proline content. No priming (NP): unprimed seeds; hydro-priming (HP): primed in water only; no-stress (NS): no PEG-6000 was added; DS: 15% PEG-6000 was added; melatonin-priming (M1): 250 µM melatonin; melatonin-priming (M2): 500 µM melatonin; melatonin-priming (M3): 1000 µM melatonin. Data represent means ± Se of three replicates. Different letters indicate significant differences based on LSD test (P < 0.05).
Malondialdehyde (MDA) content was used to determine the role of ROS in oxidative damage and lipid peroxidation in stressed maize seedlings. Overall, NS decreased the MDA content by 13% compared to DS; however, in both NS and DS conditions, melatonin-priming was significantly more effective than HP. The M2 + NS treatment significantly decreased the MDA content by 7% compared to NP + NS, and M2 + DS decreased the MDA content by 25% compared to NP + DS treatment (Figure 3C). These results showed that melatonin-priming is much more effective under DS than under NS conditions. Under NS conditions, the melatonin-priming had no significant effect on proline content. However, all the melatonin-priming treatments significantly increased the proline content compared to NP + DS treatment (Figure 3D). Interestingly, M1 + DS and M2 + DS significantly increased the proline content by 14% and 22%, respectively, compared to NP + DS treatment. Moreover, the HP + DS treatment also resulted in higher proline content compared to NP + DS but was statistically similar to the M3 + DS treatment (Figure 3D).
Melatonin-priming increases antioxidant activity in maize seedlings under DS conditions
The melatonin had no significant effect on SOD, POD, and CAT activities under no-stress conditions. However, under PEG-induced DS conditions, the melatonin treatments significantly stimulated the activities of SOD, POD, and CAT (Figure 4). The M2 + DS treatment had considerably higher activities compared to the NP + DS, HP + DS, M1 + DS, and M3 + DS treatments. The M2 + DS treatment had 65%, 35%, 23%, and 21% higher SOD activity; 63%, 39%, 19%, and 29% higher POD activity; and 94%, 38%, 20%, and 31% higher CAT activity compared to NP + DS, HP + DS, M1 + DS, and M3 + DS treatments, respectively (Figure 4, A–C). Averaged across all priming treatments, SOD, POD, and CAT activities were greater in DS than in NS (Figure 4, A–C). The changes in antioxidant enzymes appear to be directly related to the oxidative damage caused by DS. The HP + DS treatment also increased SOD (22%), POD (17%), and CAT (40%), compared to NP + DS (Figure 4, A–C). Glutathione and AsA (non-enzymatic)antioxidant activities followed the same patterns as the variations in antioxidant enzyme activity. Compared to NS, the accumulation of GSH and AsA activities were significantly higher under DS conditions (Figure 4, D and E). The GSH activity was substantially increased by 41%, 31%, 20%, and 26% in the M2 + DS treatment compared to in the NP + DS, HP + DS, M1 + DS, and M3 + DS treatments, respectively (Figure 4, D and E). Similarly, the GSH activity was significantly increased by 42%, 20%, 15%, and 5% in M2 + NS treatment compared to in the NP + NS, HP + NS, M1 + NS, and M3 + NS treatments, respectively. Under no-stress conditions, AsA activity was not significantly affected by melatonin treatments. However, the AsA activity was dramatically increased by 55% and 49% in M2 + DS compared to in NP + DS and HP + DS treatments, respectively (Figure 4E).
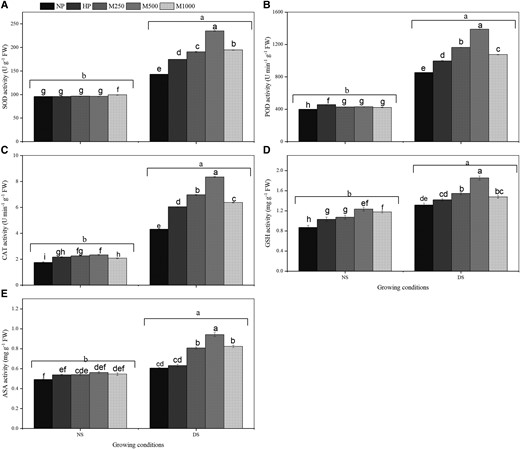
Effects of seed priming with different concentration of melatonin on SOD activity, POD activity, CAT activity, GSH activity, and ASA activity under DS conditions. (A) SOD activity (B) POD activity (C) CAT activity (D) GSH activity, and (F) ASA activity. No priming (NP): unprimed seeds; hydro-priming (HP): primed in water only; no-stress (NS): no PEG-6000 was added; DS: 15% PEG-6000 was added; melatonin-priming (M1): 250 µM melatonin; melatonin-priming (M2): 500 µM melatonin; melatonin-priming (M3): 1000 µM melatonin. Data represent means ± Se of three replicates. The number of germinated seeds was counted daily and the process germination was terminated 15 days after sowing (DAS). Bars represent ± Se of three replicates. Difference in letters indicates significant differences according to LSD test (P < 0.05).
Melatonin-priming increases total soluble protein and soluble sugar
Soluble proteins and sugars are essential osmolytes that equip plants to tolerate oxidative damage under abiotic stress. The M2 + NS treatment significantly increased the total soluble protein by 38% and 30% and total soluble sugar by 38% and 20% in maize seedlings compared to NP + NS and HP + DS treatments, respectively (Figure 5, A and B). Intriguingly, the total soluble protein content increased in NP + DS, HP + DS, M1+ DS, M2+ DS, and M3+ DS by 65%, 105%, 125%, 154%, and 111%, respectively, and total soluble sugar content increased by 78%, 120%, 145%, 184%, and 148%, respectively, compared to in the NP + NS treatment. Total soluble protein and total soluble sugar showed a similar trend across priming treatments under both no-stress and DS conditions. M2 + NS significantly increased total soluble protein content (18% and 17%) and total soluble sugar content (15% and 20%) compared to M1 + NS and M3 + NS treatments (Figure 5, C and D). In addition, the total soluble sugar and total soluble protein were significantly higher in all melatonin-priming treatments than in NP + NS and HP + NS treatments. Averaged across priming treatments, the DS resulted in higher total soluble protein and total soluble sugar compared to no-stress conditions (Figure 5, A and B).
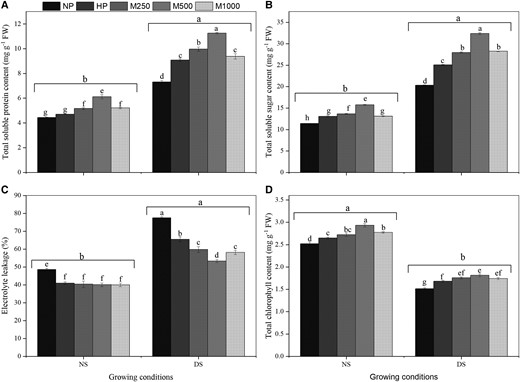
Effects of seed priming with different melatonin concentrations on total soluble protein, total soluble sugar, EL, and total chlorophyll content under DS conditions. (A) Total soluble protein, (B) total soluble sugar, (C) EL, and (D) total chlorophyll content. No priming (NP): unprimed seeds; no-stress (NS): hydro-priming (HP): primed in water only; no PEG-6000 was added; DS: 15% PEG-6000 was added; melatonin-priming (M1): 250 µM melatonin; melatonin-priming (M2): 500 µM melatonin; melatonin-priming (M3): 1000 µM melatonin. Data represent means ± Se of three replicates. Different in letters indicate significant differences according to the LSD test (P < 0.05).
Melatonin-priming improves electrolyte leakage level and chlorophyll content
The conductivity of solute leakage from the cells was used to determine the level of membrane damage. Our results showed that the electrolyte leakage (EL) was markedly higher under DS compared to under NS (Figure 5C). The EL was significantly increased by 18%, 30%, 45%, and 33% in NP + DS compared to HP + DS, M1 + DS, M2 + DS, and M3 + DS treatments. Furthermore, the EL under DS was significantly lower in HP + DS, M1 + DS, M2 + DS, and M3 + DS compared to NP + DS, but no significant differences were found between M1 + DS (59.80 mg g−1 FW) and M3 + DS (58.20 mg g−1 FW) treatments. Under the NS condition, no significant differences in EL were observed among the priming treatments except NP had significantly higher EL than the other priming treatments. The leaf chlorophyll content in maize seedlings was significantly affected by priming and stress condition (Figure 5D). Compared to NS, DS significantly decreased the chlorophyll content in maize seedlings. However, the reduction of chlorophyll content under DS conditions was markedly lower in M2 + DS treatment compared to NP + DS, HP + DS, M1 + DS, and M3 + DS treatments, and non-significantly different from M1 + DS and M3 + DS treatments. The leaf chlorophyll content was significantly increased by 11%, 16%, 20%, and 15% in HP + DS, M1 + DS, M2 + DS, and M3 + DS compared to NP + DS treatment. There were no significant differences among M1 + DS, M2 + DS, and M3 + DS treatments, but the M2 + NS treatment had significantly higher chlorophyll content than other priming treatments under NS.
Effect of melatonin-priming on leaf ultrastructure
Several processes contribute to plant growth and development, where cell division and elongation are vital. Under DS, cell size decreased in NP treatment compared to in treatments with melatonin-priming (Figure 6, A–D). M1 + DS and M2 + DS treatments resulted in more stable and robust cell expansion compared to NP + DS treatment. Changes in chloroplast structure are associated with chlorophyll synthesis for photosynthesis. The chloroplast was elliptical in shape, well-organized, and adherent to the cell wall in NP + NS treatment (Figure 6A), but the chloroplast was deformed in NP + DS treatment (Figure 6B). Both M1 + DS and M2 + DS treatments alleviated this damage to chloroplasts, resulting in well-arranged, clear, and complete edges that clung to the cell wall (Figure 6, C and D). The thick and well-formed cell walls with precise edges were observed in NP + NS (Figure 6, E and I), M1 + DS (Figure 6, G and K), and M2 + DS treatments (Figure 6, H and L). No priming under DS decreased the chloroplast length compared to melatonin-priming treatments and resulted in cell walls with no clear edges (Figure 6j).
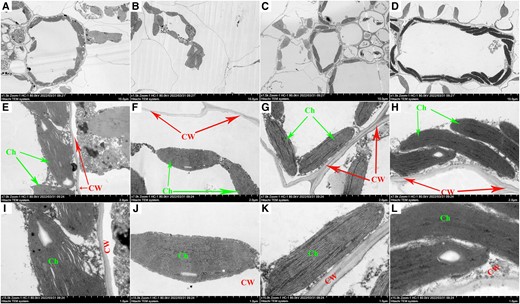
Effects of melatonin-priming on leaf ultrastructure of maize leaves cells, chloroplasts and cell wall under PEG-6000 simulated DS (15% PEG) compared to no-priming treatments under normal (0% PEG). Figures A, B, C, and D represent the effect of NP + NS, NP + DS, M1 + DS and M2 + DS treatments on cells (a–d, ×1,500 magnification, scale bars = 10 μm). Figures E, F, G, and H represent the effect of NP + NS, NP + DS, M1 + DS and M2 + DS treatments on ultrastructure changes in chloroplasts and cell wall (E–H, ×7,000 magnification, scale bars = 2 μm). Similarly, I, J, K, and L represent the effect of NP + NS, NP + DS, M1 + DS and M2 + DS treatments on ultrastructure changes in chloroplasts and cell wall (i–k, ×15,000 magnification, scale bars = 1 μm) CW, cell wall and Ch, chloroplast.
Melatonin-priming improves stomatal traits in maize seedlings
Analyses of morpho-physiological and biochemical attributes suggest that M1 and M2 were more effective than NP and M3 treatments for alleviating DS. Based on these parameters, the leaf samples of NP + NS, NP + DS, M1 + DS, and M2 + DS treatments were selected for scanning electron microscope (SEM) and TEM analysis. These selected treatments were analyzed for stomatal number, length, width, and aperture. Stomata are the key passageways for transpiration and air exchange in plants. Compared to in NP + DS, the stomatal width and aperture were significantly higher in NP + NS treatment (Figure 7 and Table 1). However, the stomatal length and number were much higher in NP + DS compared to in NP + NS treatment, demonstrating that the DS significantly increased the stomatal number and length but decreased the width and aperture (Table 1). Compared to NP + NS, the stomatal numbers increased dramatically in HP + DS (200%), M1 + DS (97%), and M2 + DS (47%). These results suggest that the most effective treatment for increasing stomatal aperture was melatonin-priming under DS conditions. Moreover, these results showed that HP (HP + DS) significantly increased stomatal number and decreased stomatal length, width, and aperture under DS conditions compared to melatonin-priming (M1 + DS and M2 + DS). Interestingly, M1 + DS and M2 + DS decreased the stomatal number by 34.30% and 51.00%, respectively, but increased the stomatal length by 8.60% and 3.20%, width by 72.60% and 80.30%, and aperture by 75.00% and 81.00% compared to HP + DS (Table 1).

Effects of melatonin-priming on maize leaf stomatal number, length and width under PEG-6000 simulated DS (15% PEG) compared to no-priming treatments under normal (0% PEG). Figures a, b, c, and d represent the effect of NP + NS, NP + DS, M1 + DS and M2 + DS treatments on the stomatal number. a–d, ×100 magnification, scale bars = 500 μm). Similarly, d, e, f, and g represent the effect of NP + NS, NP + DS, M1 + DS and M2 + DS treatments on stomatal length and width. e–h, ×3,000 magnification, scale bars = 10 μm).
Effect of seed priming with melatonin on stomatal traits of maize seedling under DS conditions
Treatment . | Stomatal length (μm) . | Stomatal width (μm) . | Stomatal aperture (μm) . | Stomatal number . |
---|---|---|---|---|
NP + NS | 20.76 ± 0.40 c | 1.28 ± 0.06 a | 26.50 ± 1.71 a | 34 ± 0.58 d |
NP + DS | 24.46 ± 0.40 b | 0.20 ± 0.04 d | 4.88 ± 0.28 c | 102 ± 2.31 a |
M1 + DS | 26.77 ± 0.40 a | 0.73 ± 0.06 c | 19.53 ± 1.85 b | 67 ± 1.73 b |
M2 + DS | 25.28 ± 0.25 b | 1.01 ± 0.06 b | 25.61 ± 1.40 a | 50 ± 2.89 c |
Treatment . | Stomatal length (μm) . | Stomatal width (μm) . | Stomatal aperture (μm) . | Stomatal number . |
---|---|---|---|---|
NP + NS | 20.76 ± 0.40 c | 1.28 ± 0.06 a | 26.50 ± 1.71 a | 34 ± 0.58 d |
NP + DS | 24.46 ± 0.40 b | 0.20 ± 0.04 d | 4.88 ± 0.28 c | 102 ± 2.31 a |
M1 + DS | 26.77 ± 0.40 a | 0.73 ± 0.06 c | 19.53 ± 1.85 b | 67 ± 1.73 b |
M2 + DS | 25.28 ± 0.25 b | 1.01 ± 0.06 b | 25.61 ± 1.40 a | 50 ± 2.89 c |
Effects of seed priming on maize leave stomatal length, stomatal width, stomatal aperture, and stomatal number under DS conditions. No priming (NP): unprimed seeds; no-stress (NS): no PEG-6000 was added; DS: 15% PEG-6000 was added; melatonin-priming (M1): 250 µM melatonin; melatonin-priming (M2): 500 µM melatonin. Data represent means ± SE of three replicates. Different letters indicate significant differences according to LSD test (P < 0.05).
Effect of seed priming with melatonin on stomatal traits of maize seedling under DS conditions
Treatment . | Stomatal length (μm) . | Stomatal width (μm) . | Stomatal aperture (μm) . | Stomatal number . |
---|---|---|---|---|
NP + NS | 20.76 ± 0.40 c | 1.28 ± 0.06 a | 26.50 ± 1.71 a | 34 ± 0.58 d |
NP + DS | 24.46 ± 0.40 b | 0.20 ± 0.04 d | 4.88 ± 0.28 c | 102 ± 2.31 a |
M1 + DS | 26.77 ± 0.40 a | 0.73 ± 0.06 c | 19.53 ± 1.85 b | 67 ± 1.73 b |
M2 + DS | 25.28 ± 0.25 b | 1.01 ± 0.06 b | 25.61 ± 1.40 a | 50 ± 2.89 c |
Treatment . | Stomatal length (μm) . | Stomatal width (μm) . | Stomatal aperture (μm) . | Stomatal number . |
---|---|---|---|---|
NP + NS | 20.76 ± 0.40 c | 1.28 ± 0.06 a | 26.50 ± 1.71 a | 34 ± 0.58 d |
NP + DS | 24.46 ± 0.40 b | 0.20 ± 0.04 d | 4.88 ± 0.28 c | 102 ± 2.31 a |
M1 + DS | 26.77 ± 0.40 a | 0.73 ± 0.06 c | 19.53 ± 1.85 b | 67 ± 1.73 b |
M2 + DS | 25.28 ± 0.25 b | 1.01 ± 0.06 b | 25.61 ± 1.40 a | 50 ± 2.89 c |
Effects of seed priming on maize leave stomatal length, stomatal width, stomatal aperture, and stomatal number under DS conditions. No priming (NP): unprimed seeds; no-stress (NS): no PEG-6000 was added; DS: 15% PEG-6000 was added; melatonin-priming (M1): 250 µM melatonin; melatonin-priming (M2): 500 µM melatonin. Data represent means ± SE of three replicates. Different letters indicate significant differences according to LSD test (P < 0.05).
Discussion
DS is one of the abiotic stresses that is gaining attention due to its detrimental impact on plant morphological and physiological characteristics, crop yields and biomass, and global food security (Arslan et al., 2018; Rimdusit et al., 2019). Seed priming is one of the most effective techniques to promote germination rate and seedling growth under DS conditions. Melatonin plays a key role in a number of different physiological processes in plants, including acting as an antioxidant against oxidative stress (Arnao and Hernández-Ruiz, 2015; Cao et al., 2021). Improving plant tolerance to harmful environmental circumstances requires external melatonin application (Alharby and Fahad, 2020; Kamiab, 2020). The current study results were also supported by Khan et al. (2019), who demonstrated that melatonin-primed seeds dramatically boosted seedling germination rate, physiological characteristics, and enzymatic activities. Plant growth regulators and phytohormones such as polyamines (Boga et al., 2019), gibberellins (Ren et al., 2020), salicylic acid, and abscisic acid control the complex physiological and biochemical process of seed germination (Farhadi and Ghassemi-Golezani, 2020; Li et al., 2021).
Seed priming with exogenous melatonin has been demonstrated to minimize the adverse impacts of drought and cold stress (Chang et al., 2021). The results of the current study suggested that melatonin and hydro primed seeds not only increased seed germination potential and rate (Figure 1), but also improved seedling growth as shown by longer root length, root and shoot biomass, and root-shoot ratio when compared to those of the NP + NS seeds (Figure 2, A–D). Our results are consistent with earlier research (Javeed et al., 2021), which showed that exogenous melatonin application stimulated root growth by increasing endogenous levels of free indoleacetic acid in the roots. According to Simlat et al. (2018), treatments with greater doses of melatonin had a clear inhibitory effect, while low doses considerably improved seed germination and characteristics. Furthermore, Chang et al. (2021) demonstrated that exogenous melatonin improved abscisic acid concentrations and had an alleviating effect on drought-primed plants when exposed to cold stress.
DS causes ROS imbalance and affects plant growth, enzymatic activities, stomatal traits, chloroplasts, photosynthesis, and cell membrane integrity (Li et al., 2021). Results showed that under DS conditions, practically all growth indices declined dramatically. However, melatonin-priming greatly mitigated the decline in shoot and root length and fresh seedling weight (Figure 2). According to recent research, melatonin acts as a growth regulator and is associated with several abiotic stresses in plants (Zafar et al., 2019; Wang et al., 2021a, 2021b). It also promotes the growth of wheat and maize in saline conditions (Zafar et al., 2019; Wang et al., 2021a, 2021b), maize under chilling stress (Kolodziejczyk et al., 2021), and maize and wheat under DS conditions (Lv et al., 2017; Ahmad et al., 2021a, 2021b). Our results showed that 500 µM exogenous melatonin under drought (M2 + DS) and no-stress (M2 + NS) efficiently improved fresh seedling weight, shoot length, and root length compared to other treatments. It was proposed that exogenous melatonin has the capacity to partially ameliorate the DS restricted growth and development in maize seedlings.
Melatonin acts as a plant growth regulator by scavenging ROS and reducing the negative effects of environmental stress (Boga et al., 2019; Huang et al., 2019). The results of this investigation showed that melatonin protects maize seedlings from the detrimental effects of drought. Furthermore, these results suggest that pretreatment of maize seeds with melatonin activated the antioxidant defense mechanisms. Oxidative stress and excessive accumulation of ROS result from the disruption of the photosynthetic electron cycle in plants during DS, leading to damage to certain organelles (Ye et al., 2016; Huang et al., 2019). Similarly, in the present study, H2O2, , MDA, and proline content production were significantly higher under DS conditions compared to under no-stress. However, our results showed that the DS-induced ROS production was significantly decreased with melatonin-priming (M2 + DS) compared to with no-priming and HP treatments (Figure 3, A–C). The reduction in ROS might be due to the sensitivity of maize seedlings in melatonin-primed treatment (M2 + DS), which subsequently activates the antioxidant enzymes to cope with the excessive ROS. Our results are supported by previous researcher showing that exogenous melatonin substantially increased ROS production under chilling (Kolodziejczyk et al., 2016), drought (Ahmad et al., 2021a, 2021b), and salinity stress (Sezer et al., 2021).
The antioxidant enzyme system must be active to counteract the effects of ROS. In particular, the SOD, POD, CAT, GSH, and ASA activities are crucial antioxidant systems in plants that can stabilize ROS levels in chloroplasts (Byeon et al., 2014; Alharby and Fahad, 2020). In our study, the activities of SOD, POD, CAT, GSH, and ASA under DS were markedly increased with melatonin-priming compared to with NP and HP, which indicates that these antioxidants might be implicated in quenching the ROS in stress environments. These results demonstrated that melatonin-priming had a significant impact on the biosynthesis of SOD, POD, CAT, GSH, and ASA. Previous studies have shown that exogenous melatonin regulates antioxidant enzymes, which is a natural plant response to oxidative stress generated by numerous biotic and abiotic stresses (Ashraf et al., 2017; Kolodziejczyk et al., 2021). The antioxidant enzyme activities in plants quench the production of ROS (Khaliq et al., 2015). Moreover, SOD is thought to be a major component in catalyzing dismutation, whereas POD and CAT are important in scavenging H2O2 (Ahmad et al., 2020; Alharby and Fahad, 2020). The enhancement of SOD activity in response to stressful environments is generally considered a defensive strategy against superoxide production (Muhammad et al., 2022b).
The findings of this study indicated that melatonin acted as a regulator by boosting the activity of related antioxidant enzymes and improving plant tolerance to oxidative stress. Moreover, under DS, melatonin-primed seedlings had considerably lower levels of MDA, H2O2, and compared to both no-primed and hydro-primed seedlings (Figure 3, A–C). Previous research revealed that using plant growth regulators could prevent plants from the oxidative damage caused by ROS (Deng et al., 2017; Campos et al., 2019). Higher antioxidant enzyme activity was linked to decreased ROS accumulation in melatonin-treated plants (Ahmad et al., 2020). Melatonin-treated plants had greater POD and CAT activities, dramatically lowering H2O2 concentrations. These enzymes convert the stable free radical H2O2 into water and oxygen (Li et al., 2021; Muhammad et al., 2022b). Melatonin-priming increases antioxidant enzyme activity while decreasing ROS accumulation under a stress environment (Khan et al., 2019; Muhammad et al., 2022b). These results are in line with those of recent studies (Kamran et al., 2018; Ahmad et al., 2021a, 2021b) that found a substantial reduction in H2O2 levels and an increase in POD and CAT enzyme activities following exogenous melatonin application in a stressful environment.
Total soluble sugar and protein levels decrease due to leaf senescence as the plants mature (Qiao et al., 2020; Ahmad et al., 2022a, 2022b). During leaf senescence, melatonin treatment considerably increases total soluble sugar and protein levels (Ahmad et al., 2020; Muhammad et al., 2022b). Exogenous melatonin also increases soluble sugar content, which reduces chlorophyll degradation and leaf senescence and dramatically increases plant photosynthetic capacity and yield (Ahmad et al., 2020, 2022a, 2022b). Melatonin-treated plants had increased chlorophyll content and lower leaf senescence, which was ascribed to lower ROS accumulation (Ahmad et al., 2020). Liang et al. (2018) reported that the decline in chlorophyll content is a sign of leaf senescence and lowers plant photosynthetic activity.
Due to chlorophyll breakdown and protein degradation, the photosynthetic ability of leaves decreases substantially under DS conditions, often triggering the development of premature leaf senescence (Kamran et al., 2020). Our results showed that total soluble protein, sugar, and total chlorophyll content were all significantly increased in melatonin-primed seedlings compared to NP in both NS and DS conditions, and the higher chlorophyll content led to a higher net photosynthetic rate (Qiao et al., 2020: Muhammad et al., 2022a). The maximum total soluble protein and sugar content were obtained with M2 + DS treatment compared to other treatments. Moreover under DS conditions M2 + DS treatment resulted in higher total chlorophyll content and lower EL than that of the HP and NP (Figure 5). According to Muhammad et al. (2022b), enhanced POD and APX activity in the melatonin-treated maize leaves under DS was associated with decreased EL level and MDA content, suggesting that oxidative damage under DS can be reduced with melatonin-priming. EL was extensively used to evaluate membrane permeability, and MDA content was widely recognized as a sign of oxidative damage (Zhang et al., 2014). Consistent with the findings of Ahmad et al. (2022a, 2022b) in maize seedlings, we found that melatonin-priming reduced MDA concentration, providing further evidence that melatonin could protect membranes against damage generated by DS.
One of the most detrimental effects of DS is a decrease in chlorophyll (Moghadam et al., 2020). It has been documented that DS has a negative impact on chlorophyll levels in rapeseed (Khan et al., 2019), grape cuttings (Meng et al., 2014), maize seedlings (Ahmad et al., 2022a, 2022b), cucumber (Zhang et al., 2013), and apples (Wang et al., 2013). The current study showed that DS significantly changed chloroplast and cell wall structure and increased ROS concentration. Melatonin, on the other hand, can efficiently protect the membrane structure and their biological activity to minimize further detrimental effects. There is some evidence that melatonin-priming increases the chlorophyll content in maize seedlings by mitigating the inhibitory effect of DS conditions on leaf pigmentation (Khan et al., 2019). Chlorophyll resides in chloroplasts, whose primary function is to carry out photosynthesis within plant cells (Wang et al., 2021a, 2021b). Chloroplasts are one of the most sensitive organelles to abiotic stress and are also the key site for ROS generation (Khan et al., 2019). Our results showed that well-established cells with clear and complete edges were observed in M2 + DS (Figure 6, C, G, and K) and M3 + DS (Figure 6, D, H, and I) but not in NP + DS (Figure 6, B, F, and J). Compared to NP + DS, M2 + DS had substantially larger chloroplasts (Figure 6, C, G, and K). These results suggest that melatonin-priming could mitigate the harmful effects of ROS on plant cell membranes by increasing the production of enzymatic and non-enzymatic antioxidants. Additionally, it is possible that the reduced osmotic potential of cells caused by the elevated levels of osmoprotectants in melatonin-primed seedlings, allows them to allure additional water and sustain a higher turgor potential, assisting in the development of a firm cell wall and well-established cell development. The results were consistent with a recent study showing that exogenous melatonin protects the internal lamellar system of chloroplasts and alleviates the ultrastructural damage caused by DS in rapeseed (Brassica napus; Khan et al., 2019).
Stomata serve as regulatory gates for the transportation of CO2 and transpiration, even though these activities are impacted by a variety of external conditions, including CO2 concentration, light, temperature, and water (Khan et al., 2019; Li et al., 2021). It is generally accepted that plants respond to DS by closing their stomata and reducing their ability to produce photosynthesis, which ultimately stunts their growth. The opening and closing of stomata, which regulate vital leaf physiological functions, including respiration, photosynthesis, and transpiration, are regulated by water balance and complicated signal transduction pathways (Ahmad et al., 2021a, 2021b; Wang et al., 2021a, 2021b). The reduction in photosynthetic rate under DS conditions is commonly attributed to the limiting of ambient CO2 diffusion to carboxylation caused by stomatal closure (Ye et al., 2016). Plants retain cellular moisture content under DS conditions by controlling stomatal closure and decreasing transpiration rate (Li et al., 2021). Our findings demonstrated that melatonin-priming resulted in higher stomatal numbers under DS conditions (Figure 7, C and D) compared to under no-stress (Figure 7A). In addition, the NP + DS had significantly higher stomatal numbers with small openings (lower stomatal length and width) compared to other treatments (Figure 7, A and D, and Table 1). These results signify that melatonin-priming increased the stomatal number under DS compared to NS conditions (Figure 7, A, C, and D) but lower than NP + DS (Figure 7B). Similarly, M2 + DS and M3 + DS resulted in wider stomatal lengths and widths (Figure 7, G and H) than NP + DS (Figure 7F). Increasing proline levels, soluble sugars, and soluble proteins, which lower the cell osmotic potential, are the key strategies that plants have to adapt under DS (Khan et al., 2019). Therefore, the melatonin-priming might have protected cell turgor during DS due to increased proline concentration, total soluble protein, and sugar accumulation. Our results demonstrated that melatonin-priming alleviated the inhibitory effect of DS on root and shoot growth, photosynthetic efficiency, stomatal conductance, and leaf ultrastructure.
Conclusions
Melatonin-priming moderately mitigated the growth inhibiting effects of DS in maize seedlings via decreasing the DS-induced ROS accumulation and activating the enzymatic (SOD, POD, and CAT) and non-enzymatic (GSH and AsA) antioxidant defense systems. Melatonin-priming greatly increased growth parameters, proline, total soluble protein, sugar, and chlorophyll contents. The leaf ultrastructure and stomatal analysis revealed that melatonin-priming protected the leaf chloroplast structure, cell wall, and significantly increased stomatal width, length, and numbers under PEG-6000 induced DS. Thus, seed priming with 500 µM melatonin could provide a valuable foundation for improving antioxidant enzyme activities, leaf chloroplasts, cell walls, stomatal traits, and seedling tolerance to DS.
Materials and methods
Study area, plant materials, and seed priming
The study was carried out in a controlled environment (growth chamber) at the Agriculture College of Guangxi University, Nanning, China, from December 15 to 30, 2021. Seeds of a widely cultivated hybrid maize (Zea mays) cultivar (Zhengda 619) were used in this study. The seeds were hand-picked to ensure they were healthy, free of debris, and spotless before being sterilized in 1% sodium hypochlorite (w/v) for 5 minutes and washed with distilled water. After sterilization, these seeds were initially dried using blotting paper and then air-dried at room temperature until they reached their initial weight. Five different melatonin-priming treatments were used to evaluate the negative effect of DS on maize seedlings.
Experimental design and treatment management
The experiment was carried out in a completely randomized design with three replications in a controlled environment in Guangxi, China. The experimental treatments labeled for this study included no-priming (NP), HP, priming with 250 µM melatonin (M1), priming with 500 µM melatonin (M2), and priming with 1,000 µM melatonin (M3) under no-stress (NS) and 15% PEG stress (DS). Melatonin solutions with concentrations of 250, 500, and 1,000 µM were freshly prepared, and seed priming was accomplished using a seed weight to volume of solution ratio of 1:5. Uniformly healthy seeds were selected and submerged in distilled water (0 µM melatonin) or 250, 500, and 1,000 µM melatonin concentrations at 25 °C in the dark for 6 h with mild steady agitation (Khan et al., 2019). The primed seeds were washed three times with distilled water, dried with blotted paper, and then air-dried using an oven at 25 °C until they reached their initial weight.
Growth conditions
The experimental treatments were maintained in the growth chamber at a temperature of 25/20 °C (day/night), a photoperiod of 14 h, and a relative humidity of 60%/70%. For germination, polyethylene boxes with 12 cm length, 6 cm width, and 6 cm height were used (Michel, 1983; Khan et al., 2019). PEG-6000 solutions of varied concentrations were placed in polyethylene boxes with three layers of sterile filter paper. Each box contained either 10 ml of distilled water (0 ml PEG solution) or 10 ml of PEG-6000 solution at different concentrations. In each germination box, 25 seeds were sown. Every other day throughout the experiment, two of the bottom filter papers were changed, and 7 ml of PEG-6000 solution at the appropriate concentration or distilled water were added to the respective germination boxes (Zhang et al., 2015a, 2015b; Khan et al., 2019). Germination rates were monitored daily, and seeds were considered to have germinated once the radicle reached 2 mm in length. The seedlings were harvested after 15 days of sowing, and root and shoot lengths were measured using 10 uniform seedlings from each treatment.
Experimental and analytical procedures
Photosynthetic pigments and metabolic content
The chlorophyll content was determined using the method of Arnon (1949) with a little modification. To prevent light from influencing the results, about 200 mg of leaf samples from each treatment were chopped up, and chlorophyll extraction was done with 80% (v/v) acetone. The supernatant was then removed and placed in a new tube, and the absorbance was measured at wavelengths of 663, 645, and 470 nm to determine the chlorophyll a and b content. As a blank control, 80% (v/v) acetone was used. The total soluble sugar was determined by milling about 0.10 g of fresh seedlings in 1 ml of distilled water. The total soluble sugar was then calculated using the anthrone technique with glucose as a standard (Lee and Kim, 2000; Khan et al., 2019). A Proline Assay Kit was used to measure the amount of proline in the sample. The proline absorbance was measured at 520 nm wavelength, and the proline content was described as µg g−1 FW.
Reactive oxygen species, MDA content, and EL
The superoxide anion () content was determined using a Assay Kit and a H2O2 Assay Kit was used to measure the concentration of H2O2. The absorbance at 532 nm was determined by collecting the upper pink supernatant. The absorbance of and H2O2 were measured at 532 and 415 nm, respectively. The and H2O2 content were expressed as µmol g−1 FW. MDA, a product of lipid peroxidation, was measured in plant leaves using the method described by Weisany et al. (2012). The EL was determined using the procedure described by Dionisio-Sese and Tobita (1998).
Determination of antioxidant enzyme activities
The antioxidant enzyme activity was determined by grinding 0.10 g of freshly harvested seedlings with 1 ml of phosphate buffer solution (50 mM; pH 7.80). To collect the supernatant, the homogenate was centrifuged at 12,000 × g for 20 min at 4 °C. The activities of SOD, POD, and CAT were measured using the protocols described by Aebi (1984) and Zhang (1992).
Determination of non-enzymatic activities
To determine the ascorbate and GSH activities, 0.10-g samples of fresh seedlings were milled with 1 ml of 10% (w/v) trichloroacetic acid and the mixture was centrifuged at 12,000 × g for 20 min at 4 °C. The supernatant was collected in a 2 ml tube after centrifugation. The standard methods previously described by Griffith (1980) were used to calculate the AsA and GSH contents.
Scanning electron microscopy and transmission electron microscopy analysis
To ensure sample uniformity, the middle parts of leaves from 1 to 2 mm were selected from three healthy seedlings. In order to clean the leaf samples, distilled water was used before being examined under an electron microscope. The samples were fixed (6 h, 4 °C) with 4% (v/v) glutaraldehyde and 0.20 M sodium phosphate buffer (pH 6.80). The samples were then washed four times with a 0.10 M sodium phosphate buffer with a pH of 6.80. Samples were rinsed twice with isoamyl acetate, serially diluted with ethanol, and freeze-dried. The fragments were firmly fixed on stubs with double-sided tape before being sputter-coated with gold (Kong et al., 2013). A JEOLJSM-6390LV Scanning Electron Microscope was used to examine the samples. For transmission electron microscope (TEM), the samples were postfixed in 1% (w/v) osmic acid in 0.20 M phosphate buffer (pH 6.80), and critical point drying was used to dry samples after being dehydrated in a graduated series of ethanol. Finally, the ultrathin segments were examined under a Hitachi 500 electron microscope after being stained with lead citrate and 2% (w/v) uranyl acetate (Kong et al., 2013).
Acknowledgments
The authors express their special gratitude to Prof. Dr. Hai Yan Wu for her strong support in experiment.
Funding
This study was financially supported by the Natural Science Foundation of Guangxi Province (2019GXNSFAA185028) and the Special Fund for Guangxi Innovation Team Construction of National Modern Agricultural Industrial Technology System (nycytxgxcxtd-2021-04-03).
Availability of data and materials
The entire dataset used and/or analyzed in the study is available from the corresponding author.
References
Author notes
These authors contributed equally.
Conceptualization and methodology: I.M. Formal analysis: I.M. and L.Y. Investigation: S.A., S.F., N.M., S.U., M.A. and Q.P.L. Resources: X.B.Z. Data curation: I.M. and A.K. Writing-original draft preparation: I.M. Writing, review, and editing: A.K. and S.A. Supervision: X.B.Z. All authors have read and agreed to the published version of the manuscript.
The author responsible for distribution of materials integral to the findings presented in this article in accordance with the policy described in the Instructions for Authors (https://dbpia.nl.go.kr/plphys/pages/General-Instructions) is: Xun Bo Zhou ([email protected]).
Conflict of interest statement. None declared.