-
PDF
- Split View
-
Views
-
Cite
Cite
Yongming Duan, Min Han, Maja Grimm, Jessica Ponath, Michael Reichelt, Axel Mithöfer, Adam Schikora, Combination of bacterial N-acyl homoserine lactones primes Arabidopsis defenses via jasmonate metabolism, Plant Physiology, Volume 191, Issue 3, March 2023, Pages 2027–2044, https://doi.org/10.1093/plphys/kiad017
- Share Icon Share
Abstract
N-acyl homoserine lactones (AHLs) are important players in plant–bacteria interactions. Different AHL-producing bacteria can improve plant growth and resistance against plant pathogens. In nature, plants may host a variety of AHL-producing bacteria and frequently experience numerous AHLs at the same time. Therefore, a coordinated response to combined AHL molecules is necessary. The purpose of this study was to explore the mechanism of AHL-priming using combined AHL molecules including N-(3-oxo-hexanoyl)-L-homoserine lactone, N-3-oxo-octanoyl-L-homoserine lactone, N-3-oxo-dodecanoyl-L-homoserine lactone, and N-3-oxo-tetradecanoyl-L-homoserine lactone and AHL-producing bacteria including Serratia plymuthica HRO-C48, Rhizobium etli CFN42, Burkholderia graminis DSM17151, and Ensifer meliloti (Sinorhizobium meliloti) Rm2011. We used transcriptome analysis, phytohormone measurements, as well as genetic and microbiological approaches to assess how the combination of structurally diverse AHL molecules influence Arabidopsis (Arabidopsis thaliana). Our findings revealed a particular response to a mixture of AHL molecules (AHL mix). Different expression patterns indicated that the reaction of plants exposed to AHL mix differs from that of plants exposed to single AHL molecules. In addition, different content of jasmonic acid (JA) and derivatives revealed that jasmonates play an important role in AHL mix-induced priming. The fast and stable decreased concentration of COOH-JA-Ile after challenge with the flagellin-derived peptide flg22 indicated that AHL mix modifies the metabolism of jasmonates. Study of various JA- and salicylic acid-related Arabidopsis mutants strengthened the notion that JA homeostasis is involved in AHL-priming. Understanding how the combination of AHLs primes plants for enhanced resistance has the potential to broaden our approaches in sustainable agriculture and will help to effectively protect plants against pathogens.
Introduction
During the course of their co-evolution with microorganisms, plants have evolved sophisticated defense systems in order to protect themselves against pathogens (Jones and Dangl, 2006; Jamil et al., 2022). Important parts of the defense are inducible mechanisms. The canonical cell surface pattern receptors (PRRs) recognize pathogen- or microbe-associated molecular patterns (PAMPs or MAMPs), inducing PRR-mediated immunity, also known as pattern-triggered immunity (PTI). In addition, intracellular receptors monitor the intracellular presence of pathogen effector proteins. As a response, those receptors activate effector-triggered immunity (ETI). Furthermore, distal tissues may enhance resistance in a process named systemic acquired resistance (SAR) or induced systemic resistance (ISR), when local sites react to pathogen attack or beneficial microbes, respectively. The establishment of either SAR or ISR depends on multiple long-distance systemic signals (Tonelli et al., 2020; Yu et al., 2022). Hormones and their derivatives play a central function in long-distance communication, salicylic acid (SA) and methyl salicylate (MeSA) are usually associated with the induction of SAR, while jasmonic acid (JA)- and ethylene (ET)-signaling pathways are essential requirements to establish ISR. In addition, several other molecules including azelaic and pipecolic acids are involved in long-distance signaling. Since the employed signaling pathways and downstream events, for example, the regulation of pathogenicity-related (PR) genes, are often overlapping between SAR and ISR, it is difficult to distinguish between those two responses on the transcriptional level.
A part of the ISR is priming for enhanced resistance. Originally described as activated by synthetic molecules, such as β-aminobutyric acid (BABA), the primed state can be triggered also by molecules produced by beneficial microorganisms. In this alerted state, a following exposure to pathogens or environmental changes stimulates stronger and faster responses. Since in primed plants, the defense responses are only induced when triggered by a secondary stimulus, priming has been proposed as an adaptive and low-fitness-cost defense mechanism.
N-acyl homoserine lactones (AHLs) are one of the best-studied quorum-sensing molecules. Bacteria synthesize various AHL molecules in order to sense cell density and coordinate their collective behavior. AHL molecules are composed of an acyl chain and a homoserine lactone ring. A 3-oxo substituent, or in rare situations a 3-hydroxy substituent, a terminal methyl branch, or variable degrees of unsaturation can diversify the acyl chain, which can range from C4 to C18 (Churchill and Chen, 2011). Bacteria recognize their own or heterogeneous AHL based on differences in the structure (Papenfort and Bassler, 2016). In addition, AHL molecules modulate interactions between bacteria and plants. This phenomenon drew special attention because of benefits it may confer to plants. One of these positive effects is modulation of the immune system. Increasing evidence demonstrates that particular AHL molecules trigger priming for enhanced resistance. AHL-priming uses signaling pathways, which partially overlaps with ISR. Recent reports showed that SA and oxylipin-dependent signaling pathways are involved in AHL-priming mediated by N-3-oxo-tetradecanoyl-L-homoserine lactone (oxo-C14-HSL) or N-3-oxo-octanoyl-L-homoserine lactone (oxo-C8-HSL) molecules (Schenk et al., 2014; Liu et al., 2020). Stronger and prolonged activation of AtMPK6 observed in AHL-primed plants was postulated to enhance the resistance to biotrophic and hemibiotrophic pathogens (Schikora et al., 2011). Specific changes in the metabolome and proteome of plants are involved in AHL-priming. Expression of many proteins involved in plant defense, energy, and metabolic activities was changed when Medicago truncatula roots were treated with AHL molecules (Mathesius et al., 2003). When Arabidopsis (Arabidopsis thaliana) seedlings were exposed to AHLs, changes in the expression of proteins involved in energy and carbohydrate metabolism, defense response, signal transduction, and cytoskeleton remodeling were also observed (Miao et al., 2012). Furthermore, AHL molecules affect plant root architecture via transcriptional factors, G-protein, and calcium signaling (Liu et al., 2012; Zhao et al., 2016). Similar to pure AHL molecules, AHLs of bacterial origin can enhance plant resistance. Several reports demonstrated that, in contrast to mutants with impaired AHL production, the wild-type, and AHL-producing, strains are able to induce resistance (Schuhegger et al., 2006; Pang et al., 2008; Zarkani et al., 2013). Root growth improvement was the predominant physiological response to AHL molecules, which were isolated from stains Aeromonas sp. SAL-17, and SAL-21 (Nawaz et al., 2020).
In nature, plants frequently experience numerous AHL at the same time because they may host a variety of AHL-producing bacteria. Therefore, a coordinated response to combined AHL molecules has been the focus of this study. In order to better understand the interactions in the rhizosphere, our group has previously investigated the complex interactions between the host plant (A. thaliana) and numerous AHL molecules (Shrestha et al., 2020). Exposure to an AHL mixture of three or four different molecules resulted in increased resistance to the hemibiotrophic pathogen Pseudomonas syringae pv. tomato (Pst). Such results demonstrate that induced resistance is a prominent effect of the perception of multiple AHLs. Interestingly, a mix of only two AHLs containing short-chained molecules was unable to induce an up-regulation of defense-related genes or enhanced resistance. These findings highlight the complexity of AHL-mediated interactions between bacteria and plants.
The purpose of this study was to explore the mechanism of AHL-priming using combined AHL molecules and AHL-producing bacteria. We used transcriptome analysis, hormone measurement as well as genetic and microbiological approaches to assess the question how diverse combined AHL molecules influence Arabidopsis. Our results revealed that plants responded to a mixture of AHLs in a specific way. As evidenced by time-course expression patterns starting with exposure to mix of four different AHL molecules until the response to challenge with the flagellin-derived 22-amino-acid peptide (flg22), response of AHL mix-primed plants differs from response of plants exposed to singe AHL molecules. Gene expression combined with changes in the content of different jasmonates revealed that jasmonates may play an important role in AHL mix-induced priming. Compared to the control and treatments with four single AHL molecules, AHL mix decreased the concentration of COOH-JA-Ile after the additional flg22 challenge. Analysis of diverse JA- and SA-related Arabidopsis mutants further strengthened the hypothesis that jasmonates are involved in AHL mix-priming. AHL mix-priming has the potential to broaden our approach in sustainable agriculture development, for example by using diverse AHL-producing bacteria in order to protect plants more effectively against pathogens.
Results
Dynamic inducible responses of plants exposed to AHL mix
The transcriptional changes occurring in plants upon exposure to AHL molecule(s) may help to understand the mechanism underpinning AHL-priming. Previously, transcriptional analysis of Arabidopsis was performed after exposure to different single AHL molecules (Zhao et al., 2016; Liu et al., 2022; Schenk et al., 2014). These studies assessed the gene expression in response to single AHL molecules as well as the response of AHL-primed plants to the flg22 (Felix et al., 1999), which is recognized by the Flagellin-Sensitive 2 (FLS2) receptor (Chinchilla et al., 2006).
In this study, we employed RNA-Seq approach to assess transcriptional changes in A. thaliana Col-0 (Col-0) upon AHL mix-priming. Plant samples were collected at the moment of treatment (t = 0 h) and throughout 96 h after application of AHL mix at 2, 24, 72, 74, and 96 h. The AHL mix consisted of N-(3-oxo-hexanoyl)-L-homoserine lactone (oxo-C6-HSL), oxo-C8-HSL, N-3-oxo-dodecanoyl-L-homoserine lactone (oxo-C12-HSL), and oxo-C14-HSL in 6 µM concentration each, an equivalent volume of acetone (solvent) was used as a control. In addition, plants were challenged with 100 nM flg22 72 h after exposure to combined AHLs (Supplemental Figure 1). For each sample, at least 30 two-week-old seedlings were pooled in quadruplicates. Read counts were normalized to the samples collected at 0 or 72 h (time point of flg22 challenge), and differentially expressed genes (DEGs) were identified in comparison to the solvent control. In total, 120, 57, 1765, 1803, and 1,546 DEGs were filtered at five different time points, separately. This experimental setup was chosen to extend previous studies that investigated the AHL-mediated transcriptional response with a restricted number of time points and a single type of AHL molecule.
To reveal the characteristics of plant response to AHL mix, functional analysis of DEGs was performed. Fifty-four, 50, and 1,692 genes responded to AHL mix at 2, 24, and 72 h, respectively (Figure 1A). Sixty-six genes responded to the AHL mix treatment both at 2 and 72, whereas only seven genes responded to AHL mix treatment 24 and 72 h after exposure. Furthermore, no common genes responding to the AHL mix treatment at all three time points were identified. The 66 genes differentially expressed at 2 and 24 h included Glutathione S-transferases 6 and 7 (GSTF6/7) involved in oxidative stress regulation and detoxification of harmful compounds (Cummins et al., 2011) and Peroxidase 4 (PER4) encoding class III peroxidase cell wall-targeted protein involved in lignification (Fernández-Pérez et al., 2015). The seven DEGs common for the 24 and 72 h time points included UDP-Glucosyl Transferase 73B1 (UGT73B1) and Extensin 18 (EXT18) encoding proline-rich extensin-like family protein. To reveal the possible functional changes, comparisons were performed using the enrichKEGG method (Figure 1C). The top three enriched KEGG pathways 2 h after exposure were related to phenylpropanoid biosynthesis, amino sugar and nucleotide sugar metabolism, as well as the mitogen-activated protein kinases (MAPK)-signaling pathway. Ribosome and its biogenesis were the top enriched pathways 24 h and plant–pathogen interaction was enriched 72 h after exposure to combined AHLs.
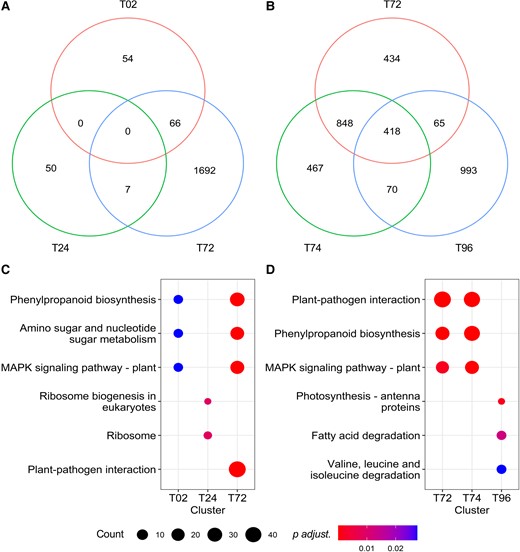
Plants respond dynamically to AHL mix-priming and a following flg22 challenge. Arabidopsis Col-0 wild-type response to AHL mix (A) was assessed at three time points: 2 h (T02), 24 h (T24), and 72 h (T72) after exposure to AHL mix (6 µM oxo-C6-HSL, oxo-C8-HSL, oxo-C12-HSL, and oxo-C14-HSL). The gene expression of T02, T24, and T72 was normalized to that prior to the exposure (0 h). AHL mix-primed plants’ response to flg22 (B) was assessed at two time points: 2 h (T74) and 24 h (T96) after additional challenge with 100 nM flg22. The gene expression of T74 and T96 was normalized to that of T72. DEGs were identified by threshold (P adjusted <0.05) and absolute value of fold change (>1.5) in comparisons between 0 h (A) or 72 h (B) and control treatment (acetone) samples. Biological pathway analysis was performed by enrichKEGG in clusterProfiler package. The comparisons of DEGs and the main enriched biological pathway from cluster T02, T24, and T72 (C) and from cluster T74 and T96 (D) revealed specific response to mixed AHLs and the following flg22 challenge.
Plants primed with AHL mix respond differently to the flg22 challenge over time
The enhanced plant resistance induced by different single AHL molecules including oxo-C8-HSL and oxo-C14-HSL has been demonstrated in several independent studies (Schikora et al., 2011; Shrestha et al., 2019; Liu et al., 2020). Shrestha et al. (2020) revealed that AHL mix-induced plant resistance against the plant pathogen Pst (Shrestha et al., 2020). However, the mechanism was not fully revealed. To address this point, we investigated the transcriptional reprogramming in PAMP-triggered plants using the flg22. Thus, AHL mix or solvent pre-treated plants were challenged with 100 nM flg22 72 h after the initial pretreatment. Plants were harvested after additional 2 h (74 h-time point) and 24 h (96 h-time point). Control samples collected at 72 h were used as a reference to reveal the AHL mix-primed plants’ response to the flg22 challenge (Figure 1B). Four hundred and sixty-seven and 993 genes were differentially expressed after the challenge with flg22 at 74 and 96 h, respectively. Seventy genes were common to both time points of 74 and 96 h, and 418 genes were identified at all three time points. Analysis of the enriched KEGG pathways revealed that among the DEGs, plant–pathogen interaction, phenylpropanoid biosynthesis, and MAPK-signaling pathway were enriched at 72 and 74 h (2 h after the flg22 challenge). In contrast, photosynthesis-antenna proteins, fatty acid degradation, and valine, leucine, and isoleucine degradation were enriched at 96 h (24 h after the challenge) (Figure 1D). These findings indicate that plants primed by AHL mix respond very dynamically to the flg22 challenge.
AHL mix changes gene expression over time and under various conditions
The responses of plants to AHL mix and to the flg22 challenge seem substantially different at different time points. Therefore, to fully mine the information, we performed a time-scale analysis of gene expression patterns across the experimental time-course. Genes with different expression patterns between control (CTL) and AHL mix treatment (MIX) were divided into six groups (Supplemental Figure 2). In groups 1 (Figure 2, A and B) and 4 (Figure 2, G and H), the expressions of genes mainly associated with plastid structure and photosynthetic dynamics declined and recovered swiftly from 72 to 96 h, compared to control. However, in groups 2 (Figure 2, C and D) and 3 (Figure 2, E and F), the expression of genes mainly associated with bacterial defense and Golgi vesicle transport spiked from 72 to 74 h in primed plants, whereas those in the control plants gradually increased. The rapid activation of defense-related genes in AHL mix treatment further emphasizes the ability for defense priming. From 0 to 96 h, genes involved in the cellular response to hypoxia and decreased oxygen levels (group 6) (Figure 2K and L) lightly varied in expression over time. Similarly, genes involved in the carboxylic acid catabolic and organic acid catabolic processes in group five (Figure 2, I and J) were steadily expressed in the presence of AHL mix. However, the up-regulated tendencies of the same genes in control conditions, from 74 to 96 h, suggest that naïve, non-primed, plants may require more energy to defend themselves against pathogens. Taken together, during the experimental period, gene expression patterns differ between the treatment with AHL mix and the control. Such changed expression, especially of photosynthesis- and defense-related genes in AHL mix-primed plants, may suggest a balanced response between the growth and resistance requirements.
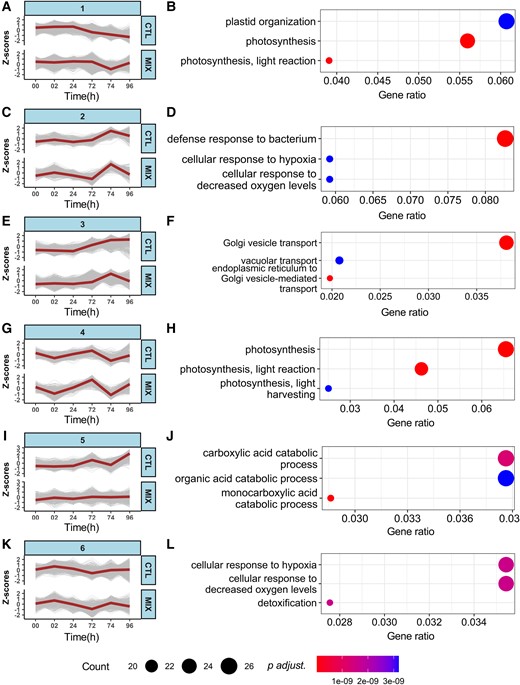
Growth and defense present a balanced response in primed plants. Gene expression patterns between control (CTL: acetone) and AHL mix (MIX: 6 µM oxo-C6-HSL, oxo-C8-HSL, oxo-C12-HSL, and oxo-C14-HSL) pre-treated samples were assessed across six time points (0, 2, 24, 72, 74, and 96 h) after exposure to AHL mix and the following flg22 challenge. Gene expression was normalized using the Z-score algorithm using expression level at each time point and the mean of gene expression in the group. A positive value of the Z-score indicates up-regulation, whereas the negative value of the Z-score indicates down-regulation. The DEGs patterns were identified by threshold (P adjusted <0.05) in comparisons between control (acetone) and AHL mix-treated samples across the experimental time-course. Six groups of gene expression patterns were identified, gene distance = 12.5. The brown lines in the plots (A, C, E, G, I, and K) show the median values of Z-score in each group. To reveal the function of each gene group, top 3 enriched biological processes are shown in left plots (B, D, F, H, J, and L) according to the gene ratio, which is calculated by (count of core enrichment genes)/(count of pathway genes). Expression trends observed from 0 to 72 h indicate that the responses to AHL mix were rather subtle. However, the pronounced trends from 72 to 74 h show fast responses to flg22 challenge in AHL mix-primed plants. The consolidated regulation of defense responses and photosynthesis suggest the existence of a balanced gene expression pattern.
Phytohormone-related genes participate in the AHL mix-priming process
To fully mine the information gained from the RNA-Seq approach, DEGs of each time point were clustered into GO term categories (Brionne et al., 2019) (Supplemental Figure 3). Interesting was the enrichment in gene ontology (GO) terms related to plant responses to chemicals or related to phytohormones such as indolebutyric acid (IBA), auxin, ET, SA, and JA. At 96 h, a high percentage of genes associated with JA- and SA-mediated signaling pathways drew our special attention.
Genes related to JA and SA were divided into biosynthesis, metabolism, perception, regulation, and signaling groups (Li et al., 2021a; Peng et al., 2021) and their expression patterns were compared to the control (Figure 3). JA biosynthesis-related genes such as Allene Oxide Cyclase 3 (AOC3), Lipoxygenases 2 and 4 (LOX2 and LOX4) were substantially down-regulated at 72 h however, up-regulated at 74 and 96 h. Meanwhile, other biosynthesis-related genes such as 12-Oxophytodienoate Reductase 1, 2 and 3 (OPR1, OPR2, and OPR3) (Beynon et al., 2009) showed a similar but lightly changed trend. In contrast, jasmonate metabolism-related genes such as Cytochrome P450 94B1 (CYP94B1) and Sulfotransferase 2A (ST2A) were substantially up-regulated at 72 h and down-regulated at 74 and 96 h. Similarly, other metabolism-related genes such as Jasmonate-Induced Oxygenase 2, 3 and 4 (JAO2, JAO3, and JAO4) showed a comparable trend. Transcription factors Octadecanoid-Responsive AP2/ERF-domain transcription factor 47 (ORA47) and basic helix-loop-helix (bHLH) DNA-binding protein MYC2 can regulate jasmonates production through binding to the promoters of biosynthesis genes (Hickman et al., 2017). Up-regulation of ORA47 was observed at 72 h and down-regulated at 74 and 96 h, while no expression changes of MYC2 were observed, indicating that ORA47 may play a key role in AHL mix-priming. In addition, Li et al. (2021b) proposed a model that homo-/heterodimers of Jasmonate Transporter 3/4 (AtJAT3/AtJAT4) facilitate jasmonates cell-to-cell transport, allowing for long-distance and self-propagating JA-signal transmission (Li et al., 2021). Gene JAT3, which encodes AtJAT3, showed up-regulation at both 74 and 96 h. This demonstrates that jasmonates accumulation may be involved in AHL mix-priming. Three genes, Lipoxygenase 1 (LOX1), Cytochrome P450 94B3 (CYP94B3), and ST2A, were noticeably up-regulated 2 h after exposure to AHL mix. Their functions are related to biosynthesis and metabolism of jasmonates. Taken together, genes related to biosynthesis and metabolism of jasmonates demonstrate changes in expression patterns upon AHL mix-priming.
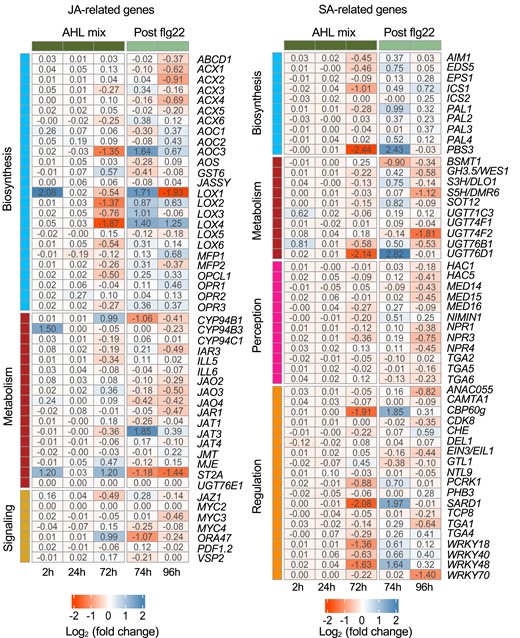
Jasmonic acid rather than salicylic acid-related genes participate in AHL mix-priming. GO terms analysis of DEGs revealed enrichment of genes related to phytohormones with a high percentage of genes associated to JA- and SA-signaling pathways. The related genes were selected according to Peng et al. (2021) and Li et al. (2021a). The fold change in expression values between AHL mix and solvent control are shown in the heatmap. Up and down regulated genes are placed in columns representing respective time point after exposure to AHL mix (2, 24, 72 h) or additional challenge with 100 nM flg22 (74 h, 96 h). Data were extracted from transcriptome analysis.
The expression of SA-signaling-related genes showed different trends (Figure 3). Several genes, including those related to SA biosynthesis: Isochorismate Synthase 1 (ICS1) and Auxin-Responsive GH3 Family Protein (PBS3/GH3.12), metabolism: Glucosyltransferases UGT76B1 and UGT76D1, and regulation: Calmodulin-Binding Protein CBP60g, SAR Deficient 1 (SARD1), W-box binding transcription factors WRKY18/40/70, and WRKY48 were down-regulated at 72 h and up-regulated at 74 h. PBS3, UGT76B1/D1, SARD1, and WRKY70 did not exhibit enhanced expression changes at 96 h. Furthermore, expression of SA receptors Nonexpresser of PR Genes 1, 2 and 3 (NPR1, NPR2, and NPR3) underwent only minor changes during the experimental period. No DEGs related to SA signaling were observed in response to AHL mix. These findings suggest that SA-signaling-related genes are only slightly regulated upon AHL mix-priming.
In summary, these findings demonstrate that the biosynthesis and metabolism of jasmonate-related genes may be involved in the AHL mix-priming process.
Jasmonate-related genes participate in the early reactions to the flg22 challenge
According to the above-described results, we hypothesized that jasmonates participate in AHL mix-priming. To confirm this hypothesis, an additional time-course transcription analysis of related genes was performed. Plants were cultivated and treated as described above; however, we were particularly interested in time points following the flg22 challenge. To keep the nomenclature consistent, the time points 0.5, 1, 2, and 4 h post flg22 challenge were defined as 72.5, 73, 74, and 76 h. The time point of 72 h represented plants primed with mixed AHLs, right before the challenge with flg22.
The jasmonate biosynthesis genes, including AOC1, LOX3, LOX4, and OPR3, switched from being down-regulated to up-regulated in a very short time (Figure 4). The expression of the biosynthesis gene Allene Oxide Synthase (AOS) also increased quickly. In contrast, the jasmonates metabolism genes, including Cytochromes P450 94B3 and 94C1 (CYP94B3/C1), and JAO2/3/4, switched from being up-regulated to down-regulated in the same period. Furthermore, the jasmonate response gene MYC2 was only slightly regulated over this period. Transcription factors encoding genes WRKY22/29 were also only slightly regulated. In addition, several genes showed unexpected changes. The biosynthesis gene LOX2 showed dynamic change over time, spiking at 72.5 h. Jasmonate Resistant 1 (JAR1), the gene encoding JA-amino synthetase (Staswick and Tiryaki, 2004), was slightly down-regulated throughout the experiment. The metabolism genes Jasmonic Acid Responcive 3 (IAR3), IAA-Amino Acid Hydrolase ILR1-like 6 (ILL6), and S-Adenosyl-l-Methionine:Jasmonic Acid Carboxyl Methyltransferase (JMT) were slightly up-regulated at 74 h, whereas the jasmonates transporter JAT1 was down-regulated after the flg22 challenge. In summary, these findings further confirm that jasmonate-related genes are specifically regulated upon AHL mix-priming.
![Genes related to jasmonates are up-regulated in the early stage of AHL mix-primed plants response to flg22. An additional time-course transcription analysis was focused on a short-time response to flg22 challenge in AHL mix-primed plants. Two-week-old plant Col-0 were transferred into ½ MS with AHL mix (MIX) or acetone (CTL) and grown for another 3 days. Subsequently plants were challenged with 100 nM flg22. Total RNA was extracted from samples for an RT-qPCR-based analysis prior to the challenge (72 h) and at 72.5, 73, 74, and 76 h. Expression of Ubiquitin ligase (At5g25760) was used for data normalization. Percentages of the target genes expression level at 72 h relative to the reference gene were shown at right of the heatmap table (CTL72 and MIX72). The values in the table represent log2 fold change (mean [MIX] vs mean [CTL]) for each time point. Each treatment and time point had four biological replicates.](https://oup.silverchair-cdn.com/oup/backfile/Content_public/Journal/plphys/191/3/10.1093_plphys_kiad017/1/m_kiad017f4.jpeg?Expires=1747884582&Signature=kL8pqkP-NkAO31yenIro4LX98n7g6JJuyxRxmFlo1Dz-AwTr-ED2bH9PEpcJ5Y10RKl9snbtU5uDu06XVS-hfb~iPEJRMLppWOtkSnugcxipz~DJ~T1C9bXAW-MtZQ0ppdEV-aHoJ0vOgiBWyiGmV0yo6Yc5hvnSODsxup-XsEHOhYJsmdnnmTLSb~aSR6fjqYRtF3hyKhMFvFsztktNz9eLBZ3jFuRwXpU9JIUa6xG1XBDdTKX58abhrDsDx8RGP63xA5W~sptFb8Of~3dkjwhzeRpSbcZn7Rdj4cMaDAL0mOvS3BZvVs5CTRo8e2Vxfg43PMktGDGPnq7iQrSKgA__&Key-Pair-Id=APKAIE5G5CRDK6RD3PGA)
Genes related to jasmonates are up-regulated in the early stage of AHL mix-primed plants response to flg22. An additional time-course transcription analysis was focused on a short-time response to flg22 challenge in AHL mix-primed plants. Two-week-old plant Col-0 were transferred into ½ MS with AHL mix (MIX) or acetone (CTL) and grown for another 3 days. Subsequently plants were challenged with 100 nM flg22. Total RNA was extracted from samples for an RT-qPCR-based analysis prior to the challenge (72 h) and at 72.5, 73, 74, and 76 h. Expression of Ubiquitin ligase (At5g25760) was used for data normalization. Percentages of the target genes expression level at 72 h relative to the reference gene were shown at right of the heatmap table (CTL72 and MIX72). The values in the table represent log2 fold change (mean [MIX] vs mean [CTL]) for each time point. Each treatment and time point had four biological replicates.
AHL mix-priming alters hormone content in primed plants exposed to flg22
To further corroborate our findings, content of particular plant hormones was measured 72 h after exposure the single AHL molecules, including oxo-C6-HSL, oxo-C8-HSL, oxo-C12-HSL, oxo-C14-HSL, as well as the AHL mix (all molecules at 6 µM concentration). In addition, we measured the hormone content 2 h (74 h) and 24 h (96 h) after challenge with 100 nM flg22.
In AHL-primed plants, the content of cis-OPDA, in case of oxo-C12-HSL treated plants, the content of JA and OH-JA-Ile, in oxo-C12-HSL- and oxo-C14-HSL-primed plants increased (Figure 5A). Surprisingly, the content of SA was significantly lower after priming with all four single AHL molecules as well as the AHL mix. Similarly, the content of abscisic acid (ABA) was lower after exposure to oxo-C6-HSL (Figure 5B). Except for SA, the AHL mix-priming had no impact on the concentration of measured hormones.
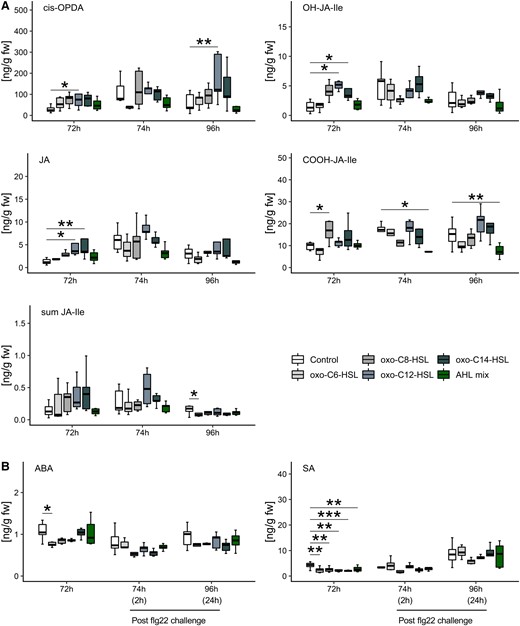
Content of jasmonates is lower in AHL mix-primed plants after exposure to flg22. Content of plant hormones was measured 72 h after exposure to either 6 µM of oxo-C6-HSL, oxo-C8-HSL, oxo-C12-HSL, and oxo-C14-HSL or its mix (AHL mix) as well as 2 h (74 h) and 24 h (96 h) after an additional challenge with 100 nM flg22. Changes in hormonal concentration were observed for cis-OPDA, JA and JA-derivative molecules; OH-JA-Ile and COOH-JA-Ile in plants primed with particular AHL molecules. Two and 24 h after flg22 challenge content of COOH-JA-Ile was lower in AHL mix-primed plants. A, Content of cis-OPDA was enhanced in long-chain AHL-primed plants 24 h after challenge with flg22. Concentrations of JA-Ile as well as of SA and ABA (B) did not change in response to the challenge, except for JA-Ile in oxo-C6-HSL pre-treated plants. Boxes represent the interquartile range between the first and the third quartile and the middle line marks the median, whiskers indicate 1.5× interquartile range. Each treatment contained minimum five biological replicates. Statistical analysis, comparisons are indicated by the horizontal lines above bars, was performed by Student's t-test, *P < 0.05, **P < 0.01, ***P < 0.001. Hormonal content is presented in ng per g plant fresh weight (ng/g fw).
Two hours after the challenge with flg22, only the content of COOH-JA-Ile in AHL mix-primed plants decreased. The same situation was observed 24 h (96 h time point) after flg22 challenge in those plants. Interestingly, and according to previous studies (Schenk et al., 2014), the content of cis-OPDA increased in long-chain AHL-primed plants (Figure 5A). In addition, the content of JA-Ile was lower after oxo-C6-HSL pretreatment, compared to the control. These findings indicate that the content of jasmonate-catabolic derivatives diminished in AHL mix-primed plant after the following exposure to flg22, COOH-JA-Ile may play an important role in AHL mix-priming.
AHL mix-priming is missing in mutants deficient in jasmonate homeostasis
In the next step, we sought to substantiate our observations in a genetic approach. In a first step, we developed a sterile hydroponic system, based on glass jars, perlite, and ¼ MS medium and verified the priming ability of Arabidopsis in such growth conditions using BABA. Our results revealed that jar-grown plants can be successfully primed for enhanced resistance by BABA against the plant pathogen Pst (Supplemental Figure 4).
In Arabidopsis, LOX2 is a key enzyme in the oxidation of unsaturated fatty acids, leading to the JA precursor, oxylipins (Grebner et al., 2013). The JA is afterwards linked by the JA-amino synthetase (JAR1) to isoleucine, in order to obtain the active JA-Ile (Staswick and Tiryaki, 2004). The bZIP transcription factors TGA2, TGA5, and TGA6 and the jasmonate receptor Coronatine-Insensitive Protein 1 (COI1) are involved in the effects of reactive oxylipins on plant growth and stress responses (Yan et al., 2009; Stotz et al., 2013). Thus, we compared the AHL mix-induced resistance against Pst in wild-type plants Col-0 and JA-signaling deficient mutants including lox2 (impaired in lipoxygenase 2), jar1-1 (impaired in JA-amino synthetase JAR1), coi1-16 (impaired in jasmonate receptor COI1) and tga2/5/6 (triple mutant of bZIP transcription factors TGA2, TGA5, and TGA6). Plants roots were pretreated with AHL mix or solvent control, and subsequently leaves were inoculated with Pst. Bacterial proliferation was used to assess plants’ resistance. The lower bacterial proliferation in AHL mix-primed Col-0 plants compared to the control demonstrates that AHL mix can prime plants in such hydroponic system (Figure 6). This is also mutually confirmed by the result of Shrestha et al. (2020). Unlike the wild-type Col-0, mutants deficient in jasmonate homeostasis such as lox2, jar1-1, tga2/5/6, and coi1-16 showed no difference in bacterial proliferation when we compared AHL mix-primed plants to control. These findings suggest that the jasmonates and the related signaling pathway are required in the AHL mix-priming process (Figure 6).
![AHL mix-priming is missing in mutants deficient in jasmonate homeostasis. Two-week-old wild-type Arabidopsis Col-0 plants and jasmonate signaling deficient mutants were transferred into a sterile hydroponic system, based on glass jars, perlite, and ¼ MS medium. Roots were pre-treated with AHL mix or solvent (control) for 72 h and subsequently leaves were inoculated with the plant pathogen Pst. Bacterial proliferation was used to assess plant resistance and expressed as difference in the number of CFU in leaf discs between 96 and 2 h time points (Δlog [CFU/leaf disc]). Lower bacteria proliferation in AHL mix-primed plants compared to the control was observed in Col-0 however, not in mutants deficient in jasmonate homeostasis: lox2, jar1-1, tga2/5/6, and coi1-16. The bsmt1 and myb72 mutants were able to induce AHL mix-priming comparable to the Col-0 wild-type plants. Boxes represent the interquartile range between the first and the third quartile and the middle line marks the median, whiskers indicate 1.5× interquartile range. Statistical analysis was performed using Student's t-test, **P < 0.01, ***P < 0.001, ****P < 0.0001, n ≥ 3.](https://oup.silverchair-cdn.com/oup/backfile/Content_public/Journal/plphys/191/3/10.1093_plphys_kiad017/1/m_kiad017f6.jpeg?Expires=1747884582&Signature=Ezrs~ogGfKuc-MU0X80e3pDhyauAt~mzeUer2W9SYEATF7HMLJ1uegGxWj09jAt2c6WWTptVCWouD3jAyjYbXhEY85vWvuZsLKFjyOyAXkF3mc4VYotXZiHw3QxIo34-prTTAfHuZT4riEqHn3xof1jFssjLrCYnDrn~Bu0zKjzATKqJbn6NTKFfCqkWPsVxWiqNdPwaAIJBvoiEfKErzgtdEoUXndCo9dfWeY4orLQMpmpKR~M7frfSkNiNzP~BSzOM7n9UHG-hJhFeO~DfOnp51IciqPDYSUZQ42uOhIsa~De3SdTM1dfdKgglzPX~vpExGKc8yfJS-jII-R~2TQ__&Key-Pair-Id=APKAIE5G5CRDK6RD3PGA)
AHL mix-priming is missing in mutants deficient in jasmonate homeostasis. Two-week-old wild-type Arabidopsis Col-0 plants and jasmonate signaling deficient mutants were transferred into a sterile hydroponic system, based on glass jars, perlite, and ¼ MS medium. Roots were pre-treated with AHL mix or solvent (control) for 72 h and subsequently leaves were inoculated with the plant pathogen Pst. Bacterial proliferation was used to assess plant resistance and expressed as difference in the number of CFU in leaf discs between 96 and 2 h time points (Δlog [CFU/leaf disc]). Lower bacteria proliferation in AHL mix-primed plants compared to the control was observed in Col-0 however, not in mutants deficient in jasmonate homeostasis: lox2, jar1-1, tga2/5/6, and coi1-16. The bsmt1 and myb72 mutants were able to induce AHL mix-priming comparable to the Col-0 wild-type plants. Boxes represent the interquartile range between the first and the third quartile and the middle line marks the median, whiskers indicate 1.5× interquartile range. Statistical analysis was performed using Student's t-test, **P < 0.01, ***P < 0.001, ****P < 0.0001, n ≥ 3.
In addition to jasmonate-related mutants, we monitored the AHL mix-induced priming in two supplementary signaling mutants. MeSA might be used as a long-range SAR signal (Park et al., 2007). BSMT1 is a methyltransferase from Arabidopsis that converts SA to MeSA in vitro (Chen et al., 2003). In addition, the transcriptional regulator MYB72 has been described an essential component of ISR signaling mediated by rhizobacteria (Van der Ent et al., 2008). Both mutants (bsmt1 and myb72) revealed improved resistance upon AHL mix-priming. These data suggest that AHL mix-priming may have certain unique characteristics.
Taken together, these findings imply that the jasmonates are essential for AHL-priming, and in contrast to ISR or SAR, AHL mix-priming may be dependent on specific unique features.
AHL-producing bacteria cannot induce priming in mutants deficient in jasmonate homeostasis
When employing the AHL mix-priming approach to agricultural systems, bacteria-originated AHLs may be an interesting strategy. Therefore, we asked if joined AHL-producing bacteria may impact plant resistance in a manner similar to AHL mix-priming. To answer this question, four AHL-producing bacteria including Serratia plymuthica HRO-C48, Rhizobium etli CFN42, Burkholderia graminis DSM17151, and Ensifer meliloti (Sinorhizobium meliloti) Rm2011, were chosen. Independent studies revealed that AHL produced by those bacteria mainly include oxo-C6-HSL, oxo-C8-HSL, oxo-C12-HSL, and oxo-C14-HSL, respectively (Liu et al., 2007; Suarez-Moreno et al., 2008; Zarkani et al., 2013; Zheng et al., 2015). AHL-negative strains, expressing the lactonase AttM (Carlier et al., 2003) from the plasmid pBBR2-attM, were used as bacterial control. Six-week-old plants of Arabidopsis were root drenched three times with AHL-producing bacterial suspension (wild-type strains), AHL-negative bacteria (attM strains), or the solvent 10 mM MgCl2 (control), and subsequently, leaves were infiltrated with Pst. Bacterial proliferation was assessed by enumeration of colony forming units (CFUs) 2 and 96 h after infiltration. Bacterial growth rate was calculated accordingly. Inoculation with AHL-producing bacteria enhanced plant resistance against Pst, as indicated by the slower pathogen growth rate (Figure 7), whereas AHL-negative bacteria could not induce this effect in Col-0 plants (Figure 7). This suggests that bacterial AHL molecules may enhance resistance in Arabidopsis against Pst.
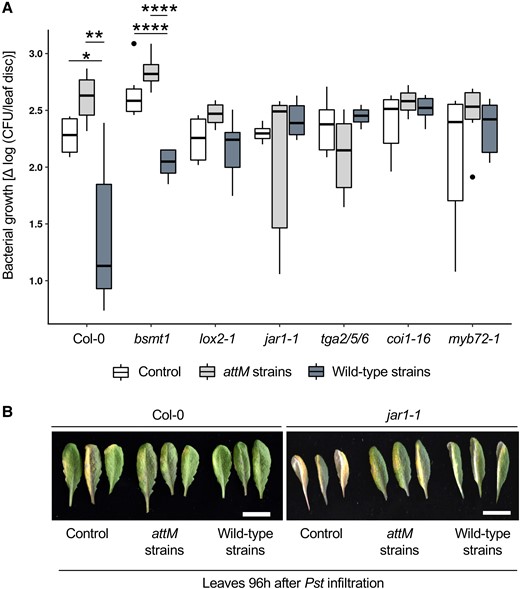
Bacteria-originated AHL molecules prime Col-0 but not mutants deficient in JA-signaling. Roots of 6-week-old Arabidopsis plants were drenched three times with four AHL-producing bacteria: S. plymuthica HRO-C48, R. etli CFN42, B. graminis DSM17151, and S. meliloti Rm2011 (wild-type strains). AHL-negative strains, expressing the lactonase AttM from the plasmid pBBR2-attM, were used as bacterial control (attM strains) in addition to 10 mM MgCl2 used as solvent control. Leaves were subsequently infiltrated with Pst, OD600 = 0.01 (107 CFU/ml) in 10 mM MgCl2. Bacterial proliferation was assessed by enumeration of CFUs, 2 and 96 h after infiltration. Bacterial growth rate was calculated accordingly. Inoculation with AHL-producing bacteria enhanced resistance against Pst in Col-0 plants, as revealed by the lower pathogen growth rate, whereas AHL-negative bacteria could not induce this effect (A). However, lox2, jar1-1, tga2/5/6, and coi1-16 mutants revealed no difference in the bacterial growth rate. Boxes represent the interquartile range between the first and the third quartile and the middle line marks the median, whiskers indicate 1.5× interquartile range, points indicate outliers. Statistical analysis was performed by Student's t-test, *P < 0.05, **P < 0.01, ****P < 0.0001, n ≥ 3. Representative infected leaves of wild-type Col-0 and jar1-1 were collected 96 h after the Pst infiltration from all three treatments (B). The bar indicates 1 cm.
In analogy to the in vitro assays, mutants deficient in jasmonate homeostasis were cultivated in soil and treated in a similar manner. In all treatments, lox2, jar1-1, tga2/5/6, and coi1-16 mutants revealed no difference in the bacterial (Pst) growth rate (Figure 7). This suggests that the jasmonates are involved in priming with diverse bacterial AHL molecules. In contrast, inoculation with AHL-producing bacteria, improved resistance of the bsmt1 mutant to Pst in the same way as the pure chemicals. This shows that in both hydroponic and soil settings, MeSA molecules may not be required for AHL mix-priming. Surprisingly, no difference in Pst proliferation was observed in case of the soil-grown myb72 mutant, opposite to the result in hydroponic system. In conclusion, the jasmonate homeostasis seems necessary for bacterial AHL mix-priming.
Discussion
Plants and microbes communicate in a variety of ways, many of which rely on chemical signals (Jamil et al., 2022) of both, plant and microbial origins. Some of microbial molecules can trigger a systemic response in plants. AHLs are well-studied signaling compounds that act as mediators in plant–microbe communication (Schikora et al., 2016; Hartmann et al., 2021). Understanding plant responses to distinct AHL molecules is required to unravel the process of AHL-induced stimulation. Furthermore, insight into the mechanisms of AHL-priming might aid in optimizing the use of AHL-producing bacteria in sustainable agriculture.
Plant responses to AHL molecules are determined by AHL structure, concentration, time of exposure, and plant species. In Arabidopsis, independent studies have demonstrated that the beneficial activity of AHLs depends on the length of their lipid chain. Short-chain AHLs such as C6-HSL, oxo-C6-HSL, and oxo-C8-HSL mainly promote root growth (von Rad et al., 2008; Liu et al., 2012; Miao et al., 2012; Schenk et al., 2012; Zhao et al., 2016). Furthermore, Liu et al. (2012) revealed that certain biological activities such as root elongation were influenced by AHL concentration. In tomato (Solanum lycopersicum), wheat (Triticum aestivum), and oilseed rape (Brassica napus var. napus) plants, enhanced resistance against pathogens or increased salt tolerance was observed in plants inoculated with C6- and C8-AHL-producing bacteria or treated with the pure molecules (Schuhegger et al., 2006; Muller et al., 2009; Zhao et al., 2020). In Arabidopsis and barley (Hordeum vulgare), several long-chain AHL such as oxo-C12-HSL and oxo-C14-HSL have been shown to induce resistance against pathogens (Schikora et al., 2011; Shrestha et al., 2019; Wehner et al., 2021). Mixed AHLs used in this study included two short-chain AHL (oxo-C6-HSL and oxo-C8-HSL), which were shown to promote plant growth in many studies (Schenk et al., 2014; Zhao et al., 2016, 2020; Liu et al., 2020), and a known AHL-priming inducer oxo-C14-HSL (Schikora et al. 2011), in addition to oxo-C12-HSL.
The gene expression in response to different single AHL molecules, including C6-HSL, oxo-C6-HSL, oxo-C8-HSL, oxo-C10-HSL, and oxo-C14-HSL assessed in previous studies, revealed substantial differences (Schenk et al., 2014; Zhao et al., 2016; Liu et al., 2022). To explore the characteristics of plant responses to AHL mix, results from previous studies were re-annotated and compared to results obtained in this study (Figure 8). The relationship between the different single AHL treatments and AHL mix shows a high percentage of unique DEGs in AHL mix-treated plants (Figure 8). In detail, 495, 466, and 50 genes responded to oxo-C6-HSL, oxo-C8-HSL, and AHL mix 24 h after exposure, respectively (Supplemental Figure 5A). Only 3 genes responded to the treatment with oxo-C6-HSL, oxo-C8-HSL, and the AHL mix. One gene responded to oxo-C8-HSL and AHL mix. Furthermore, a gene list of 1,390 genes regulated 72 h after exposure was identified in this comparison (Supplemental Figure 5B). Of all the genes, 61% (852 genes) responded to specifically to AHL mix, 9% (126 genes) responded to C6-HSL, 12% (169 genes) responded to oxo-C10-HSL, and 8% (118 genes) responded to oxo-C14-HSL treatments, and only 5 genes responded to both C6-HSL treatment and AHL mix treatment. Those genes include PER5 encoding peroxidase superfamily protein, PP2B13 encoding phloem protein 2-B13 and CRK37 encoding a cysteine-rich receptor-like protein kinase. Fourteen genes responded to both oxo-C10-HSL and AHL mix treatments, including PME56 encoding pectin lyase-like superfamily protein, ATHMP07 encoding heavy metal transport/detoxification superfamily protein, and HUP32 encoding a hypothetical protein. Six genes responded to both oxo-C14-HSL and AHL mix treatments, and those genes include FOX2 encoding FAD-binding berberine family protein, PCR9 encoding PLAC8 family protein and BGLU45 encoding beta-glucosidase 45. No genes seem to respond to all four different treatments. These findings showed that the plant responses to mixed AHLs are quite different from the responses to single AHL molecules. Further comparison of genes responding to the flg22 challenge in single AHL- or AHL mix-primed plants, revealed that the common genes between oxo-C14-HSL and AHL mix-primed plants have different expression trends (Supplemental Figure 6). This indicates that the coordinated reaction in AHL mix-primed plants is rather specific.
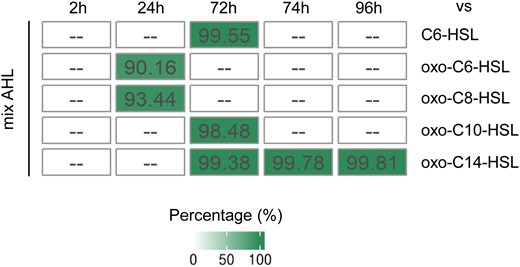
The proportion of unique DEGs in AHL mix-treated plants if compared to different single AHL-treated plants. To clarify the relationship between single AHL treatment and treatment with AHL mix, the unique genes, regulated in response to given AHL molecule or their mix were identified. The proportion of unique DEGs was present as percentage of all DEGs. Data were collected from previous studies (Zhao et al., 2016; Liu et al., 2022; Schenk et al., 2014) on Arabidopsis treated with N-hexanoyl-L-homoserine lactone (C6-HSL), oxo-C6-HSL, oxo-C8-HSL, oxo-C10-HSL, and oxo-C14-HSL. Results were arranged by time point assessed in the particular study. — indicates missing data.
Time of exposure is another important factor for AHL action in plants (Mathesius et al., 2003). No alterations of a transient effect or systemic responses were observed in plants treated with individual C6-HSL, oxo-C10-HSL or oxo-C14-HSL for an extended period (Schenk et al., 2014). In this study, we highlighted the plant responses to AHL mix in the early stage of exposure, up to 72 h. The dynamic change of DEGs indicates that plants adapt to AHL mix. Twenty-four hours after exposure, DEGs mainly involved in the ribosome biogenesis however, not JA-related functions. Further analysis of the gene expression in response to the two single AHL molecules (oxo-C6-HSL and oxo-C8-HSL) revealed that genes related to JA biosynthesis (Supplemental Table 1). Surprisingly, the increased number of DEGs at 72 h revealed the changed physiological states, involving mainly photosynthesis and defense responses (Figure 1). Comparing the impact of single AHL action in Schenk et al., (2014) to mixed AHLs, revealed that AHL mix triggers expression of genes related mainly to plant–pathogen interaction pathway, phenylpropanoid biosynthesis and MAPK-signaling pathway (Figure 1). This indicates that AHL mix influences plant resistance rather than growth. However, the composition of AHL mix may be an essential factor in the reaction of plants, for example the mix of short-chain AHL molecules improved root parameters of wheat under high salinity (Nawaz et al., 2020).
Phytohormones play a fundamental role in plants’ reactions. JA and ET are involved in induced systemic resistance, whereas SA accumulation is observed during the systemic acquired resistance (Jamil et al., 2022). In this study, we assessed the function of jasmonates in AHL mix-priming. The changes in expression of jasmonate biosynthesis and metabolism genes and lower concentration of jasmonate-catabolism molecules were observed in plants inoculated with AHL mix (Heitz et al., 2012; Hu et al., 2013; Huang et al., 2021; Li et al., 2017a; Li et al., 2021a; Li et al., 2021b; Li et al., 2017b; Smirnova et al., 2017). The activated and prolonged expression of jasmonate biosynthesis genes, together with repression of jasmonate-catabolic genes in AHL mix-primed plants after challenge with flg22, further supported this notion that AHL mix-priming may suppress the catabolism of jasmonates and therefore alter the jasmonate homeostasis. Such alternation could positively impact plants resistance (Figure 9). The missing AHL mix-priming for induced resistance in several jasmonate-related mutants confirmed the basic role of the jasmonates during AHL-priming.
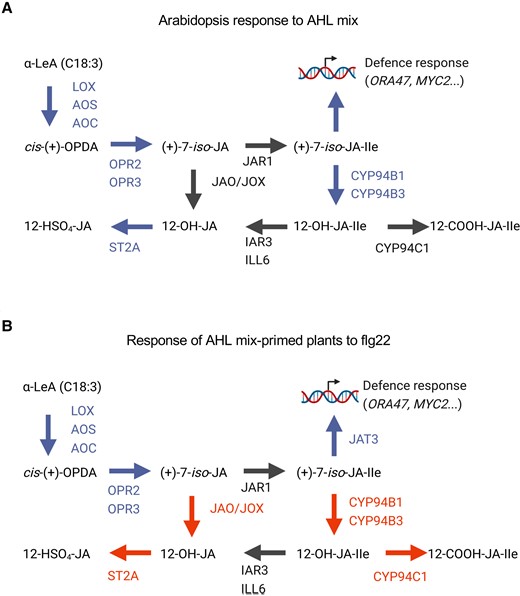
Model of jasmonates participation in AHL mix-priming. Transcriptome analysis, plant hormones measurement, and genetic analysis suggest that jasmonate biosynthesis and metabolism participate in AHL mix-priming. In response to AHL mix molecules, biosynthesis of active compounds in the JA-signaling pathway seems to be induced (A). In response to the flg22 challenge in AHL mix-primed plants, genes responsible for catabolism of jasmonates were repressed, and the content of jasmonates catabolic derivatives was reduced. This indicates that primed plant would actively inhibit the degradation of active compounds sustaining therefore the JA-signaling (B). Blue color indicates enhanced expression, red color indicates decreased expression or inhibiting activity, black color indicates no changes in gene expression. Arrows indicate the following steps in jasmonate metabolism. Involved jasmonate molecules: α-linolenic acid (α-LeA or C18:3); cis-(+)-12-oxo-phytodienoic acid (cis-OPDA); (+)-7-iso-jasmonate (JA); (+)-7-iso-jasmonyl-L-isoleucine (JA-Ile); (+)-7-iso-12-hydroxyjasmonoyl-L-isoleucine (12-OH-JA-Ile); 12-carboxy-jasmonoyl-isoleucine (12-COOH-JA-Ile); 12-hydroxy-jasmonic acid sulfate (12-HSO4-JA). Involved enzymes: Lipoxygenase (LOX); Allene oxide synthase (AOS); Allene oxide cyclase (AOC): OPDA reductase (OPR2, OPR3); Jasmonoyl-isoleucine synthetase (JAR1); Jasmonate-induced oxygenase (JOX); Jasmonic acid oxidase (JAO); cytochrome P450 (CYP) enzymes (CYP94B1, CYP94B3, CYP94C1); amidohydrolases (IAR3, ILL6); sulfotransferase (ST2A); jasmonate transporter (JAT3).
Plant responses involve changes in concentration of many phytohormones. In infected plants, SA initiates the early defense-related gene expression. Activated expression of genes in SA-signaling pathway was observed in AHL mix-primed plants. However, compared to the control, no accumulation of SA nor induced expression of genes encoding for SA receptors NPR1, NPR3, and NPR4 were observed. These phenomena suggest that SA signaling is not involved in the AHL mix-priming. In this study, we assessed also auxin-, ET- and ABA-related genes (Supplemental Figure 3). However, their expression was not different from the control. Remarkable was the increased expression of IBA-related genes, IBA may be an auxin storage form (Strader et al., 2011).
AHL molecules are important players in plant–bacteria interaction. Inoculum composed of different AHL-producing bacteria can be a practical solution for improving plant growth and repress plant diseases in agricultural context (Hartmann et al., 2021). In this study, we extended the AHL mix-priming from the pure chemicals to inoculation with 4 different AHL-producing bacteria. Both, the AHL mix-induced resistance and the resistance induced after inoculation with diverse AHL-producing bacteria were verified in pathogen proliferation assays. Both phenomena depend on jasmonate homeostasis. Up to now, besides the bacterial AHL receptors, AHL-interacting proteins were postulated only in animal systems: the IQ-motif Containing Ras GTPase-Activating-like Protein (IQGAP), the Peroxisome Proliferator-Activated Receptors (PPAR), or the Taste Receptor 2 Member 38 (T2R38) (Turkina and Vikstrom, 2019). Very recently, a plant AHL-Priming Protein 1 (ALI1) was postulated to mediate the response to oxo-C14-HSL in Arabidopsis (Shrestha et al., 2022). How plants perceive other AHL molecules is not known yet. Comprehensive research is still required to reveal the details of interaction between diverse AHL molecules and plants. Nonetheless, our study highlighted the function of jasmonates in AHL mix-priming. Understanding how mixed AHLs prime plants should help to develop appropriate mixed inocula for sustainable agriculture.
Materials and methods
Plant growth and AHL mix treatments
For transcriptome analysis, surface-sterilized seeds of Arabidopsis (A. thaliana) genotype Col-0 plants (Col-0) as well as T-DNA insertion lines: bsmt1, coi-1-16, jar1-1, lox2-1, myb72-1, tga2/5/6, were germinated on half-strength MS (Murashige and Skoog) (½ MS) and grown under 8 h light/16 h dark photoperiod at 21°C in a growth chamber for 2 weeks. Thereafter, seedlings were transferred into six-well plates with ½ MS liquid medium and grown for additional 24 h prior to the treatment. The blank control samples (BLA00) were collected before the addition of AHL mix or solvent control (acetone). The AHL mix was prepared by mixing 4 different AHL: oxo-C6-HSL, oxo-C8-HSL, oxo-C12-HSL, and oxo-C14-HSL (Sigma–Aldrich Chemie GmbH, Munich, Germany) (Supplemental Figure 1). Subsequently, AHL combination (AHL mix) was added to the growth medium at 6 µM concentration each. The same volume of solvent (acetone) was added to control (CTL) samples. Samples were collected 2, 24, 72, 74 and 96 h thereafter and named CTL02, MIX02, CTL24, MIX24, CTL72, MIX72, CTL74, MIX74, CTL96, MIX96, depending on treatment and time. In addition, 72 h after AHL mix-priming, plants were challenged with 100 nM flg22. Each well in the six-well plates contained 40–60 seedlings, and was defined as one biological replicate. Each treatment had 4 different biological replicates (Supplemental Figure 1).
For reverse transcription quantitative PCR (RT-qPCR) gene expression assays, AHL mix and the solvent control samples were prepared similarly in ½ MS medium. Plants were grown in a growing chamber for 72 h after being transferred into six-well plates. The samples were collected right before or 0.5, 1, 2, 4 h post the 100 nM flg22 challenge. To keep the nomenclature consistent, the time point before flg22 challenge was named 72 h, and the following time points after the flg22 challenge were defined as 72.5, 73, 74, and 76 h, respectively. Each experimental variant had four independent biological repetitions.
For plant hormone measurement, plants were grown and treated as described above. Samples were collected right before and 2 h as well as 24 h post 100 nM flg22 challenge. The time points 2 and 24 h post flg22 challenge were named 74 and 96 h, respectively. Each treatment had five independent biological repetitions.
In pathogenicity test assays, 3-week-old seedlings were transferred to sterile glass jars in the in vitro system or pots in the greenhouse system. Jar-grown Arabidopsis were supported with sterile Perlite and quarter-strength MS medium (¼ MS). Soil-grown Arabidopsis were supported with around 100 g standard bedding substrate. Plants were grown in a growth chamber for another 3–4 weeks. During the growth period, fresh ¼ MS was added weekly to the jars, according to the plant requirement. AHL mix and its solvent control (acetone) were prepared in ¼ MS. Thereafter, the liquid medium in the jars was completely replaced with the medium containing AHL mix or the solvent (control). Jar-grown Arabidopsis were grown for another 72 h and subsequently spray-inoculated with Pst at a concentration of OD600 = 0.01 (107 CFU/mL) in 10 mM MgCl2. Soil-grown Arabidopsis were inoculated with either AHL-producing or AHL-negative bacterial combinations three times. Thereafter leaves were infiltrated with Pst using the same bacterial concentration as above mentioned. Samples were collected at 2h and 96 h post Pst challenge, homogenized and diluted with 10 mM MgCl2, dropped in duplicates on King's B plates, and incubated for additional 30 h for CFU enumeration. Each sample contained 4 leaf discs collected from different plants, and each experimental variant had at least 3 biological replicates.
Bacterial strains and growth
Serratia plymuthica HRO-C48, R. etli CFN42, B. graminis DSM17151, and E. meliloti (S. meliloti) Rm2011 were used as source of bacteria-originated AHL mix. AHL-negative bacterial strains were constructed by introducing the plasmid pBBR1MCS-2 carrying the lactonase gene attM from Agrobacterium tumefaciens (Carlier et al., 2003) into S. plymuthica and P. graminis (A. tumefaciens (pBBR2-attM) and P. graminis (pBBR2-attM)). R. etli (pBBR2-attM) and E. meliloti (pBBR2-attM) were constructed previously (Zarkani et al., 2013). Growth media were supplemented with either streptomycin (250 µg/ml), kanamycin (100 µg/ml), or ampicillin (50 µg/ml), as required. Overnight grown bacterial cultures were harvested by centrifugation and washed in 10 mM MgCl2. Bacterial suspensions were mixed and inoculated to plants at a final concentration of 106 CFU per gram soil, 10 mM MgCl2 was used as control.
Gene expression assays, RT-qPCR
Total RNA extraction and purification were performed using TriFast (peqGOLD, USA) and DNase I (Quanta Biosciences, USA) kits according to manufacturer recommendations. One microgram RNA was used for cDNA synthesis using qScript cDNA Synthesis Kit (Quanta Biosciences, Beverly, MA, USA). The RT-qPCR reaction was set as following: 10 µL qPCR Master Mix (NEB, USA), 0.5 µL forward primer (10 µM), 0.5 µL reverse primer (10 µM), 5 µL cDNA and 4 µL nuclease-free water using in the following program: initial denaturation (95°C, 60 s, 1×), denaturation (95°C, 15 s), extension (60°C, 30 s) with 40 cycles and additional melting curve (60°C–95°C, 1×). The primers list is presented in Supplemental Table 2.
Plant hormone measurement
Phytohormone extraction and subsequent LC–MS analysis was performed as described previously (Vadassery et al., 2012; Davila-Lara et al., 2021). Briefly, each sample (100 mg fresh weight) was extracted with 1.5 mL methanol containing 60 ng D6–abscisic acid (Toronto Research Chemicals, North York, ON, Canada), 60 ng of D6–jasmonic acid (HPC Standards GmbH, Borsdorf, Germany), 60 ng D4–salicylic acid (Santa Cruz Biotechnology, Dallas, TX, USA) and 12 ng of D6–jasmonoyl-isoleucine conjugate (HPC Standards GmbH, Borsdorf, Germany) as internal standard. Phytohormone analyses were carried out by LC–MS/MS on an Agilent 1260 series HPLC system (Agilent Technologies) with a tandem mass spectrometer QTRAP 6500 (SCIEX). Chromatographic separation and mass spectrometry were performed according to Davila-Lara et al. (2021).
Whole transcriptome approach
Whole RNA extraction and purification were performed using Rneasy Plant Mini Kit (Qiagen, Germany). Library preparation and DNBSEQ PE100 sequencing were performed by the Beijing Genomics Institute (BGI, China). Read mapping and feature counting were performed using Rsubread with the default setting (Liao et al., 2019). The Arabidopsis genome Col-CEN_v1.2 (Naish et al., 2021) was used as a reference in this study. DEGs were identified using Deseq2 (Love et al., 2014) (Supplemental Figure 1). Untreated control (BLA00) was used as a reference to normalize gene expression in the samples collected 2, 24, and 72 h after AHL mix treatment. Meanwhile, samples of 72 h were used as references to normalize the gene expression in the samples collected at 74 and 96 h (2 and 24 h after additional flg22 challenge). The DEGs were filtered by threshold (P adjust <0.05, fold change >1.5). For the functional analysis, KEGG pathway enrichment and biological process (BP) were performed with the R package clusterProfiler (Wu et al., 2021). For time-course gene expression analysis, the DEGs were filtered by P adjust value (0.05) in the comparison between full model (time + treat + treat: time) and reduced model (time + treat). The DEGs were filtered by threshold (P adjust <0.05) in the comparisons between treatment AHL mix and control. Figures were created using R (version 4.2.1) and RStudio (“Ghost Orchid” Release).
Statistical analysis
All statistical analysis was performed using R (version 4.2.1) and RStudio (“Ghost Orchid” Release), and details are indicated in figure legends, methods, and Supplementary Figures. Reverse transcription quantitative PCR assays were performed in four biologically independent experiments and Pst assays were performed in three biologically independent experiments. P values <0.05 in the Student's t-test were considered as indicative for a significant difference.
Accession numbers
The raw sequences were uploaded to Sequence Read Archive (SRA) with the BioProject number: PRJNA865543. Accession numbers of genes relevant for this study are listed in Supplemental Table 1 and Supplemental Table 2.
Supplemental data
The following materials are available in the online version of this article.
Supplemental Figure S1. Experimental setting and time schedule of the transcriptome analysis.
Supplemental Figure S2. Cluster dendrogram of gene expression patterns across the experimental time-course.
Supplemental Figure S3. Changes in the GO term “biological process response to chemical” during the experimental time-course.
Supplemental Figure S4. BABA induces enhanced resistance in plants grown in a jar hydroponic system.
Supplemental Figure S5. Gene expression in response to AHL mix differs from that of response to single AHL molecules.
Supplemental Figure S6. Responses to the flg22 challenge differs between plants primed with AHL mix and oxo-C14-HSL.
Supplemental Table S1. Jasmonic acid (JA)-related gene expression in a single AHL treatment.
Supplemental Table S2. List of oligonucleotides used in this study.
Funding
The work of Yongming Duan was supported by China Scholarship Council (CSC), grant number: 201806350041. The work of Min Han was supported by China Scholarship Council (CSC), grant number: 201906350038. The project was supported by the PrimedPlant-2 project, founded by Federal Ministry of Education and Research (BMBF), managed by Projektträger Jülich (PtJ) granted to Adam Schikora, grant number 031B0886B.
Acknowledgments
The mutant seeds were kindly shared by Susanne Berger (tga2/5/6), Corné Pieterse (myb72-1), and Daniel Klessig (bsmt1).
References
Author notes
Y.D. and A.S. conceived the research project. Y.D. performed the whole transcriptome analysis and analyzed the data of this research project. Y.D., M.H., and M.G. performed plant sample preparation, RNA extraction, and RT-qPCR. A.M. and M.R. performed phytohormone measurement. Y.D., M.H., M.G., and J.P. performed the Pst pathogen assay. Y.D. wrote the manuscript and A.M., M.H., and A.S. contributed to writing, reviewing, and editing the manuscript. All authors agreed with the publication of this manuscript.
The author responsible for distribution of materials integral to the findings presented in this article in accordance with the policy described in the Instructions for Authors (https://dbpia.nl.go.kr/plphys/pages/General-Instructions) is Adam Schikora ([email protected]).
Conflict of interest statement. The research was conducted without a potential conflict of interest in any commercial or financial relationships.