-
PDF
- Split View
-
Views
-
Cite
Cite
Jingxuan Guo, Lizhi Long, Anle Chen, Xiaonan Dong, Zhipeng Liu, Limei Chen, Junying Wang, Lixing Yuan, Tonoplast-localized transporter ZmNRAMP2 confers root-to-shoot translocation of manganese in maize, Plant Physiology, Volume 190, Issue 4, December 2022, Pages 2601–2616, https://doi.org/10.1093/plphys/kiac434
- Share Icon Share
Abstract
Almost all living organisms require manganese (Mn) as an essential trace element for survival. To maintain an irreplaceable role in the oxygen-evolving complex of photosynthesis, plants require efficient Mn uptake in roots and delivery to above-ground tissues. However, the underlying mechanisms of root-to-shoot Mn translocation remain unclear. Here, we identified an Natural Resistance Associated Macrophage Protein (NRAMP) family member in maize (Zea mays), ZmNRAMP2, which localized to the tonoplast in maize protoplasts and mediated transport of Mn in yeast (Saccharomyces cerevisiae). Under Mn deficiency, two maize mutants defective in ZmNRAMP2 exhibited remarkable reduction of root-to-shoot Mn translocation along with lower shoot Mn contents, resulting in substantial decreases in Fv/Fm and plant growth inhibition compared to their corresponding wild-type (WT) plants. ZmNRAMP2 transcripts were highly expressed in xylem parenchyma cells of the root stele. Compared to the WT, the zmnramp2-1 mutant displayed lower Mn concentration in xylem sap accompanied with retention of Mn in root stele. Furthermore, the overexpression of ZmNRAMP2 in transgenic maize showed enhanced root-to-shoot translocation of Mn and improved tolerance to Mn deficiency. Taken together, our study reveals a crucial role of ZmNRAMP2 in root-to-shoot translocation of Mn via accelerating vacuolar Mn release in xylem parenchyma cells for adaption of maize plants to low Mn stress and provides a promising transgenic approach to develop low Mn-tolerant crop cultivars.
Introduction
Manganese (Mn) is an essential micronutrient for plants, acting as a co-factor and activator of many enzymes. Mn plays prominent roles in the oxygen-evolving complex (OEC) of photosystem II (PSII) and superoxide dismutase (Alscher et al., 2002; Shen, 2015; Schmidt et al., 2016; Schmidt and Husted, 2019). Plants are prone to Mn deficiency in calcareous and sandy soils, which are prevalent in northern Europe, USA, and Canada and also in southern Australia (Pallotta et al., 2000; Hebbern et al., 2005; Husted et al., 2005; Stoltz and Wallenhammar, 2014). Lack of Mn in plants leads to reduced photosynthetic activities and damage of reactive oxygen species, ultimately resulting in growth limitations and yield losses (Broadley et al., 2012). Like all other essential transition metals, Mn is toxic to plants in excess (Alejandro et al., 2020). Therefore, plants have to evolve suitable mechanisms of Mn uptake and transport to maintain Mn homeostasis for ensuring optimal growth and development.
Mn acquisition from soil and long-distance translocation in plants are mediated by the members of various transporter families, including natural resistance-associated macrophage protein (NRAMP), ZRT/IRT-like protein (ZIP), yellow stripe like (YSL), and metal tolerance protein (MTP; Socha and Guerinot, 2014; Alejandro et al., 2020). In Arabidopsis (Arabidopsis thaliana), AtNRAMP1 serves as a primary Mn transporter localized at the plasma membrane of root epidermis and cortex cells, and mediates the high-affinity uptake of Mn from soil (Cailliatte et al., 2010). In rice (Oryza sativa), the polar-localized Mn transporters, OsNRAMP5 in the distal and OsMTP9 in the proximal side of the root exodermis and endodermis, drive the radial transport of Mn from soil into the stele (Sasaki et al., 2012; Ueno et al., 2015). In addition to Mn uptake at the root surface, HvIRT1 (Iron Regulated Transporter 1) in barley (Hordeum vulgare) is involved in Mn transport across the root endodermis into the stele (Long et al., 2018). The radial movement and storage of Mn into the root stele and subsequent xylem loading are assumed to be crucial for delivering Mn to shoots. However, the underlying mechanisms of root-to-shoot Mn translocation are poorly understood so far.
Plant vacuoles contribute essentially to root-to-shoot transport of metals, through the modulation of vacuolar sequestration capacity, which is mainly controlled by the tonoplast-localized transporters (Peng and Gong, 2014). For example, surplus cadmium (Cd) is sequestered into the vacuole of root cells by the vacuolar membrane-localized OsHMA3 (Heavy Metal ATPases 3), consequently decreasing root-to-shoot translocation of Cd in rice (Ueno et al., 2009; Miyadate et al., 2011; Yan et al., 2016). The tonoplast-localized copper (Cu) exporter Copper Transporters 5 (COPT5) is also involved in the reallocation of Cu from the roots to reproductive organs (Garcia-Molina et al., 2011; Klaumann et al., 2011). For vacuolar Mn transport, AtNRAMP3 and AtNRAMP4 mediate Mn release from the vacuole, and on the contrary, AtMTP8, OsMTP8.1, and OsMTP8.2 confer Mn loading into the vacuole (Thomine et al., 2003; Lanquar et al., 2005, 2010; Chen et al.,2013; Eroglu et al., 2016, 2017; Takemoto et al., 2017). Under Mn-replete conditions, AtMTP8 is supposed to negatively affect the root-to-shoot Mn translocation as the atmtp8 mutant shows increased Mn concentration in shoots but decreased in roots (Eroglu et al., 2016). However, the vacuolar Mn transport process for the root-to-shoot Mn translocation, and its physiological contribution to adaption of plants to low Mn stress, remain to be elucidated.
As a C4 crop species, maize (Zea mays) requires substantial amounts of Mn in both light-dependent and carbon-assimilating reactions of photosystem, and hence shows more sensitivity to Mn deficiency with reduction of PSII stability and functionality (Long et al., 2020). The maize genotypes tolerant to Mn deficiency usually display efficient Mn uptake and root-to-shoot translocation (Long et al., 2021). In this study, using subcellular and tissue-specific localization analysis, we showed that a maize NRAMP family member, ZmNRAMP2, was localized to the vacuolar membrane and mainly expressed in xylem parenchyma cells in the root stele. The function of ZmNRAMP2 in Mn transport was further verified by growth complementation of yeast (Saccharomyces cerevisiae) mutants. Employing the knockout/down mutants and transgenic overexpressing lines of maize, we demonstrated the role of ZmNRAMP2 in root-to-shoot translocation of Mn, which was crucial for maintaining photosynthetic activity and plant growth under Mn-limited condition. Thus, our study highlights that ZmNRAMP2-mediated vacuolar Mn release in root stellar cells determines the ability of Mn translocation to shoots, and offers a promising target for genetic improvement of low Mn-tolerant crops.
Results
ZmNRAMP2 encodes a tonoplast-localized protein
Members of the NRAMP family are assigned into two distinct clades based on the phylogenetic analyses (Supplemental Figure S1). Within the upper clade, most members are localized at the plasma membrane, such as AtNRAMP1, OsNRAMP1, OsNRAMP5, and OsNRAMP3 (Cailliatte et al., 2010; Takahashi et al., 2011; Sasaki et al., 2012; Yamaji et al., 2013; Chang et al., 2020), except AtNRAMP6 that only preferentially targets to the plasma membrane under Mn-limited condition (Li et al., 2022). In the lower clade, most members mainly are localized in the endomembrane system, including the tonoplast-localized AtNRAMP3, AtNRAMP4, and OsNRAMP2 (Thomine et al., 2003; Lanquar et al., 2005, 2010; Li et al., 2021) and the trans-Golgi network (TGN)-localized AtNRAMP2 (Alejandro et al., 2017; Gao et al., 2018). Based on the amino acid sequence similarities, eight putative NRAMP proteins are identified in maize (Supplemental Figure S1). Among them the protein encoded by GRMZM2G178190 shares 89% amino acids sequence similarity with the rice vacuolar membrane-localized transporter OsNRAMP2 (Li et al., 2021), and hence is named as ZmNRAMP2.
To investigate the subcellular localization of ZmNRAMP2, the ZmNRAMP2–eGFP fusion protein was transiently expressed under control of the CaMV 35S promoter into protoplasts of maize mesophyll cells. The ZmNRAMP2–eGFP-dependent green fluorescence signal and the chlorophyll auto-fluorescence red signal were imaged by confocal laser microscopy. It showed that ZmNRAMP2–eGFP signal delineated a sharp closed circle, corresponding to the tonoplast. Whereas the chloroplasts with red fluorescence and nucleus appeared outside of the green circle within the protoplasts (Figure 1, A–D). This result indicates that ZmNRAMP2-encoded protein targets to the tonoplast in maize cells.
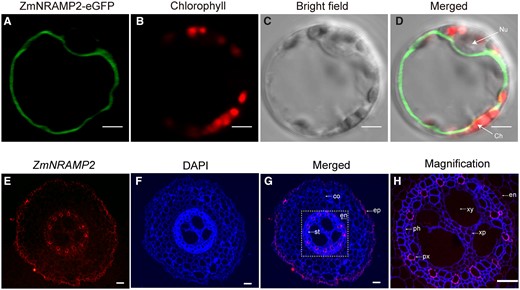
Subcellular and tissue localization of ZmNRAMP2 in maize. A–D, Tonoplast localization of ZmNRAMP2 in maize mesophyll protoplasts. Maize mesophyll protoplasts transiently expressing ZmNRAMP2–eGFP fusion protein were imaged by confocal laser microscopy. A, GFP fluorescence of ZmNRAMP2–eGFP, (B) chlorophyll autofluorescence, (C) bright-field, (D) merged images. Nu, nuclear; Ch, chloroplast. Scale bars = 5 μm. E–H, In situ RNA hybridization in maize roots with the ZmNRAMP2 antisense probe. E, Red fluorescence revealed by antisense probe of ZmNRAMP2, (F) root structure dyed by DAPI, (G) merged images, (H) enlargement of the yellow dotted box in (G). Ep, epidermis; co, cortex; en, endodermis; pe, pericycle; st, stele; xy, xylem; xp, xylem parenchyma cells; ph, phloem; Scale bars = 40 μm.
ZmNRAMP2 is mainly expressed in xylem parenchyma cells in root stele
We first employed reverse transcription–quantitative polymerase chain reaction (RT–qPCR) to investigate the expression patterns of ZmNRAMP2 in shoots and roots of 7-day-old maize seedlings exposed to varied Mn regimes. The result showed that the expression levels of ZmNRAMP2 neither in root nor in shoot were altered by the prolonged Mn deficiency or excess up to 7 days (Supplemental Figure S2, A and B). This indicates that the expression of ZmNRAMP2 is not subjected to transcriptional regulation in response to plant Mn nutritional status.
We further employed in situ RNA hybridization to determine the tissue-specific localization of ZmNRAMP2 transcripts in maize root. The root cross sections approximately 1 cm from the root apical meristem of 10-day-old maize seedlings were sampled for hybridization. The sense probe for ZmNRAMP2 served as a negative control and did not display any hybridization signal (Supplemental Figure S3). In contrast, the red fluorescence signal of ZmNRAMP2 arising from the antisense probe was revealed in the epidermis and root stele. Specifically, in root stele the strongest signal was observed in the xylem parenchyma cells (Figure 1, E–H). These results therefore indicated ZmNRAMP2 is mainly expressed in xylem parenchyma cells in root stele where translocation of nutrients to shoots takes place.
ZmNRAMP2 protein mediates transport of Mn in yeast
To determine the Mn transport activity of ZmNRAMP2, the yeast mutant defective in Mn influx across the plasma membrane (Δsmf1) or endomembrane (Δsmf2) was employed for the functional growth complementation assay. Under Mn-limited conditions induced by the addition of the Mn-chelator ethylene glycol-bis(2-aminoethyl) tetraacetic acid (EGTA), both yeast mutants Δsmf1 and Δsmf2 expressing the empty vector pDR196 showed poor growth (Figure 2). The heterologous expression of ZmNRAMP2 or ZmNRAMP2–eGFP fusion protein was able to restore the growth arrest of Δsmf1, like AtNRAMP1 that mediates Mn influx across the plasma membrane (Figure 2A;Thomine et al., 2000). Similarly, ZmNRAMP2 or ZmNRAMP2–eGFP expression was able to rescue the growth arrest of Δsmf2, like AtNRAMP2 that mediates Mn influx across the endomembrane (Figure 2B; Alejandro et al., 2017). Along with the functional expression of ZmNRAMP2–eGFP in yeast, we also determined that the ZmNRAMP2–eGFP-dependent green fluorescence signal was also exclusively localized to the tonoplast in both yeast mutants Δsmf1 and Δsmf2 (Supplemental Figure S4). This was consistent with its vacuolar membrane localization in maize mesophyll protoplasts. Thus, these results suggest that ZmNRAMP2 functions as a Mn transporter that mediates vacuolar Mn release to cytosol, ensuring growth of yeast mutants under Mn-limited conditions.
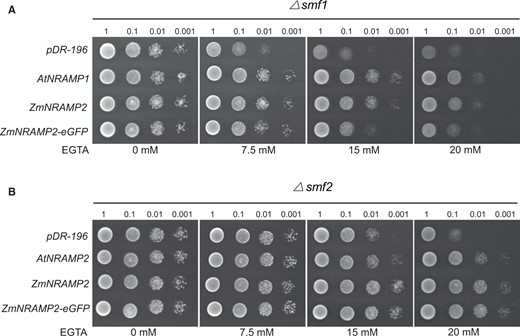
Mn transport of ZmNRAMP2 in yeast. A, Functional complementation of ZmNRAMP2 in yeast mutant Δsmf1 defective in Mn transport across the plasma membrane. Yeast cells were transformed with empty vector pDR-196, AtNRAMP1 (positive control), ZmNRAMP2, and ZmNRAMP2-eGFP. B, Functional complementation of ZmNRAMP2 in yeast mutant Δsmf2 defective in Mn transport across the endomembrane. Yeast cells were transformed with empty vector pDR-196, AtNRAMP2 (positive control), ZmNRAMP2, and ZmNRAMP2-eGFP. Four-fold serial dilutions were dropped on the SD-U agar plate supplemented with gradient concentrations of Mn-chelator EGTA (0, 7.5, 15, or 20 mM), and incubated at 28°C for 3 days.
Disruption of ZmNRAMP2 reduces photosynthetic activity and plant growth under Mn deficiency
We further assessed the physiological function of ZmNRAMP2 in maize by employing the gene-knockout mutant (zmnramp2-1) and -knockdown mutant (zmnramp2-2). The zmnramp2-1 mutant and the corresponding wild-type (WT; B73) were segregated from an EMS (ethyl methane sulfonate)-induced mutant line ems61eec (Lu et al., 2018). In zmnramp2-1, a G-to-A substitution at 305 bp was introduced into genomic sequence of ZmNRAMP2 as revealed by Sanger sequencing (Supplemental Figure S5B). This led to a nonsense mutation (TGG to TAG) in the first exon of ZmNRAMP2 and resulted in a null mutation via a premature termination mutation 102C/* (Supplemental Figure S5A). The zmnramp2-2 mutant and the corresponding WT (W22) were segregated from a Mu-transposon insertion line UFMu-07918 (McCarty et al., 2005). The zmnramp2-2 mutant and WT(W22) were identified by the Mu-TIR-specific primer (TIR6) and the ZmNRAMP2 gene-specific primers (6F and 6R; Supplemental Figure S5D). By sequencing, the position of the Mu insertion was determined at the genomic region (+494 to +502 bp) in first exon of ZmNRAMP2 gene (Supplemental Figure S5C). As a result, ZmNRAMP2 transcript levels in the zmnramp2-2 mutant were significantly reduced to 10%–15% of that in W22, as revealed by RT–qPCR analysis (Supplemental Figure S5E).
These two mutants and their corresponding WT plants were then grown in nutrient solution supplied with 1-µM Mn (+Mn; Mn sufficiency) or without Mn (−Mn; Mn deficiency) for up to 14 days. The responses of plants to Mn deficiency were evaluated based on the time-course changes of chlorophyll fluorescence parameter Fv/Fm, representing the maximal photochemical efficiency of PSII, in the youngest fully emerged leaf (Figure 3, A–C; Schmidt et al., 2013; Long et al., 2021). Under the +Mn condition, the dynamic Fv/Fm values maintained close to 0.8 in all tested genotypes. Under the –Mn condition, the Fv/Fm values of B73 were slightly reduced to 0.7 at 12 days after treatment (DAT). In contrast, the Fv/Fm values of the zmnramp2-1 mutant were dramatically reduced to 0.55 at 10 DAT, being substantially lower than that of B73 across the whole period (Figure 3A). This genotypic difference of Fv/Fm in leaves of Mn-deficient plants was further illustrated by using an imaging pulse-amplitude-modulation (PAM; Figure 3C). Compared to B73, W22 plants showed a higher sensitivity under Mn deficiency as the Fv/Fm values dropped to 0.6 at 10 DAT (Figure 3B). Even so, a substantially reduction of Fv/Fm values was observed in the zmnramp2-2 mutant compared to that in W22 (Figure 3B). The two zmnramp2 mutants and their respective WT plants revealed a similar growth at 14 DAT under Mn-sufficient condition (Figure 3, D–F). In contrast, owing to the severe reduction of photosynthetic efficiency under Mn deficiency, the two zmnramp2 mutants exhibited significant growth suppression, in terms of reduced shoot and root dry weight (Figure 3, D–F). Thus, these results indicate that disruption of ZmNRAMP2 leads to a severe decline of the PSII photosynthetic efficiency and subsequent inhibition of plant growth under the Mn limited condition.
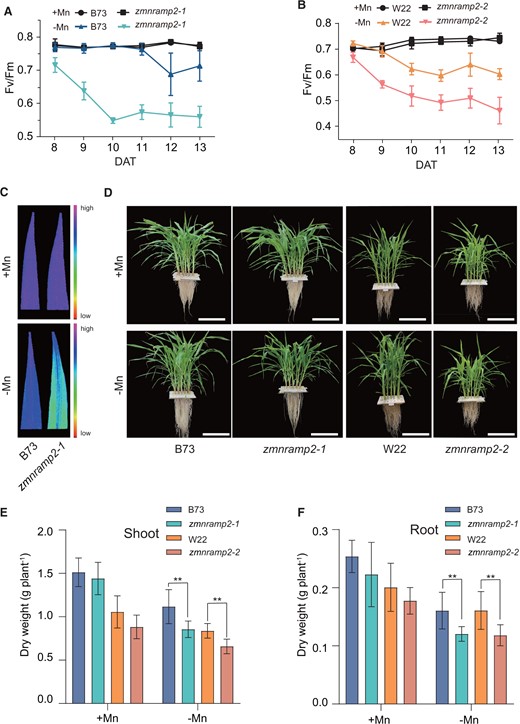
Disruption of ZmNRAMP2 reduces photosynthetic activity and plant growth under Mn deficiency. A and B, Dynamic changes of Fv/Fm in the youngest fully emerged leaf of zmnramp2-1 and WT (B73) (A), and zmnramp2-2 and WT (W22) (B) from 8 to 13 DAT. Maize seedlings were grown in nutrient solution supplied with 1-µM Mn (+Mn) or without Mn (−Mn). Data are presented as means ± sd (n = 6, biological replicates). C, Images of Fv/Fm in the youngest fully emerged leaf of zmnramp2-1 and WT (B73) at 13 DAT determined by PAM. D–F, Plant growth and dry weight of zmnramp2-1, zmnramp2-2, and the corresponding WT (B73, W22) plants grown under contrasted Mn regimes. D, Images of plant growth (scale bar = 25 cm), (E) shoot dry weight, and (F) root dry weight. Maize seedlings were grown in nutrient solution supplied with 1-µM Mn (+Mn) or without Mn (−Mn) for 14 days. Data are presented as means ± sd (n = 6, biological replicates). Significant difference was indicated at **P < 0.01 by Student’s t test.
Disruption of ZmNRAMP2 impairs root-to-shoot translocation of Mn
To investigate the role of ZmNRAMP2 in Mn transport in maize, the root and shoot Mn concentrations were measured in different maize genotypes hydroponically grown under contrasted Mn regimes. Under the +Mn condition, the root and shoot Mn concentrations did not differ between the two zmnramp2 mutants and their respective WT plants, except for a slight increase in the root of zmnramp2-2 (Figure 4, A and B). However, under the −Mn condition, the root Mn concentrations of the two zmnramp2 mutants were almost twice as much as those of the WT, although the shoot Mn concentrations were similar among the different genotypes (Figure 4, A and B).
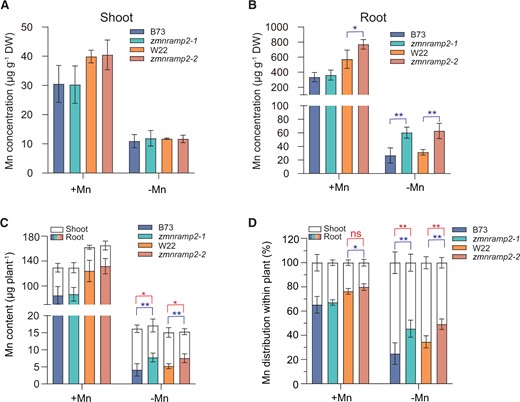
Disruption of ZmNRAMP2 impairs the root-to-shoot Mn translocation in maize. A and B, Mn concentrations of shoot and root respectively, C, Mn contents of shoot and root, and D, Mn distribution between root and shoot of zmnramp2-1, zmnramp2-2, and the corresponding WT (B73, W22) plants grown under contrasted Mn regimes. Maize seedlings were grown in nutrient solution supplied with 1-µM Mn (+Mn) or without Mn (−Mn) for 14 days. Data are presented as means ± sd (n = 6, biological replicates). Significant difference was indicated at *P < 0.05 and **P < 0.01 by Student’s t test (labeling in red and blue for shoots and roots, respectively), and ns, not significant.
We then calculated the Mn contents in root and shoot, to determine the whole plant Mn accumulation (Figure 4C) as well as Mn distribution between root and shoot within plants (Figure 4D). The results showed that the overall plant Mn accumulation did not differ between the two zmnramp2 mutants and their corresponding WT irrespective of Mn conditions (Figure 4C), indicating that ZmNRAMP2 is not involved in Mn uptake in maize.
Under Mn deficiency, the two zmnramp2 mutants showed higher Mn contents in roots but lower in shoots, compared to those of the WT (Figure 4C). Moreover, the calculations of Mn distribution within plants showed that about 75% of the total plant Mn content was allocated to shoots in WT plants (B73), while this allocation was significantly reduced to 55% in the zmnramp2-1 mutant (Figure 4D). Compared to B73, another WT plant (W22) showed lower Mn allocation to shoots (about 65%), which was consistent with its higher sensitivity to low Mn (Figure 3, A and B and Figure 4D). Again, in the zmnramp2-2 mutant this proportion was down to 50%, being significantly lower than that in W22 (Figure 4D). The significant decreases in Mn distribution to shoots in the two zmnramp2 mutants indicate a reduced capacity of root-to-shoot Mn translocation. In contrast, the root and shoot zinc (Zn) contents and the root–shoot distribution did not significantly differ between the two zmnramp2 mutants and the WT (Supplemental Figure S6). These results demonstrate that, rather than Mn uptake by plants, ZmNRAMP2 is crucial for Mn translocation from root to shoot within plants.
Disruption of ZmNRAMP2 leads to Mn retention in root stellar cells
The Mn concentration of xylem sap was further determined to verify the reduction of root-to-shoot Mn translocation in the zmnramp2 mutant. The zmnramp2-1 mutant displayed a significantly lower Mn concentration in xylem sap than the corresponding WT (B73) irrespective of Mn supply levels (Figure 5A). Notably, the decline percentage of zmnramp2 relative to WT was about 17% under Mn sufficiency, while under Mn deficiency it extended to 36%. Because ZmNRAMP2 does not affect the root-to-shoot Zn translocation (Supplemental Figure S6), Zn concentration in xylem sap was detected as a control and showed no difference between the two genotypes (Figure 5B). Thus, disruption of ZmNRAMP2 in maize results in reduction of Mn into xylem vessels and subsequently decreased Mn translocation to shoots, particularly under low Mn condition.
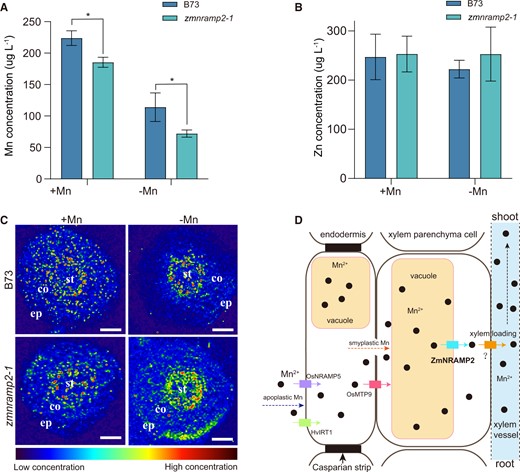
Reduction of Mn in xylem sap and retention of Mn in roots of the zmnramp2-1 mutant. A and B, Concentration of Mn (A) and Zn (B) in xylem sap of zmnramp2-1 and WT (B73) plants. Maize seedlings were grown in nutrient solution supplied with 1-µM Mn (+Mn) or without Mn (−Mn) for 14 days. Data are presented as means ± sd (n = 3, biological replicates). Significant difference was indicated at *P < 0.05 by Student’s t test. C, 55Mn distribution in the cross sections of roots of zmnramp2-1 and WT (B73) plants from (A). Segments about 1-cm distance from the root tip were sampled for LA–ICP–TOF–MS analysis with spot size of 10 μm. The signal intensities were normalized to endogenous carbon (measured as 13C). The red-to-blue color scale represents high to low range of Mn concentration, respectively. Co, cortex; ep, epide rmis; st, stele. Scale bar = 100 μm. D, Schematic model illustrating the function of ZmNRAMP2 in the root-to-shoot Mn translocation. The plasma membrane-localized OsNRAMP5 (purple box), OsMTP9 (carmine box) and HvIRT1 (green box) transporters are responsible for the apoplastic Mn entering root stele across the endodermal cells. In xylem parenchyma cells, the tonoplast-localized ZmNRAMP2 (cyan box) mediates vacuolar Mn release into cytoplasm, providing an adequate amount of intracellular Mn for xylem loading via unknown transporters (tawny box). Disruption of ZmNRAMP2 then leads to retention of Mn in root stellar cells, and reduces Mn in xylem sap for subsequent translocation to shoots.
Laser ablation–inductively coupled plasma–time of fly–mass spectrometry (LA–ICP–TOF–MS) was then employed to examine the Mn retention in roots of zmnramp2-1 due to the defective Mn translocation to shoots. Under the Mn-sufficient condition, the bioimaging of Mn distribution in the cross-section of roots showed no difference between zmnramp2-1 and WT (B73; Figure 5C). Under the Mn-deficient condition, the Mn-dependent signals appeared aggregated in root stellar cells. Importantly, these signals were higher in the zmnramp2-1 mutant than in the WT, indicating a retention of Mn in root stele (Figure 5C). We then propose a working model on ZmNRAMP2-dependent root-to-shoot Mn translocation in maize (Figure 5D). ZmNRAMP2 is mainly expressed in xylem parenchyma cells and mediates vacuolar Mn release into the cytoplasm. This allows to provide an adequate amount of intracellular Mn for xylem loading via unknown transporters. However, loss of function of ZmNRAMP2 leads to disruption of vacuolar Mn release, which results in limited intracellular Mn being loaded into xylem for subsequent root-to-shoot translocation.
Overexpression of ZmNRAMP2 enhances tolerance of transgenic maize plants to Mn deficiency
To test whether elevated expression of ZmNRAMP2 could improve maize plant tolerance to Mn deficiency, we generated two independent transgenic lines (OE-1 and OE-4) by overexpressing ZmNRAMP2 under the control of the maize Ubiquitin promoter. In these two transgenic lines, the transcript levels of ZmNRAMP2 were significantly increased up to seven- to nine folds of that in the WT (ND101; Figure 6A). Lines OE-1, OE-4, and the WT (ND101) were then grown hydroponically and subjected to Mn sufficiency (+Mn, 1-μM Mn) or -deficiency (−Mn, without Mn) for 17 days. Again, under the +Mn condition the Fv/Fm values in all tested genotypes remained close to 0.8 (Figure 6B). Mn deficiency substantially reduced the Fv/Fm values of the WT (ND101), which initially dropped to 0.6 at 15 DAT and continuously down to 0.5 at 17 DAT. Remarkably, under the –Mn condition the two ZmNRAMP2-overexpressing lines revealed slightly reduced Fv/Fm values down to 0.7 until 17 DAT, indicating their higher tolerance to Mn deficiency than that of the WT (ND101). No difference in dry weight of shoot or root was observed between transgenic lines and the WT (Supplemental Figure S7, A and B). Under the −Mn condition, however, the two overexpressing lines exhibited much higher shoot, but lower root, Mn concentrations and contents compared to the WT (ND101; Figure 6, C–E). In the Mn-deficient WT (ND101) about 70% of the total plant Mn content was allocated to shoots, while this allocation to shoots was enhanced up to 81%–86% in the two overexpressing lines (Figure 6F). Thus, these results indicate that elevated expression of ZmNRAMP2 could improve the capacity of root-to-shoot translocation of Mn in transgenic plants, resulting in enhanced tolerance of plants to Mn deficiency.
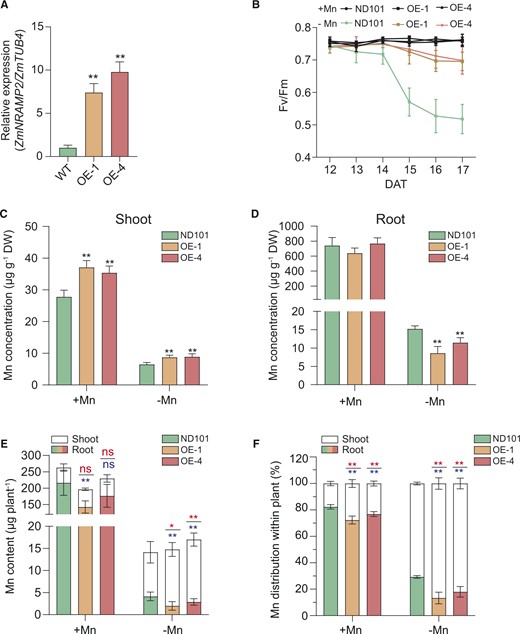
Overexpression of ZmNRAMP2 enhances the tolerance of transgenic maize plants to Mn deficiency. A, Relative expression of ZmNRAMP2 in the two independent overexpression lines (OE-1 and OE-4) and WT (ND101). Data are presented as means ± sd (n = 3, biological replicates). Significant difference was indicated at **P < 0.01 by Student’s t test. B, Dynamic changes of Fv/Fm in the youngest fully emerged leaf of the ZmNRAMP2-overexpressing lines (OE-1 and OE-4) and WT (ND101) from 12 to 17 DAT. Maize seedlings were grown in nutrient solution supplied with 1-µM Mn (+Mn) or without Mn (−Mn). Data are presented as means ± sd (n = 6, biological replicates). C and D, Mn concentrations of shoot and root respectively, E, Mn contents of shoot and root, and F, Mn distribution between root and shoot of the ZmNRAMP2-overexpressing lines (OE-1 and OE-4) and WT (ND101) plants grown under contrasted Mn regimes. Maize seedlings were grown in nutrient solution supplied with 1-µM Mn (+Mn) or without Mn (−Mn) for 17 days. Data are presented as means ± sd (n = 6, biological replicates). Significant difference was indicated at *P < 0.05 and **P < 0.01 by Student’s t test (labeling in red and blue for shoots and roots, respectively), and ns, not significant.
Disruption of ZmNRAMP2 does not affect root-to-shoot translocation of Cd
Given that the root-to-shoot Cd translocation mainly relies on vacuolar sequestration of Cd in root (Miyadate et al., 2011; Ueno et al., 2009; Yan et al., 2016), we investigated the role of ZmNRAMP2 in root-to-shoot translocation of Cd besides Mn. The Cd transport activity of ZmNRAMP2 was first examined by heterologous expression in yeast (Figure 7A). Yeast strain BY4741 transformed with empty vector pDR196 showed slightly enhanced growth inhibition in the medium with increased concentration levels of CdCl2. In contrast, the ZmNRAMP2-expressing strain appeared more sensitive to high levels of Cd as did by expressing AtNRAMP1 (a positive control; Thomine et al., 2000), indicating ZmNRAMP2 is able to transport Cd in yeast (Figure 7A).
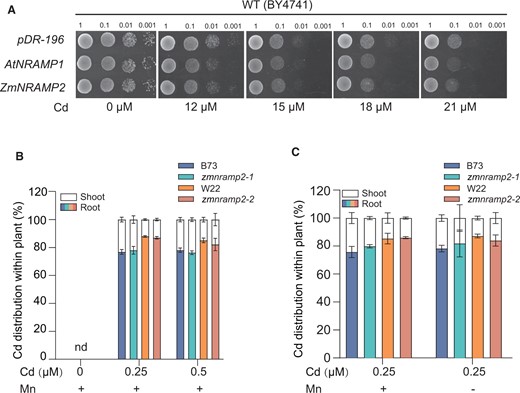
Disruption of ZmNRAMP2 does not affect the root-to-shoot Cd translocation in maize. A, Cd transport of ZmNRAMP2 in yeast. WT yeast strain (BY4741) were transformed with empty vector pDR-196, AtNRAMP1 (positive control), and ZmNRAMP2. Four-fold serial dilutions were dropped on the SD-U ager plate supplemented with gradient Cd concentrations (0, 12, 15,18, or 21 μM), and incubated at 28°C for 3 days. B, Cd distribution between root and shoot of zmnramp2 mutants and the corresponding WT (B73, W22) under increased concentration levels of Cd. Maize seedlings were exposed to nutrient solution containing 0, 0.25-, or 0.5-μM Cd together with 1-μM Mn (+Mn) for 14 days. Data are presented as means ± sd (n = 6, biological replicates). No significant difference was detected by Student’s t test (P < 0.05); nd, not detected. C, Cd distribution between root and shoot of zmnramp2 mutants and the corresponding WT (B73, W22) under contrastive Mn conditions. Maize seedlings were exposed to nutrient solution containing 0.25-μM Cd and 1-µM Mn (+Mn) or without Mn (−Mn) for 14 days. Data are presented as means ± sd (n = 6, biological replicates). No significant difference was detected by Student’s t test (P < 0.05).
To verify whether ZmNRAMP2 functioned in root-to-shoot translocation of Cd in maize, the two zmnramp2 mutants and their corresponding WT were exposed to external Cd with increased concentrations (0, 0.25, or 0.5 μM; Figure 7B). No difference in the root-to-shoot distribution of Cd was determined between the zmnramp2 mutants and WT at all tested Cd levels. Given the relevant contribution of ZmNRAMP2 to root-to-shoot Mn translocation in the Mn-deficient condition, the plants were then subjected to nutrient solutions containing 0.25-μM Cd together with 1-µM Mn (Mn sufficiency) or without Mn (Mn deficiency). Likewise, irrespective of Mn conditions no difference in the root-to-shoot distribution of Cd was observed between the zmnramp2 and WT plants (Figure 7C). Thus, these results indicate that, despite its ability to transport Cd, ZmNRAMP2 is unlikely to be involved in the root-to-shoot Cd translocation in maize plants.
Discussion
In plants, translocation of Mn from roots to aboveground is crucial for providing indispensable Mn to ensure stability and functionality of photosynthetic apparatus (Schmidt et al., 2016; Long et al., 2021). Despite extensive investigations on the Mn uptake and intracellular distribution (Socha and Guerinot, 2014; Alejandro et al., 2020; He et al., 2021), the mechanism of root-to-shoot Mn translocation remains unclear. We show here that a maize tonoplast-localized Mn transporter ZmNRAMP2 makes a substantial contribution to Mn translocation to shoots by mediating the vacuolar Mn release in xylem parenchyma cells in root stele. Furthermore, the ZmNRAMP2-dependent root-to-shoot Mn translocation is crucial for maintaining photosynthetic activity and plant growth in response to Mn deficiency. These findings highlight an essential role of vacuole sequestration process in long-distance metal transport in plants, and open an avenue for genetic improvement of low Mn tolerance in crops.
In this work, the function of ZmNRAMP2 on Mn transport was demonstrated by growth complementation of yeast mutants defective in Mn transport across the plasma membrane (Δsmf1) and endomembrane (Δsmf2; Figure 2). In yeast, ZmNRAMP2 exclusively was localized to the vacuolar membrane (Supplemental Figure S4) that could rescue the growth depression of these two yeast mutants under Mn limitations (Figure 2). We propose that in yeast ZmNRAMP2 can promote release of vacuolar Mn into cytosol for maintaining the cytosolic Mn concentration, which is crucial for yeast growth with limited Mn, particularly for Δsmf1 and Δsmf2 mutants. Given that ZmNRAMP2 was also localized to the tonoplast in maize protoplasts (Figure 1, A–D), it suggests that, in plant cells, similar to AtNRAMP3/4, ZmNRAMP2 also functions in Mn release from vacuole to cytosols. Additionally, ZmNRAMP2 was also shown to transport Cd in yeast (Figure 7A). We then suppose that, besides Mn2+, ZmNRAMP2 can transport a broad range of divalent metals such as Fe2+, Zn2+, and Cd2+ as the other NRAMP members (Socha and Guerinot, 2014; Alejandro et al., 2020).
Radial movement and accumulation of Mn in root stellar cells are prerequisite for xylem loading and subsequent translocation to aboveground. Owing to the barrier of Casparian Strip, the apoplastic Mn entering the root stele requires transport across the plasma membrane of endodermal cells. Indeed, the plasma membrane-localized rice OsNRAMP5 and OsMTP9 and barley HvIRT1 transporters are all reported to participate in Mn uptake and radial movement into root stele (Sasaki et al., 2012; Ueno et al., 2015; Long et al., 2018; Figure 5D). However, this is not the case for ZmNRAMP2 because the overall plant Mn accumulation did not differ among plants of zmnramp2 mutants, ZmNRAMP2-overexpressing lines and their respective WT (Figures 4, C and 6, E). Given the tissue and subcellular localization of ZmNRAMP2 (Figure 1), we assume that vacuolar Mn homeostasis in root stele is capable to determine ability of Mn translocation to shoots.
In plants many intracellular Mn transporters are responsible for subcellular Mn distribution and homeostasis (Alejandro et al., 2020; He et al., 2021). The chloroplast-localized Arabidopsis Chloroplast Manganese Transporter 1 (AtCMT1) and Photosynthesis Affected Mutant71 (AtPAM71) drive Mn across the inner and thylakoids envelop, respectively, delivering Mn to OEC of PSII (Schneider et al., 2016; Zhang et al., 2018; Eisenhut et al., 2018). The Golgi-localized AtPML3 and TGN-localized AtNRAMP2 coordinately maintain Mn homeostasis within Golgi complex which is indispensable for activating Mn-containing enzymes, like Golgi mannosidases and mitochondrial superoxide dismutase (Alejandro et al., 2017; Gao et al., 2018; Yang et al., 2021; Zhang et al., 2021). In plant cells substantial amounts of Mn can be stored in the vacuole which occupies over 80% of the cellular volume. Vacuolar sequestration of Mn is shown to be primarily controlled by the tonoplast-localized Mn transporters, such as AtNRAMP3/4 and AtZIP1 for vacuole release alleviating Mn deficiency, and AtMTP8 and OsMTP8.1/8.2 for vacuole storage preventing Mn toxicity (Lanquar et al., 2010; Chen et al., 2013; Eroglu et al., 2016; Takemoto et al., 2017). Loss of function of some of these vacuolar membrane-localized transporters, like AtNRAMP3, AtZIP1, and AtMTP8, results in changes of Mn concentrations in root or in shoot (Thomine et al., 2003; Milner et al., 2013; Eroglu et al., 2016). This may indicate their roles in root-to-shoot Mn translocation, but direct evidence is still lacking. In contrast, our work provided several lines of evidences to support a crucial role of ZmNRAMP2 in root-to-shoot Mn translocation, particularly under Mn deficiency.
First, the tissue and subcellular localization and biochemical property suggested that in xylem parenchyma cells ZmNRAMP2-mediated release of vacuolar Mn into cytosols for subsequent loading into xylem (Figures 1 and 2). Second, this was verified by zmnramp2 mutants that showed aberrant Mn retention in root stele and significantly reduced Mn concentration in xylem sap (Figure 5, A and C). Third, as a consequence, under Mn-limitation condition, zmnramp2 mutants showed reduced shoot Mn contents but more Mn accumulations in roots (Figure 4, C and D). It was opposite in the ZmNRAMP2-overexpressing lines in which the elevated ZmNRAMP2 expression significantly increased Mn concentrations and contents in shoot but decreased in root (Figure 6, C–E). Despite a broad range of divalent metals being transported, ZmNRAMP2 did not affect root-to-shoot translocation of other metals, such as Zn and Cd (Figure 7, B and C; Supplemental Figure S6, A and B). Thus, we consider the ZmNRAMP2-dependent vacuolar Mn release in xylem parenchyma cells as the key rate-limiting process that determines root-to-shoot Mn translocation ability (Figure 5D), although the plasma membrane-localized Mn xylem loader is still unknown.
Efficient root-to-shoot Mn translocation can improve tolerance of plants to low Mn stress. In maize, the low Mn-tolerant inbred line K22 reveals a higher Mn distribution to shoots along with optimal photosynthetic activity (Fv/Fm) than the sensitive inbred line BY815 under Mn-limited condition (Long et al., 2021). The zmnramp2 mutants with lower Mn allocation to shoots resulted in reduced Fv/Fm and growth arrest, being more sensitive to Mn deficiency than the WT (Figures 3 and 4, C and D). Although the expression level of ZmNRAMP2 was not upregulated by Mn deficiency (Supplemental Figure S2A), the constitutive upregulation of ZmNRAMP2 expression in overexpressing maize lines allowed to improve low Mn tolerance of plants by maintaining photosynthetic activity (Figure 6B). It is expected that the overexpression of the plasma membrane-localized AtNRAMP1 and OsNRAMP5 can improve low Mn tolerance of transgenic plants by enhancing Mn uptake capacity (Cailliatte et al., 2010; Sasaki et al., 2012). However, there are risks on unexpected Cd uptake and accumulation in these plants as these transgene-encoded transporters also transport Cd (Thomine et al., 2000; Sasaki et al., 2012). Notably, ZmNRAMP2 did not affect root-to-shoot Cd translocation in maize plants (Figure 7, B and C), possibly because release of vacuolar Cd by ZmNRAMP2 is negligible, assuming Cd–phytochelatin complex are main existing form of Cd in vacuole (Cobbett and Goldsbrough, 2002; Huang et al., 2012). Therefore, we consider that genetic modification of ZmNRAMP2 can be a promising approach to develop low Mn-tolerant crops.
ZmNRAMP2 was expressed constitutively in root and shoot (Supplemental Figure S2, A and B). In Arabidopsis leaves AtNRAMP3/4 transport Mn from vacuole into cytosol, providing indispensable Mn for photosynthesis (Lanquar et al., 2010). Similarly, ZmNRAMP2 may also confer vacuolar Mn release in leaf cells, because the Fv/Fm values in zmnramp2 mutants were still substantially lower than those in the WT although Mn concentrations remained equal in Mn-deficient leaves (Figures 3, A–C and 4, A). Nevertheless, under −Mn condition phenotypic changes of photosynthetic activity and plant growth in the zmnramp2 mutants and overexpressing lines were mainly attributed to their varied abilities of root-to-shoot Mn translocation (Figures 3, 4, C and D, and 6, B, E, and F). This supports the dominating function of ZmNRAMP2 in Mn allocation from root to shoot, although other possible functionality in the aboveground cannot be excluded.
In conclusion, we identified a tonoplast-localized maize transporter ZmNRAMP2, which plays a crucial role in root-to-shoot translocation of Mn via mediating Mn release from vacuole of root stellar cells, and substantially contributes to plant tolerance to low Mn availability. Considering that ZmNRAMP2 expression was not transcriptionally regulated by Mn deficiency or excess (Supplemental Figure S2), the regulation of ZmNRAMP2 at protein levels may occur in response to internal Mn nutritional status and/or external Mn fluctuations. Recent study shows that phosphorylation of the plasma membrane-localized AtNRAMP1 is the trigger for its Mn-induced endocytosis to responding of Mn excess (Castaings et al., 2021). Furthermore, phosphorylation of the tonoplast-localized transporter AtMTP8 is regulated by CPK- and CBL-CIPK via calcium signal pathway for ensuring precise Mn storage into vacuole (Zhang et al., 2021; Ju et al., 2022). Therefore, identification of upstream regulators and signaling pathways for the tonoplast-localized NRAMP proteins will be of great interest for further research.
Materials and methods
Plant materials
The knockout line zmnramp2-1 (B73 background) was obtained from maize (Zea mays) EMS-mutagenesis mutant collection (http://www.elabcaas.cn/memd) and the knockdown line zmnramp2-2 (W22 background) was from Genetics Cooperation Stock Center (http://maizecoop.cropsci.uiuc.edu/). The transgenic maize lines were generated by overexpressing ZmNRAMP2 in maize inbred line ND101 driven by the ubiquitin promoter. Two independent lines were obtained from the Center for Crop Functional Genomics and Molecular Breeding, China Agricultural University, with the accession number CAUE0190 (CAUID). Primers ZmNRAMP2-ems-F/R and primers 4F/6R (ZmNRAMP2-Mu-4F/6R), TIR6 were used for genotypic identification of zmnramp2-1 and zmnramp2-2, respectively. Primers qPCR-2F/2R (ZmNRAMP2-qPCR-2F/2R) were used for the expression levels analysis of ZmNRAMP2 in zmnramp2-2 and W22. Primers ZmNRAMP2-qPCR-1F/1R were used for the expression levels analysis of ZmNRAMP2 in transgenic maize plants. Sequences of primers were listed in Supplemental Table S1.
Phylogenetic analysis
Peptide sequence alignment was analyzed by ClustalW using default settings and the phylogenetic tree was constructed using the maximum-likelihood method by MEGA5 software.
Hydroponic experiments
Maize seeds were sterilized with 10% H2O2 (v/v) solution for 30 min and rinsed twice with distilled water, followed by immersed in a saturated CaSO4 solution for 12–15 h. The selected seeds were placed at pre-wetted filter papers in dark at 28°C for germination. After 2 days, germinated seeds were vertically placed in filter paper and immersed in distilled water. Uniform maize seedlings were then transfer into 10-L bucket with 1/2 full nutrient solution. After 2 days, plants were transferred into full nutrient solution supplied with 1 µM or without MnSO4, and renewed every 3 days. Plants were grown in a 16-h/28°C and 8-h/22°C day–night rhythm, at a light intensity of 300 μ mol m−2 s−1 and 70% humidity. The full nutrient solution contained 750-µM K2SO4, 650-µM MgSO4·7H2O, 100-µM KCl, 100-µM KH2PO4, 2,000-µM Ca(NO3)2·4H2O, 100-µM Fe(III)-EDTA-Na, 1-µM ZnSO4·7H2O, 0.1-µM CuSO4, 0.035-µM Na2MoO4·2H2O, and 1-µM H3BO3. The pH was adjusted to 5.8. For Cd treatment, maize seedlings were grown in full nutrient solution supplied with 1-µM MnSO4 and 0, 0.25-, 0.5-μM CdSO4 for 14 days. For evaluation the root-to-shoot Cd translocation of plants grown under contrasted Mn levels, the maize seedlings were exposed to full nutrient solution supplied with or without 1-µM MnSO4 and 0.25-μM CdSO4 for 14 days. At harvest, plants were divided into shoots and roots and their dry weights and metal concentrations were determined accordingly.
Gene cloning and plasmid constructions
The open reading frame (ORF) sequence of ZmNRAMP2 (ZmNRAMP2-ORF) was amplified from B73 cDNA library with primers ZmNRAMP2-CDS-F/R. For subcellular localization analysis, the full-length ZmNRAMP2-ORF sequence was cloned into pEZS-NL vector under control of the CaMV 35S promoter using primers ZmNRAMP2-GFP-F/R with BamHI sites, yielding constructs of p35S::ZmNRAMP2-eGFP. To examine the transport activity of ZmNRAMP2 in yeast (Saccharomyces cerevisiae), the full-length ZmNRAMP2-ORF sequence was cloned into yeast expression vector pDR-196 using primers ZmNRAMP2-196-F with EcoRI site and ZmNRAMP2-196-R with BamHI site. The full-length ZmNRAMP2-eGFP-ORF sequence was also cloned into vector pDR-196 using primers ZmNRAMP2-196-F with EcoRI site and ZmNRAMP2-GFP-R with BamHI site. The full-length AtNRAMP1-ORF sequence was cloned into vector pDR-196 using primers AtNRAMP1-196-F with XhoI site and AtNRAMP1-196-R with BamHI site. The full-length AtNRAMP2-ORF sequence was cloned into vector pDR-196 using primers AtNRAMP2-196-F/R with EcoRI sites. Sequences of primers were listed in Supplemental Table S1.
Expression analysis of ZmNRAMP2
Seven-day-old maize seedlings were grown in full nutrient solution containing 1-μM MnSO4 (+Mn), and exposed to Mn deficiency (without Mn, −Mn), or Mn excess (with 100 μM, ++Mn). The root and shoot samples were separately harvested for RNA extraction after the treatment of 0, 1, 3, or 7 days. Total RNA was extracted from plant samples using RNAiso Plus Kit (Takara). The cDNA was generated using the PrimeScript RT reagent Kit (Takara). The RT–qPCR was performed using the primers ZmNRAMP2-qPCR-1F/1R and SYBR Premix Ex Taq (Takara) on CFX96 Touch Real-Time PCR Detection System (Bio-Rad, USA). The maize TUBULIN4 gene (ZmTUB4) served as a reference and its expression level was analyzed using the primers ZmTUB4-qPCR-F/R. Sequences of primers were listed in Supplemental Table S1.
Heterologous expression of ZmNRAMP2 in yeast
Empty vector (pDR-196, negative control) and the constructs of pDR-196-AtNRAMP1/2 (positive control), -ZmNRAMP2, and -ZmNRAMP2-eGFP were electroporation-transformed into yeast mutant strain Δsmf1 (MATa; his3, leu2, met15, ura3, smf1::kanMX4) and Δsmf2 (MATa; his3, leu2, met15, ura3, smf2::kanMX4). The transformants were selected on synthetic medium lacking uracil (SD-U, Clontech). For growth tests, yeast cells were grown in liquid SD-U cultures for 2 days at 28°C. The cultures were adjusted to OD600 = 1, and aliquots of 10-fold serial dilutions were spotted on SD-U agar plates containing 0, 7.5-, 15-, or 20-mM EGTA and incubated for 3 days at 28°C. The yeast strain BY4741(MATa; his3, leu2, met15, ura3) was used for analysis of Cd transport activity of ZmNRAMP2. Serial dilutions were spotted on SD-U agar plates containing 0, 12-, 15-, 18-, or 21-μM CdSO4 and incubated for 3 days at 28°C.
Subcellular localization of ZmNRAMP2 in mesophyll cell protoplasts and in yeast
Leaves of etiolated maize seedlings (inbred B73) grown in dark were digested for preparation of mesophyll cell protoplasts. The fusion constructs of ZmNRAMP2-eGFP were transformed into maize mesophyll cell protoplasts by the polyethylene glycol induced method. After incubation of transformed protoplasts at 23°C in the dark for 12–15 h, the GFP and chloroplast auto-fluorescence signals were observed by a confocal laser scanning microscope with a 40× objective (LSM880; Carl Zeiss). Excitation wavelengths and emission filters were 488 nm/band-pass 495–550 nm for GFP, and 488 nm/band-pass 640–720 nm for chloroplast auto-fluorescence. For the subcellular localization of ZmNRAMP2 in yeast, the transformed yeast with constructs of pDR-196-ZmNRAMP2-eGFP was incubated to OD = 0.8 and observed by a confocal laser scanning microscope (LSM880; Carl Zeiss).
Measurement of metal concentration by ICP–atomic emission spectrometer
At harvest, plant shoot samples were washed twice by deionized water. The root samples were rinsed in deionized water for 5 min, then placed in solution buffer (200-mM CaCl2 and 12.5-mM HBO3, pH 6.0) for 5 min and rinsed twice in deionized water. All samples were dried at 80°C for 3–4 days. Every 0.3-g powder samples were mixed with 6-mL HNO3 and 2-mL 30% H2O2 (v/v), and digested in a microwave (MARS6, CEM, USA) by the steps of 120°C for 2 min, 160°C for 8 min and 180°C for 20 min. The digested samples were diluted to 25 mL, then metal concentration was analyzed by ICP–atomic emission spectrometer (7300DV, PerkinElmer, USA).
Fv/Fm measurements
After adapted in dark for 30 min, the maximal photochemical efficiency of PSII (Fv/Fm) was detected on the youngest fully emerged leaf of plants by a Mn-scanner (NN-Easy55, NutriNostica). The dynamic changes of Fv/Fm were evaluated from 8 DAT to 13 DAT. For imaging fluorometer of Fv/Fm, the 14-day-old plants were adapted in dark for 30 min, and the fluorescence was captured in the youngest fully emerged leaf using an Imaging PAM (Walz, Germany).
Xylem sap collection
The stem of 2-week-old hydroponically grown maize plants were cut off with a clean razor blade about 2 cm above the root. A silicon tube was then placed on the top of stem, and the drops of bleeding sap were collected by a capillary tube for 30 min. Each sampled solutions were collected from three individual seedlings and then digested for element analysis by ICP-MS (7700, Agilent Technologies, USA).
LA–ICP–TOF–MS analysis
Analysis of element distribution in maize roots with LA–ICP–TOF–MS according to Persson et al. (2016). Root segments were cut about 1 cm behind root tip. The samples were gently dried and immersed in liquid paraffin (70°C) for 3 s, made the surface of samples coated with paraffin. The samples were then embedded in pre-cooled frozen embedding medium (OCT, Sakura, Japan), followed by freezing in liquid nitrogen. Cross sections of 30-μm thick were generated for element distribution analysis. For LA–ICP–TOF–MS analysis, the laser NWR 193 was used (New Wave Research; Fremont, CA, USA) with the following settings: Energy: 6.1 J cm−2, Spot size: 10 μm, Repetition rate: 100 Hz. All element signals were obtained with the ICP–TOF–MS (TOFWERK, Zurich, Switzerland) operated in He-mode.
In situ mRNA hybridization
About 1 cm apart from root tip of 10-day-old maize seedlings (inbred B73) were sampled, followed by fixed in formaldehyde–acetic acid–ethanol fixative, consisting of 50% ethanol (v/v), 5% acetic acid (v/v), and 10% formalin containing 37% formaldehyde (v/v). Paraffin-embedded tissues were cut into 8–12 μm sections. Samples on slides were deparaffinized and treated with 20 μg·mL−1 proteinase K for 30 min at 37°C. Preliminary hybridization took place at 37°C for 1h. The hybridization solution containing antisense or sense probe labeled with red fluorescence was dropped at a concentration of 5 ng·uL−1 and incubated at 37°C overnight. Slides were washed 10 min successively in 2×SSC, 37°C; 1×SSC, 37°C and 0.5×SSC at room temperature. The 4',6-diamidino-2-phenylindole dye solution (2 μg·mL−1) were added to monitor root structure. The fluorescence images were observed and collected by a fluorescence microscope (DP25, Olympus). Sequences of probes were listed in Supplemental Table S1.
Statistical analysis
Data were analyzed using software SPSS 18.0 (IBM, Chicago, IL, USA) and Student’s t test with a two-tailed distribution were applied for comparison of sample means at *P < 0.05 or **P < 0.01.
Accession numbers
Sequence data from this article can be found in the GenBank/EMBL data libraries under the following accession numbers: ZmNRAMP2 (GRMZM2G178190), AtNRAMP1 (AT1G80830), and AtNRAMP2 (AT1G47240).
Supplemental data
The following materials are available in the online version of this article.
Supplemental Figure S1. Phylogenetic tree of NRAMP proteins in Arabidopsis, rice, and maize.
Supplemental Figure S2. Transcriptional expression of ZmNRAMP2 in response to Mn deficiency or excess.
Supplemental Figure S3. In situ RNA hybridization in maize roots with the ZmNRAMP2 sense probe.
Supplemental Figure S4. Vacuolar membrane localization of ZmNRAMP2 in yeast.
Supplemental Figure S5. Identification of zmnramp2-1 and zmnramp2-2 mutants.
Supplemental Figure S6. Shoot and root Zn contents and root–shoot Zn distributions of zmnramp2-1, zmnramp2-2, and WT plants grown under contrasted Mn regimes.
Supplemental Figure S7. Shoot and root dry weight of ZmNRAMP2-overexpressing lines and WT plants grown under contrasted Mn regimes.
Supplemental Table S1. List of primers and probes used in this study.
Acknowledgments
We thank Prof. Catherine Curie (Université de Montpellier, F-34060 Montpellier, France) and Dr Pai R. Pedas (Carlsberg Research Laboratory) for providing the yeast mutant. We thank Genetics Cooperation Stock Center for providing the Mu-transposon insertion line UFMu-07918. The transgenic seeds of maize were generated by Center for Crop Functional Genomics and Molecular Breeding of China Agricultural University. We thank Prof. Jingbo Zhang (China Agricultural University) for critical reading of the manuscript and Mr Xiangyu Wu, Jing Hui, Zhi Liu, Zheng Zhao, and Kuihui He (China Agricultural University) for experimental assistance.
Funding
The study was financially supported by the National Key Research and Development Program of China (grant No. 2021YFF1000500) and the National Natural Science Foundation of China. (grant Nos. 31972484; 31801366).
Conflict of interest statement. The authors declare no conflict of interest.
J.G., L.L., and L.Y. conceived and designed the research; J.G., L.L., A.C., X.D., Z.L., and L.Y. did the experiments and analyzed the data; L.C. and J.W. assisted in generation of plant materials; J.G. and L.Y. drafted and finalized the manuscript; L.L. and A.C. revised the manuscript; All authors discussed the results and approved the final manuscript.
The author responsible for distribution of materials integral to the findings presented in this article in accordance with the policy described in the Instructions for Authors (https://dbpia.nl.go.kr/plphys/pages/general-instructions) is: Lixing Yuan ([email protected]).