-
PDF
- Split View
-
Views
-
Cite
Cite
Lihua Zhang, Baiquan Ma, Changzhi Wang, Xingyu Chen, Yong-Ling Ruan, Yangyang Yuan, Fengwang Ma, Mingjun Li, MdWRKY126 modulates malate accumulation in apple fruit by regulating cytosolic malate dehydrogenase (MdMDH5), Plant Physiology, Volume 188, Issue 4, April 2022, Pages 2059–2072, https://doi.org/10.1093/plphys/kiac023
- Share Icon Share
Abstract
The content of organic acids greatly influences the taste and storage life of fleshy fruit. Our current understanding of the molecular mechanism of organic acid accumulation in apple (Malus domestica) fruit focuses on the aluminum-activated malate transporter 9/Ma1 gene. In this study, we identified a candidate gene, MdWRKY126, for controlling fruit acidity independent of Ma1 using homozygous recessive mutants of Ma1, namely Belle de Boskoop “BSKP” and Aifeng “AF.” Analyses of transgenic apple calli and flesh and tomato (Solanum lycopersicum) fruit demonstrated that MdWRKY126 was substantially associated with malate content. MdWRKY126 was directly bound to the promoter of the cytoplasmic NAD-dependent malate dehydrogenase MdMDH5 and promoted its expression, thereby enhancing the malate content of apple fruit. In MdWRKY126 overexpressing calli, the mRNA levels of malate-associated transporters and proton pump genes also significantly increased, which contributed to the transport of malate accumulated in the cytoplasm to the vacuole. These findings demonstrated that MdWRKY126 regulates malate anabolism in the cytoplasm and coordinates the transport between cytoplasm and vacuole to regulate malate accumulation. Our study provides useful information to improve our understanding of the complex mechanism regulating apple fruit acidity.
Introduction
Fruit acidity, as measured by titratable acidity and/or pH, is an important index of fruit organoleptic quality (Etienne et al., 2013; Li et al., 2016) and, with the soluble sugars, affects the fruit flavor. The acidity in a fruit cell is due to the accumulation of organic acids in the vacuole, and malic, citric, and tartaric acid are the main acids found in most ripe fruits (Hulme and Wooltorton, 1957; Zhang et al., 2010). Understanding how the accumulation of organic acids is regulated in fruit cells is thus an important aspect of fruit quality improvement.
Organic acid accumulation in the vacuoles of fruit cells occurs through both metabolism and transport. Organic acids are produced through two pathways: conversion from oxaloacetate (OAA) in the cytosol or production through the tricarboxylic acid cycle in the mitochondria (Etienne et al., 2013). Malate dehydrogenase (MDH), which catalyzes the reversible reaction between OAA and malate, is an important enzyme regulating malate metabolism, and it was reported that MDH in cytoplasm (cyMDH) and mitochondria could regulate the content of malate in apple (Malus domestica) (Yao et al., 2011; Yu et al., 2020; Gao et al., 2022). In addition, two major malate transporters (aluminum-activated malate transporter [ALMT] and tonoplast dicarboxylate transporter (DIT; tDT)) and three proton pumps (vacuolar H+-ATPase, vacuolar pyrophosphatase, and P-ATPase proton pumps) also play essential roles for malate or citrate accumulation (Hurth et al., 2005; Hu et al., 2017; Ma et al., 2018a). Among them, a series of pH genes (PH1–7) involved in vacuole hyper-acidification have been reported in petunia (Petunia hybrida) (De-Vlaming et al., 1983). PH4 encodes an MYB protein that interacts with bHLH transcription factors to reduce vacuolar acidity (Quattrocchio et al., 2006). The mutants of PH6 (ANTHOCYANIN1 alleles) lost their capacity to acidify the vacuole (Spelt et al., 2002). PH3, encoding a WRKY transcription factor, is crucial for the expression of the acid-related proton pump gene PH5 and for vacuole acidification (Verweij et al., 2016). The homologs of these PH genes were confirmed to have roles in determining the acidity in some fleshly fruits, such as citrus (Citrus sinensis) (Strazzer et al., 2019) and grape (Vitis vinifera) (Amato et al., 2019).
In addition, many transcription factors have confirmed roles in regulating organic acid content through affecting the expression of genes related to organic acid metabolism and transport. In tomato (Solanum lycopersicum) fruit, overexpression of the SlAREB1 upregulates the expression of the mitochondrial citrate synthase gene (SlmCS), resulting in an increase in citrate and malate contents (Bastías et al., 2011), and overexpression of SlDREB significantly increases the content of organic acids (Nishawy et al., 2015). In apple, the protein phosphatase MdPP2CH inhibits the activity of the H+ proton pump and Ma1 to reduce malate content (Jia et al., 2018). Some WRKY transcription factors also play roles in the regulation of fruit acidity. PH3, encoding a WRKY protein, can regulate the P-type proton pump PH5 on the vacuolar membrane to control the accumulation of organic acids in citrus fruit (Strazzer et al., 2019), petunia (Verweij et al., 2016), and grape (Amato et al., 2019). In citrus (Citrus reticulata), CiWRKY1 can combine with CiNAC62 to degrade the citrate content in citrus fruit by upregulating the expression of CiACO3 (Li et al., 2017), while in Arabidopsis (Arabidopsis thaliana), AtWRKY46 can negatively regulate the transcription of AtALMT1 and malate content in roots (Ding et al., 2013), while in rice (Oryza sativa). OsWRKY22 binds to the promoter of OsFRDL4 (ferric reductase defective-like 4) and activates its transcription to enhance citric acid secretion (Li et al., 2018a, 2018b). It is possible that members of the WRKY gene family might regulate the content of intracellular acid through various pathway in plants.
Apple is an important fleshly fruit crop in many regions of the world. In apple fruits, malic acid (Ma) accounts for ∼85% of the organic acids, mainly in the dianionic form of malate (Zhang et al., 2010; Ma et al., 2018b). The apple quantitative trait loci for Ma was mapped to a region on linkage group 16 and accounts for 17%–42% of the variation in apple fruit acidity (Xu et al., 2012). The Ma1 gene on chromosome 16, encoding an ALMT9 homolog, has been considered the key gene controlling apple fruit acidity (Bai et al., 2012; Li et al., 2020a, 2020b). A single-nucleotide polymorphism at base 1,455 leads to the truncation of the Ma1 protein by 84 amino acids. The ma1 mutant disrupts malate transport into the vacuole (Li et al., 2020a, 2020b). Generally, apples of genotype ma1/ma1 have significantly lower acidity at fruit maturity than those of genotypes Ma1/ma1 and Ma1/Ma1 (Xu et al., 2012; Ma et al., 2015b). Ma1 expression can be regulated by the transcription factors MdMYB73 (Hu et al., 2017; Zhang et al., 2020a, 2020b), MdMYB44 (Jia et al., 2021), and MdbHLH3 (Yu et al., 2020) to acidify apple vacuoles. Additionally, malate content in apple could be regulated by cyMDH (Yao et al., 2011) and proton pump H+-ATPase (Ma et al., 2018a). However, some apple plants with the ma1/ma1 genotype have high malate content (Ma et al., 2015b). Understanding the regulatory mechanism of malate accumulation in ma1/ma1 genotype apple is thus of primary importance for fruit quality improvement.
In a previous study, we found that two cultivated apple varieties, “Belle de Boskoop” (BSKP) and “Aifeng” (AF), were homozygous recessive at the Ma locus (ma1/ma1), but the “BSKP” fruit showed high acidity and malate content, while the acidity and malate content of “AF” was very low (Ma et al., 2015b). After transcriptome differential expression analysis, an acidity-related candidate gene, MdWRKY126, was identified. MdWRKY126 is a homolog of the WRKY transcription factor PhPH3 and showed significantly higher expression in “BSKP” fruit than in “AF” fruit (Ma et al., 2018a). Here, we report that MdWRKY126 directly binds to the promoter of the malate metabolism gene MdMDH5, activating its expression and increasing apple fruit acidity. Our findings improve our understanding of the molecular mechanism of organic acid accumulation in apple.
Results
Identification, homology analysis, and expression of candidate gene MdWRKY126 and subcellular localization of its protein
In our previous study, 77 candidate genes involved in apple fruit acidity were found in transcriptome data analyzed by digital gene expression of acidic (“BSKP”) and nonacidic (“AF”) apple fruits. Both varieties were genotype ma1/ma1 at the Ma locus and were analyzed 30 and 90 days after full bloom (DAFB) (Ma et al., 2018a). The candidate gene MdWRKY126 was differentially expressed between the two varieties. MdWRKY126 belongs to group I in the WRKY transcription factor family and harbors 2 WRKY domains and a zinc finger motif (Figure 1B; Meng et al., 2016). MdWRKY126 is located on chromosome 11 of the Malus genome, and its open-reading frame (ORF) contains 1,719 bases encoding 572 amino acids. GFP-tagged MdWRKY126 protein localized to the cellular nucleus (Figure 1C). Phylogenetic analyses of MdWRKY126 and its homologous proteins from other species showed that MdWRKY126 was homologous with PhPH3 (Figure 1A), which was reported to regulate petal cell acidity in petunia (Verweij et al., 2016).
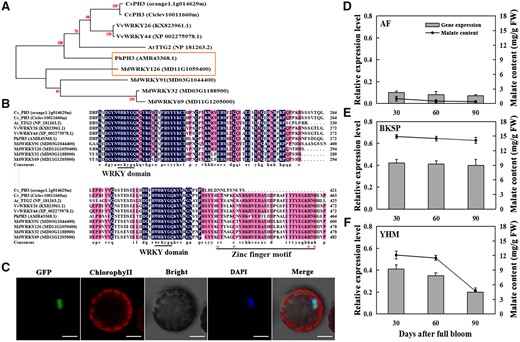
Localization and phylogenetic analysis of MdWRKY126 and its expression in apple fruit with different acidity levels. A, Phylogenetic analysis of MdWRKY126. The WRKY126 protein sequences of six different species were obtained to build a phylogenetic tree using the maximum likelihood method on the basis of 1,000 bootstrap analyses of the MEGA version 6.0 software. The bootstrap values were provided near branched lines. For each protein, a GenBank accession number or gene identifier was displayed in bracket. The specific species involved as follows: Cs, C. sinensis; Cc, Citrus clementina; Vv, V. vinifera; At, A. thaliana; Ph, P. hybrida; Md, M. domestica. B, Sequence alignment of MdWRKY126. The WRKY domain was blasted using DNAMAN version 6.0 software, the conserved amino acid residues were displayed in dark blue. The WRKYGQK motif was underlined, cysteine and histidine forming the zinc finger were marked with red asterisk and underlined. C, Subcellular localization of MdWRKY126. 35Spro::MdWRKY126::GFP was expressed transiently in protoplasts of Arabidopsis leaves with 35Spro::GFP alone as control. Fluorescence was observed under a confocal laser scanning microscope (LSM 710; Carl Zeiss, Oberkochen, Germany) equipped with a water immersion objective (100×/1.40). GFP excitation was performed using a 488 nm solid-state laser, fluorescence was detected at 498–535 nm, and the intensity and gain were 11.9% and 800, respectively. DAPI excitation was performed using a 405 nm solid-state laser, and fluorescence was detected at 430–470 nm, and the intensity and gain were 6.61% and 800, respectively. Stacks of 1,024 × 1,024 pixels (pixel size of 0.363 × 0.363 μm) optical section were generated with a Z-interval of 0.5 μm. DAPI served as a nuclear dye. Scale bars, 20 µm. D–F, Malate content and MdWRKY126 expression in fruit of “BSKP,” “AF,” and “YHM” during development. The fruits were collected 30, 60, and 90 DAFB. GC–MS was used to measure the malate content (line graph), and quantitative expression analysis of MdWRKY126 was conducted in fruit of “BSKP,” “AF,” and “YHM” (bar graph). Values are presented as the mean ± SD of three repetitions.
The expression levels of MdWRKY126 in acidic and nonacidic apple fruits were analyzed. RNA-seq analysis indicated that MdWRKY126 was expressed about eight times higher in “BSKP” fruit than in “AF” fruit at the two stages tested (Supplemental Figure S1). Reverse transcription-quantitative PCR (RT-qPCR) analysis of MdWRKY126 in fruits of “BSKP,” “AF,” and “Yanhongmei” (YHM, which is genotype Ma1/Ma1 at the Ma locus and shows a low acidity in the ripening fruit; Ma et al., 2015b) showed similar results, with high mRNA expression levels of MdWRKY126 in fruit of “BSKP” and low expression levels in fruit of “AF” at the three developmental stages tested (Figure 1, D and E). In “YHM” fruit, the expression level of MdWRKY126 decreased with ripening (Figure 1F). The malate contents in the fruits of “BSKP,” “AF,” and “YHM” corresponded to the MdWRKY126 expression levels through development (Figure 1, D–F). These results indicated that MdWRKY126 might play a key role in regulating malate content during fruit development.
Overexpression of MdWRKY126 increases malate accumulation
To investigate the role of MdWRKY126 in regulating organic acid content in apple, its CDS was cloned into the pCAMBIA2300 vector to generate MdWRKY126 overexpressing apple calli (Figure 2A) and tomato lines (Figure 2G). In addition, an interference vector, pK7GWIWG2(I)-MdWRKY126, was constructed and transformed into apple calli to obtain MdWRKY126 silenced calli lines (Figure 2A). The contents of malate and citrate were measured in the MdWRKY126 transgenic lines. In the MdWRKY126 overexpressing apple calli and tomato fruit, the content of malate was significantly increased (Figure 2, C and I). On the other hand, in MdWRKY126 silenced calli, the malate content was significantly lower compared with the control, while the content of citrate showed little change (Figure 2C). To further investigate the effect of MdWRKY126 on organic acid content, a pTRV2-MdWRKY126 virus-induced gene silencing vector and a pCAMBIA2300-MdWRKY126 overexpression vector were transiently transformed into “Fuji” apple fruits respectively (Figure 2D). Overexpression of MdWRKY126 increased malate content in flesh of “Fuji” apple, while the silencing of MdWRKY126 decreased it (Figure 2F), similar to the results in the transgenic apple calli. Only low levels of citrate were detected, and there was no significant (ns) difference in citrate content between MdWRKY126 overexpression and silenced apple samples compared with the control (Figure 2F). These results supported our hypothesis that MdWRKY126 positively regulated malate accumulation in apple.

Phenotype and acid content in control and MdWRKY126 transgenic lines. A–C. Expression of MdWRKY126 in “Orin” calli. A, The complete cDNA and specific antisense cDNA fragments of MdWRKY126 were inserted into the pCAMBIA2300 and pK7GWIWG2(I) vector, respectively, for gene overexpression (MdOW-1,2,3) or silencing (MdiT-1,2,3). The empty vectors pCAMBIA2300 (P2300) and pK7GWIWG2(I) (PK7) were used as controls. Scale bar, 1 cm. B, The mRNA relative expression levels of MdWRKY126 and (C) the malate and citrate contents in control and transgenic calli. Three biological replicates were analyzed. The values are presented as the mean ± SD. Different letters over each bar indicate significant difference as determined via one-way ANOVA at P < 0.05. D–F, Transient expression of MdWRKY126 in “Fuji” apple fruit. D, The complete cDNA of MdWRKY126 gene were inserted into the pCAMBIA2300 vector for gene overexpression (MdOW-1,2,3), while the specific antisense cDNA fragment of MdWRKY126 was cloned into viral vector pTRV2 for silencing (MdTW-1,2,3). The empty vector pCAMBIA2300 (P2300) and pTRV2 (TRV) served as controls respectively. Scale bar, 1 cm. E, The mRNA relative expression levels of MdWRKY126 and (F) the malate and citrate contents in control and transgenic apple fruit. Three biological replicates were analyzed. The values are presented as the mean ± SD. Different letters over each bar indicate significant difference as determined via one-way ANOVA at P < 0.05. G–I, Heterologous expression of MdWRKY126 in “Micro Tom” tomato fruit. G, Phenotypes of MdWRKY126 overexpressed tomato (SlOW-1,2,3) with the fruit overexpressed empty vector pCAMBIA2300 (P2300) as control. Scale bar, 1 cm. H, The mRNA relative expression levels of MdWRKY126 and (I) the malate and citrate contents in control and transgenic tomato fruit. Three biological replicates were analyzed. The values are presented as the mean ± sd. Different letters over each bar indicate significant difference as determined via one-way ANOVA at P < 0.05.
Expression levels of malate metabolism- and transport-associated genes in MdWRKY126 overexpression calli
The anabolism of Ma and the transport of malate to the vacuole are essential for malate accumulation in plant cells (Sweetman et al., 2009). To explore the downstream functional genes of MdWRKY126, the expression levels of genes related to malate synthesis and transport, including MDHs, transporters, and proton pumps (as shown in Supplemental Figure S2), were analyzed in MdWRKY126 overexpression apple calli. Five transporter, three proton pumps, and three MDH genes showed increased mRNA expression level in three MdWRKY126 transgenic lines compared with control (Figure 3, A–C). To further detect the relationship between mRNA expression levels of the ˃11 upregulated malate-related genes and malate content in apple fruit, RT-qPCR was carried out in “AF,” “BSKP,” and “Fuji” apple fruit. Six genes were highly expressed in “BSKP” fruit and low expressed in “AF” fruit, including MdMDH5, MdMDH1, MdMDH18, tonoplast dicarboxylate transporter 1 (MdtDT1), vacuolar proton ATPase A3-1 (MdVHA-A3-1), and vacuolar H+ pyrophosphatase 3 (MdAVP3) ), similar to MdWRKY126 expression. However, others (MdVHA-C2-2, dicarboxylate transporter 1-2 [MdDIT1-2], MdDIT1-3, MdALMT9-3, and MdALMT6-1) were not expressed or had very low expression in apple fruit (Figure 3D and Supplemental Figure S3). These results suggested that MdMDH5, MdMDH1, MdMDH18, MdtDT1, MdVHA-A3-1, and MdAVP3 might be candidate genes regulated by MdWRYK126 in apple fruit.
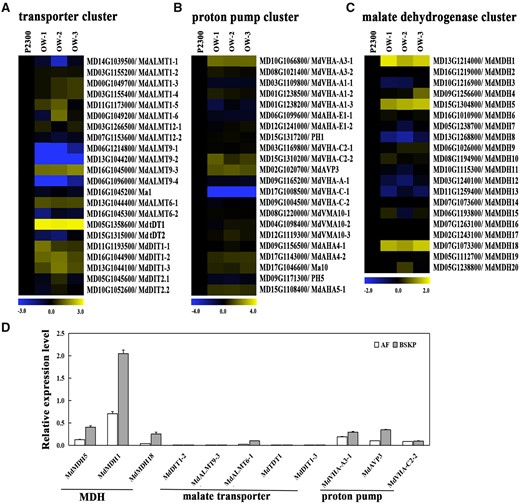
The expression level of genes involved in Ma metabolism and malate transport in MdWRKY126-overexpressing apple calli. A–C, Transcript accumulation of acid-associated genes in the control (P2300) and three MdWRKY126 overexpression apple calli (OW-1, OW-2, and OW-3). A, Heat map of the expression levels of malate transporter genes in MdWRKY126 transgenic calli compared to control. B, Heat map of the expression levels of proton pump genes in MdWRKY126 transgenic calli compared with control. C, Heat map of the expression levels of MDH genes in MdWRKY126 transgenic calli compared with control. AHA-E1, vacuolar ATP synthase subunit E1; VMA, vacuolar membrane ATPase. The fold change was specified as a log2 value and the data of P2300 was set as 1 for each gene. D, The mRNA relative expression level of malate-related genes (upregulated in MdWRKY126 transgenic calli) in “AF” and “BSKP” apple fruit. For RT-qPCR, gene transcript levels were normalized to those of MdActin and the relative expression levels of each gene were calculated with the ddCT method. Values are presented as the mean ± sd on the basis of three repetitions.
MdWRKY126 binds to the promoter of MdMDH5 and activates its transcription
Then the promoter regions (2,000 bp upstream of the translation start site) of malate-associated genes MdMDH5, MdMDH1, MdMDH18, MdtDT1, MdVHA-A3-1, and MdAVP3 were analyzed using PlantCare (http://bioinformatics.psb.ugent.be/webtools/plantcare/html/) for cis-acting elements. W-box elements were only observed in the promoter regions of MdMDH5, MdAVP3, and MdMDH1 (Supplemental Figure S4A), while WRKY transcription factors are known to function by binding to the W-box element (TTGACC/T) in promoters of their downstream target genes (Rushton et al., 2010). Then, MdMDH5, MdAVP3, and MdMDH1 were picked to verify whether MdWRKY126 protein bound to their promoters via an in vivo and in vitro system.
First, cross-linked chromatin samples were extracted from 35Spro::MdWRKY126-GFP transgenic apple calli for chromatin immunoprecipitation PCR (ChIP-PCR) assay. The results showed that about 6.8-fold enrichment was observed in the promoter of MdMDH5, while ns enrichment was detected in the promoters of MdAVP3 and MdMDH1 genes compared with the control (Figure 4B). Then, a luciferase (LUC) activation assay was performed to test the regulation of MdWRKY126 on the transcript activities of MdMDH5, MdMDH1, and MdAVP3 promoters. The results showed that the co-expression of MdMDH5pro::LUC and 35Spro::MdWRKY126 produced a significantly increased luminescence signal compared with the other combinations in tobacco (Nicotiana benthamiana) leaves (Figure 4, E and F) and apple calli (Supplemental Figure S5, B and C), but MdWRKY126 expression had ns influence on LUC expression driven by MdMDH1 or MdAVP3 promoter (Supplemental Figure S4). These results showed that MdWRKY126 bound to the promoter region of MdMDH5 in vivo, but not MdMDH1 and MdAVP3 promoters.
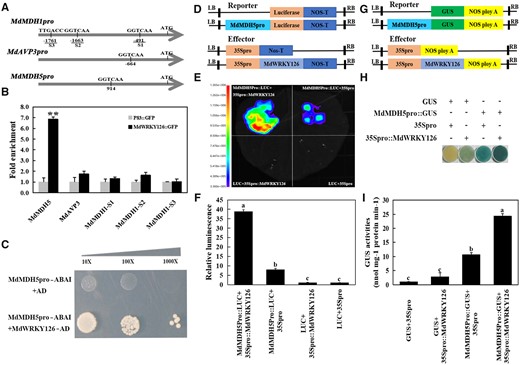
MdWRKY126 binds to the promoter of MdMDH5. A, W-BOX elements in the upstream promoter region of three genes (MdMDH5, MdAVP3, and MdMDH1) upregulated in MdWRKY126 overexpressing calli. B, ChIP-PCR demonstrated the binding of MdWRKY126 to the MdMDH5 promoter in vivo. Cross-linked chromatin samples were extracted from calli carrying 35Spro::MdWRKY126-GFP (GFP-overexpressing calli as control) and sedimented using an anti-GFP antibody. The ChIP test was repeated three times and the eluted DNA fragments in each ChIP were considered one biological replicate for the amplification of promoter sequences near the W-box. Significant difference (**P < 0.01) was calculated by Student’s t test. C, Y1H assay showed that MdWRKY126 bound to the MdMDH5 promoter containing one W-box motif. The co-transformation of empty vector PGADT7 and the MdMDH5 promoter (PGADT7+MdMDH5Pro-pAbAi) into yeast cells served as control. The screening concentration of ABA was 300 ng/mL. D–F, LUC assay in tobacco (N. benthamiana) leaves revealed that MdWRKY126 interacted with MdMDH5 promoter. D, Schematic diagram of the effector vector (35Spro::MdWRKY126) and Luc reporter vector (MdMDH5pro::Luc). E, Fluorescence imaging of tobacco leaves expressing both 35Spro::MdWRKY126 and MdMDH5pro::Luc showed enhanced fluorescence signal, revealing that MdWRKY126 could activate the expression of MdMDH5. F, Quantitative analysis of luminous intensity for the transformants, with the fluorescence intensity of 35Spro+Luc set as 1. Values are presented as the mean ± sd on the basis of three repetitions. Different letters over each bar indicate significant difference as determined via one-way ANOVA at P < 0.05. G–I, GUS reporter assay indicated that MdWRKY126 promoted the expression of MdMDH5. G, Schematic diagram of the GUS effector vector (35Spro::MdWRKY126) and GUS reporter vector (MdMDH5pro::GUS). H, Agrobacterium containing 35Spro::MdWRKY126 and MdMDH5pro::GUS constructs were transiently transformed into apple calli for staining to verify the relationship between MdWRKY126 and the MdMDH5 promoter. I, Relative quantitative analysis of GUS activity for the transformants, with the GUS activity of 35Spro + GUS set as 1. Values are presented as the mean ± sd on the basis of three repetitions. Different letters over each bar indicate significant difference as determined via one-way ANOVA at P < 0.05.
In vitro, yeast one-hybrid (Y1H) assay was performed to investigate the binding of MdWRKY126 and the promoter of MdMDH5. Transformants with MdMDH5pro-pAbAi and MdWRKY126-AD grew well on SD/Leu + 300 ng/mL ABA plates, while the control containing MdMDH5pro-pAbAi and pGADT7 vector did not grow (Figure 4C), which indicated that MdWRKY126 could bind to the MdMDH5 promoter.
Furthermore, a β-glucuronidase (GUS) reporter assay was also carried out to test that MdWRKY126 activated the expression of MdMDH5. The GUS staining level and GUS content were significantly higher in apple calli cells containing MdMDH5pro::GUS and 35Spro::MdWRKY126 than in cells containing MdMDH5pro::GUS and 35Spro (Figure 4, H and I). Therefore, overexpression of MdWRKY126 indeed triggered an increase in expression of MdMDH5 by binding to the promoter of MdMDH5.
MdWRKY126 mainly increased malate content by upregulating the expression level of MdMDH5
To characterize the biological role of MdMDH5 in malate accumulation, an MdMDH5::GFP construct was transiently transformed into Arabidopsis leaf protoplasts for observation of subcellular localization. The result demonstrated that MdMDH5 was targeted to the cytoplasm in vivo (Figure 5A). Subsequently, calli overexpressing MdMDH5 and calli carrying the empty vector pCAMBIA2300 (control) were generated (Figure 5B). RT-qPCR evaluation showed that the mRNA expression levels of MdMDH5 in overexpressing MdMDH5 calli were about four to five times higher than that of the control (Figure 5C). The accumulation of malate was significantly increased in the MdMDH5 overexpressing apple calli (Figure 5D). To further verify the function of MdMDH5 in promoting malate synthesis, the redox activity of MDH was examined in MdMDH5 and MdWRKY126 overexpressing calli and apple flesh. The reductive activity of MDH in both calli and fruit overexpressing MdMDH5 or MdWRKY126 were significantly enhanced compared to the control, while the difference in their oxidative activity was not obvious (Figure 5, E–H). These results indicated that overexpression of MdMDH5 or MdWRKY126 could increase the reductive activity of MDH, thereby accelerating the conversion of OAA to malate.
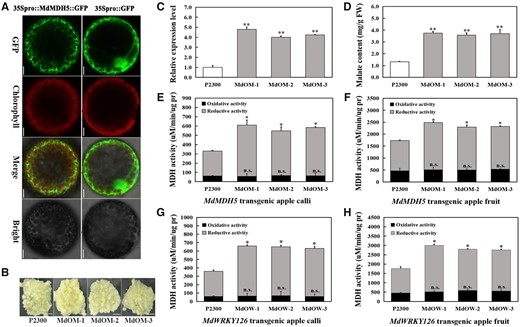
Functional analysis of MdMDH5. A, Subcellular localization of 35Spro::MdMDH5::GFP expressed transiently in protoplasts of Arabidopsis leaves with 35Spro::GFP alone as control. Scale bars, 20 µm. B, Phenotype of control and three MdMDH5 transgenic calli lines. Overexpression vector pCAMbia2300-MdMDH5 and empty pCAMbia2300 vector were transformed into apple calli for MdMDH5 overexpressing lines (MdOM-1,2,3) and control (P2300). Scale bars, 1 cm. C, The mRNA relative expression level of MdMDH5 in control and MdMDH5 overexpressing calli. D, The malate content in control and MdMDH5 overexpressing calli. E–F, The oxidative and reductive activities of MDH in MdMDH5 overexpressing calli (E) and apple fruits (MdOM-1,2,3) (F) with tissues carrying pCAMbia2300 as controls (P2300). G–H, The oxidative and reductive activities of MDH in MdWRKY126 overexpressing calli (G) and apple fruits (MdOW-1,2,3) (H) with tissues carrying pCAMbia2300 as controls (P2300). Values are presented as the mean ± sd of three repetitions. Significant difference (P > 0.05; *P < 0.05; **P < 0.01) were calculated by Student’s t test.
To clarify that MdWRKY126 increased malate content by regulating the expression of MdMDH5, three recombinant plasmids, pCAMBIA2300-MdMDH5, pTRV2-MdMDH5, and pCAMBIA2300-MdWRKY126+pTRV2-MdMDH5, were individually infiltrated into apple calli, with the empty vectors pCAMBIA2300 and pTRV2 as controls (Figure 6A). The overexpression of MdMDH5 triggered the accumulation of malate while the suppression of MdMDH5 expression resulted in a decrease in malate content. When MdMDH5 was silenced in MdWRKY126-overexpressing calli, malate content was significantly lower than in calli overexpressing MdWRKY126 alone (Figure 6C). A similar experiment was conducted in “Fuji” apple fruit. MdMDH5 overexpression and interference constructs and a combination containing pCAMBIA2300-MdWRKY126 and pTRV2-MdMDH5 were injected into apples separately. Apples were sampled 4 days after injection transformation (Figure 6D). After injection with pCAMBIA2300-MdMDH5, the expression of MdMDH5 was elevated by about four times compared with the control, while injection with pTRV2-MdMDH5 reduced MdMDH5 expression by about half (Figure 6E). Transient overexpression of MdMDH5 also increased the malate content, while transient interference significantly decreased the accumulation of malate in “Fuji” apple fruit (Figure 6F). Compared with MdWRKY126 overexpressing apple, the malate content was significantly reduced in apple co-transformed with pCAMBIA2300-MdWRKY126 and pTRV2-MdMDH5 (Figure 6F). These results indicated that the function of MdWRKY126 in malate accumulation was highly dependent on MdMDH5 in apple calli and fruit.
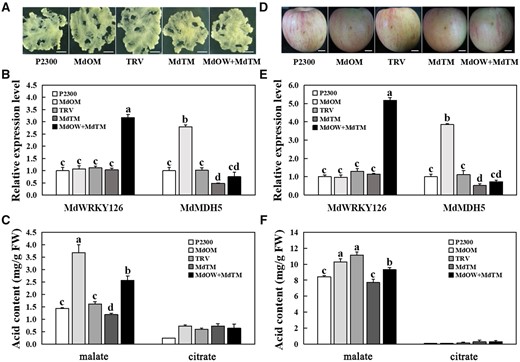
MdWRKY126 facilitates malate accumulation via increasing MdMDH5 expression level. A, Expressions of MdWRKY126 and MdMDH5 in “Orin” calli. The complete cDNA of MdWRKY126 and MdMDH5 genes were inserted respectively into pCAMBIA2300 vector for MdOW and MdOM, whereas the specific antisense cDNA fragments of MdMDH5 were introduced into the pTRV2 vector for suppression (MdTM). The empty vector pCAMBIA2300 (P2300) and pTRV2 (TRV) served as controls respectively. Scale bar, 1 cm. B, The mRNA relative expression levels of MdWRKY126 and MdMDH5 in control and transgenic calli. C, The malate and citrate contents in control and transgenic calli. D, MdOM MdTM as well as the co-transformed of pCAMBIA2300-MdWRKY126+pTRV2-MdMDH5 (MdOW+MdTM) in “Fuji” apple fruit. Scale bar, 1 cm. E, The mRNA relative expression levels of MdWRKY126 and MdMDH5 in control and transgenic apple fruit. F, The malate and citrate contents in control and transgenic apple fruit. Values are presented as the mean ± sd of three repetitions. Different letters over each bar indicate significant difference as determined via one-way ANOVA at P < 0.05.
Quantitative detection in “BSKP” and “AF” apple fruit showed that the expression level of MdMDH5 in “BSKP” fruit was about three times higher than that in “AF” fruit (Supplemental Figure S6) similar with MdWRKY126, which provided additional strong evidence that MdWRKY126 combined with the MdMDH5 promoter to regulate acid content in apple fruit. This result also provided an explanation for why “BSKP” is a high-acid variety while “AF” is a low-acid variety.
Discussion
Malate is a crucial factor affecting the flavor quality of apple fruit (Bugaud et al., 2011). Since flavor quality directly determines the quality of fresh apples, the quality of fruit wine and the consumer demand for different apple varieties (Ma et al., 2015a; Huang et al., 2018), it is important to analyze the mechanism through which malate accumulates in apple fruit. Current studies on apple fruit acidity mainly focus on the key gene Ma1 (Bai et al., 2012; Ma et al., 2015b; Li et al., 2020a, 2020b). In our study, a candidate gene for controlling fruit acidity independent of Ma1, MdWRKY126, was selected from the varieties “BSKP” and “AF,” which are both homozygous recessive ma1/ma1 mutants but show significant differences in malate content during fruit development. In addition, although “YHM” is Ma1/Ma1 at the Ma locus, the RT-qPCR result in our previous study showed that Ma1 gene expression increased, while malate content decreased during fruit development of “YHM” (Ma et al., 2015b). So, we speculated that the increase of malate content was also independent of Ma1 during fruit development of “YHM,” just as in “BSKP” and “AF.” Interestingly, the change trends of MdWRKY126 mRNA expression level were consistent with the changes of malate content in fruits of these three varieties at three developmental stages, suggesting that MdWRKY126 might regulate malate content independent of the Ma1 pathway.
WRKY transcription factors are multifunctional transcription factors that influence anthocyanin accumulation (An et al., 2019; Liu et al., 2019), sugar and acid quality of fruit (Chen et al., 2019; Strazzer et al., 2019), fruit ripening and senescence (Jiang et al., 2014; Chen et al., 2017), and response to biological and abiotic stresses (Ren et al., 2010; Sun et al., 2019) during environmental and developmental events. In fact, the regulation of the proton pump genes PH5 and PH1 by the WRKY transcription factor PH3 has been reported to be an important regulatory mechanism of vacuolar acidification in a variety of plants. In the juice vesicles of high-acid citrus, the expression levels of PH3, PH1, and PH5 genes were high, while their expression levels were low in juice vesicles of low-acid citrus (Strazzer et al., 2019). In leaf cells of grapevine, the interference of VvWRKY26 (PH3) led to a significant downregulation of PH5 and PH1 expression (Faraco et al., 2014; Verweij et al., 2016). A similar control mechanism has been demonstrated in petunia (Amato et al., 2019). In this study, WRKY126 (a PhPH3 homolog) influenced the vacuolar acidity of apple fruit.
Based on the above studies, we speculated that PH1 and PH5 genes may also play roles in the regulation of apple fruit acidity, and there may be a positive association of the expression of PH3 and PH1/PH5 in fruits. We determined the expression of the homologous genes MdPH1 and MdPH5 in MdWRKY126 overexpressing calli. Quantitative determination showed that the increased expression level of MdWRKY126 did not enhance the transcription of MdPH1 or MdPH5 (Supplemental Figure S7), which suggested that MdWRKY126 may not influence the acidity of fruit vacuoles through MdPH1 or MdPH5 in apple fruit.
Considering that the accumulation of malate is influenced by both its anabolism and transport (Etienne et al., 2013), we focused on the MDH, proton pump, and transporter genes whose mRNA expression levels were increased in MdWRKY126 overexpressed calli. Combined with data on physical interactions, we found a MdWRKY126 downstream target gene MdMDH5 that encoded a cytoplasmic NAD (nicotinamide adenine dinucleotide)-dependent MDH. MDH is a conserved enzyme that plays an important role in catalyzing the reversible conversion between malate and OAA. There are four types of MDHs that differ in their subcellular localization and co-factor (Schreier et al., 2018). Among them, cyMDH has been demonstrated to mainly catalyze the synthesis of Ma in apple (Yao et al., 2011), wheat (Triticum aestivum) (Pastore et al., 2003; Ding and Ma, 2004), alfalfa (Medicago sativa) (Miller et al., 1998), pineapple (Ananas comosus) (Cuevas and Podestá, 2000), and cordifolia (Aptenia cordifolia) (Trípodi and Podestá, 2003). In MdMDH5 overexpressing calli and apple fruit, the cytoplasmic MDH showed a much higher affinity for OAA and NADH than for malate and NAD+. This indicated that MDH in MdMDH5 overexpression lines preferred to catalyze the reaction toward Ma synthesis in vitro, which was supported by previous studies (Yao et al., 2011; Wang et al., 2016). Although enzyme kinetics demonstrated that MDH had the ability to catalyze Ma production in vitro, the conversion of OAA to Ma may be affected by other metabolic pathways or physiological environment in plants. However, in our experiment, the overexpression of MdMDH5 significantly increased the malate accumulation in apple calli and “Fuji” fruit, accompanied by the upregulated MDH reduction activity, indicating that MdMDH5 mainly catalyzed the synthesis of Ma in both apple fruit and calli.
In addition to MdMDH5, the expression of malate-associated proton pump and transporter genes were also increased in the MdWRKY126 overexpression transgenic calli (Figure 3), suggesting that MdWRKY126 not only affected the anabolism of malate, but directly or indirectly regulated genes related to malate transport. Yu et al. (2020) reported similar results for the transcription factor MdbHLH3, which directly activated the transcriptional expression of MdcyMDH and also increased the expression of acid-related genes such as MdVHA-B1/B2 and MdtDT. We hypothesized that this mechanism was conducive to maintaining malate homeostasis in cytoplasm of MdWRKY126 overexpressing apple calli and fruit cells. Overexpression of MdWRKY126 directly activated the transcription of MdMDH5, resulting in the accumulation of large amounts of malate in the cytoplasm. Excessive accumulation of malate in the cytoplasm could cause damage to plant cells, which might serve as a signal to accelerate the efficiency of malate transporters and proton pumps in the vacuolar membrane. A large amount of malate2− and H+ entering the vacuole and combining to form Ma would acidify the vacuole, which would not only relieve the excessive pressure of malate in the cytoplasm, but would also promote the synthesis of a new round of malate in the cytoplasm.
Here, we crafted a model to elucidate the molecular mechanism of malate accumulation in apple (Figure 7). MdWRKY126 enhances MdMDH5 transcription capability by binding to its promoter. The enhanced enzyme activity of MDH will increase the conversion of OAA to malate, resulting in a large accumulation of malate in the cytoplasm. In addition, the overexpression of MdWRKY126 increases the mRNA expression of malate transporters and proton pumps located on the vacuolar membrane, which was conducive to the transfer of the accumulated malate into the vacuole and the re-accumulation of malate in the cytoplasm.
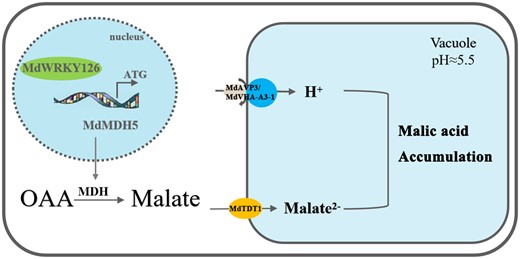
Working model for MdWRKY126 function on the malate regulation in apple fruit. MdWRKY126 activates MdMDH5 transcription and enhances the activity of MDH, resulting in increased accumulation of malate in the cytoplasm. In addition, MdWRKY126 increases the expression of malate transporters MdtDT1 and proton pumps MdAVP3 and MdVHA-A3-1, which contributes to delivery of the accumulated malate into the vacuole, then promotes re-accumulation of malate in the cytoplasm.
Materials and methods
Plant materials and growth conditions
Apple calli (M. domestica cv. “Orin”) were grown on Murashige and Skoog solid medium plus 1.0 mg/L 2,4-dichlorophenoxyacetic acid and 1.0 mg/L 6-benzyladenien (6-BA) at 25°C under dark conditions and were sub-cultured every 3 weeks until at least three generations before Agrobacterium tumefaciens-mediated genetic transformation.
Apple trees (M. domestica cv. “Gala,” “Fuji”) were planted in a garden at Northwest A&F University, Yangling (34°20′N, 108°24′E), Shaanxi, China and apple trees (M. domestica “AF,” “BSKP,” and “YHM”) were planted in the Xingcheng Institute of Pomology of the Chinese Academy of Agricultural Sciences, Xingcheng, Liaoning, China at a spacing of 2.5 m × 0.5 m. Apple fruit of “Gala” was collected 120 DAFB for gene cloning, and apple fruit of “AF,” “BSKP,” and “YHM” were sampled at 30, 60, and 90 DAFB for the RT-qPCR assay. Apple fruit of “Fuji” was harvested and treated 150 DAFB for virus-induced vector injection and gene expression analysis. For each replicate of various apple fruits, six apples of similar growth were collected from three trees, and frozen in liquid nitrogen and stored at –80°C.
Control and MdWRKY126-transformed tomato (S. lycopersicum cv. “Micro Tom”) seedlings were grown in plastic pots filled with a mixture of soil, sand, and organic-mineral fertilizer (3:1:1, v:v:v) at 23°C and 70% relative humidity for a 16-h light/8-h dark cycle. Mature tomato fruits were collected 45 DAFB for determination of acid content.
Sequence alignment and phylogenetic tree analysis
To identify WRKY126 gene homology of petunia (P. hybrida) and apple, we searched the M. × domestica genome, GDDH13 version 1.1 (https://www.rosaceae.org) with a BLASTp (protein–protein BLAST) analysis and confirmed homology by phylogenetic analysis via maximum likelihood method on the basis of 1,000 bootstrap analyses of the MEGA version 6.0 software (http://www.megasoftware.net/; Strazzer et al., 2019). Structural motif annotation of WRKY126 was performed using the MEME program (http://meme-suite.org/). For malate metabolism and transport genes, candidate gene sequences were obtained from the M. × domestica genome, GDDH13 version 1.1 and the counterpart in Arabidopsis (A. thaliana) were collected from the Arabidopsis Information Resource (http://www.Arabidopsis.org/). Sequence alignment was carried out with DNAMAN version 6.0 software (Lynnon Biosoft, San Ramon, CA, USA) and the phylogenetic tree was constructed following the description above.
RNA extraction and RT-qPCR reactions
Total RNA was extracted using an RNAprep plant kit (Tiangen, Beijing, China) following the manufacturer’s instructions. PrimeScript RT Reagent Kit and gDNA Eraser (TaKaRa, Dalian, China) were used to convert RNA into sscDNA. RT-qPCR was performed according to a previous description (Zhang et al., 2021). Three independent biological replicates containing three technical replicates each were tested and significant difference was analyzed via one-way ANOVA (P < 0.05) or independent t tests (*P < 0.05; **P < 0.01) using IBM SPSS Statistics version 21. The primer sequences designed are shown in Supplemental Table S1.
Subcellular localization
The complete coding sequences of MdWRKY126 and MdMDH5 without stop codon were cloned into the PacI and AscI sites downstream of GFP in the pMDC83-GFP vector, forming the fusion expression vectors 35Spro::MdWRKY126::GFP and 35Spro::MdMDH5::GFP. Subsequently the two fusions were transformed into Arabidopsis leaf protoplasts for 24 h using methods of previous studies (Yoo et al., 2007). Fluorescence was observed under a confocal laser scanning microscope (LSM 710; Carl Zeiss) equipped with a water immersion objective (100×/1.40). GFP excitation was performed using a 488 nm solid-state laser, fluorescence was detected at 498–535 nm, and the intensity and gain were 11.9% and 800, respectively. 4′,6-Diamidino-2-phenylindole (DAPI) excitation was performed using a 405 nm solid-state laser, and fluorescence was detected at 430–470 nm, and the intensity and gain were 6.61% and 800, respectively. Stacks of 1,024 × 1,024 pixels (pixel size of 0.363 × 0.363 μm) optical section were generated with a Z-interval of 0.5 μm. 0.5 µg/mL DAPI (Solarbio, Beijing, China) was used as a cell nuclear dye. All transient expression assays were repeated at least three times. The primer sequences designed are shown in Supplemental Table S1.
Construction of the plasmid and genetic transformation
To obtain MdWRKY126 and MdMDH5 transgenic apple calli and tomato, the two coding sequences were inserted into pCAMBIA2300 vectors with the CaMV35S promoter, while partial 5′-UTR antisense sequences of MdWRKY126 was introduced into pK7GWIWG2(I), constructing overexpression vectors of MdWRKY126 and MdMDH5 as well as MdWRKY126 antisense inhibition vector. Genetic transformation of apple calli and tomato plants was conducted by the Agrobacterium-mediated method (Sun et al., 2006; Yoo et al., 2007). The primer sequences designed are shown in Supplemental Table S1.
Measuring organic acid content
Malate and citrate were extracted in 75% (v/v) methanol with 400 ppm ribitol as an internal standard. After derivatization with methoxyamine hydrochloride and N-methyl-N-trimethylsilyl-trifluoroacetamide sequentially, malate and citrate content were determined by gas chromatography–mass spectrometry (GC–MS) (Shimadzu Corporation, Tokyo, Japan) as previously described (Li et al., 2018a, 2018b). The data were tested for three biological replicates containing three technical replicates each.
Y1H assay
Y1H experiments were carried out as described by Jia et al. (2021). The promoter of MdMDH5 was inserted into the pABAI vector (MdMDH5pro-pAbAi) and MdWRKY126 was cloned to the pGADT7 vector with an activation domain (MdWRKY126-AD). The vectors MdMDH5pro-pAbAi and MdWRKY126-AD were transformed and co-expressed in yeast cells with the compound containing MdMDH5pro-pAbAi and pGADT7 (AD) vector as control. An aliquot of 300 ng/mL Aureobasidin A (ABA) (Warbio, Nanjing, China) was used in this assay. The primer sequences used are shown in Supplemental Table S1.
Dual-LUC assay
The procedures for the LUC assay were as previously described (An et al., 2018). The complete MdWRKY126 ORF was introduced into the effector vector pGreenII62-SK with the 35S promoter (35Spro::MdWRKY126). Promoter fragments from MdMDH5, MdMDH1, and MdAVP3 were individually cloned into the reporter vector pGreenII0800-LUC (MdMDH5pro::LUC/MdMDH1pro::LUC/MdAVP3pro::LUC). After Agrobacterium-mediated infiltration of the effector and reporter vectors into N. benthamiana leaves for 48–72 h, Renilla and Firefly LUC signals were measured with an Infinite M200 (Tecan, Zürich, Switzerland). The luminescence measurement was conducted using a living imaging apparatus. Each LUC assay was repeated three times and all primer sequences designed are shown in Supplemental Table S1.
GUS analysis
The promoter sequence of MdMDH5 was cloned into the PST1 and EcoR1 sites upstream of the GUS reporter gene in the pCAMBIA0390 vector to generate the GUS reporter plasmid (MdMDH5pro::GUS). The MdWRKY126 ORF was introduced into the pCAMBIA2300 vector to form an effector plasmid (35Spro::MdWRKY126). Four combinations of vectors, namely, MdMDH5pro::GUS and 35Spro::MdWRKY126, MdMDH5pro::GUS and 35Spro, GUS and 35Spro::MdWRKY126, and GUS and 35Spro were simultaneously transformed into apple calli. The detection of GUS activity was as described by An et al. (2018). The infiltration was repeated independently at least three times and the primer sequences used are shown in Supplemental Table S1.
ChIp-qPCR analysis
ChIP assay was carried out following the method of Hu et al. (2019). The recombinant 35Spro::MdWRKY126-GFP was transformed into apple calli with 35Spro::GFP as a control to obtain the transgenic material tagged by GFP, which were used for the ChIP-qPCR analysis. An anti-GFP antibody (Beyotime, Jiangsu, China) was used to show interactions between MdWRKY126 and the associated promoters in vivo. Each ChIP assay was repeated three times. The primer sequences designed are shown in Supplemental Table S1.
Determination of MDH activity
MDH activity was determined according to the procedure of Wang et al. (2016). The MDH reductive activity was assayed at 30°C in a 1-mL mixture that contained 50 mM Tris–HCl (pH 7.8), 2 mM MgCl2, 0.5 mM EDTA, 0.2 mM NADH, 2 mM oxaloacetate (OAA), and 50 µL desalted enzyme extract. The same mixture without OAA served as a control. The MDH oxidative activity was measured in 1 mL assays consisting of 50 mM Tris–HCl (pH 8.9), 2 mM MgCl2, 0.5 mM EDTA, 0.2 mM NAD+, 25 mM malate, and 50 µL desalted enzyme extract. The control lacked malate. For each reaction, the spectrophotometric absorbance was measured at 340 nm at 30 s intervals for 5 min. MDH activities were calculated based on the change of absorbance per unit time. The data were tested for three biological replicates and three technical replicates.
Overexpression and virus-induced silencing of genes in apple fruit
Partial antisense sequences of MdWRKY126 and MdMDH5 were isolated and introduced into the pTRV2 (tobacco rattle virus) vector, while the full-length cDNAs of these two genes were cloned into pCAMBIA2300 vector under the control of the 35S promoter. The resulting vectors were named pTRV2-MdWRKY126, pTRV2-MdMDH5 and pCAMBIA2300-MdWRKY126, and pCAMBIA2300-MdMDH5, respectively. These recombinant vectors were used individually or in combination for transient transformation of apple fruit mediated by Agrobacterium (EHA105) as described previously (Zhu et al., 2021). The injected samples were placed in darkness for 1 day and transferred to natural light for 3 days at 23°C. Then the injected part of fruits was collected and analyzed for gene expression and malate content. One fruit was used as a biological replicate and at least three biological replications were performed.
Statistical analysis
The data were analyzed via one-way ANOVA (P < 0.05) or independent t tests (*P < 0.05; **P < 0.01) using IBM SPSS Statistics version 21. The values are presented as the mean ± standard deviation (SD) of biological triplicates.
Accession numbers
Sequence data from this article can be found in GenBank/GDR data libraries under accession numbers MdWRKY126 (MD11G1059400), MdMDH5 (MD15G1304800), MdAVP3 (MD02G1020700), MdMDH1 (MD13G1214000), PhPH3 (AMR43368.1), and MdActin (MDP0000752428).
Supplemental data
The following materials are available in the online version of this article.
Supplemental Figure S1. The expression of MdWRKY126 within transcriptome data of “BSKP” and “AF” fruit at different developmental stages.
Supplemental Figure S2. Phylogenetic analyses of malate content-related transporters, proton pumps, and MDHs.
Supplemental Figure S3. The mRNA relative expression level of 11 malate-related genes in “Fuji” apple fruit.
Supplemental Figure S4. Screening and validation of MdWRKY126 direct downstream functional candidate genes.
Supplemental Figure S5. LUC assay in apple calli revealed that MdWRKY126 interacted with MdMDH5 promoter.
Supplemental Figure S6. The mRNA relative expression level of MdMDH5 in apple fruit from cultivars “AF” and “BSKP.”
Supplemental Figure S7. The expression of MdPH1 and MdPH5 in control (P2300) and MdWRKY126-overexpressing calli (MdOW-1,2,3).
Supplemental Table S1. Primers used in this study.
M.L., F.M., Y.L.R., and B.M. designed and supervised this research. L.Z., C.W., X.C., and Y.Y. performed this research. M.L., B.M., L.Z., and C.W. analyzed the data. L.Z. wrote the manuscript. M.L., F.M., Y.L.R., B.M., and L.Z. discussed this study and revised the manuscript.
The author responsible for distribution of materials integral to the findings presented in this article in accordance with the policy described in the Instructions for Authors (https://dbpia.nl.go.kr/plphys/pages/General-Instructions) is Mingjun Li ([email protected]).
Acknowledgments
We thank the Horticulture Science Research Center at the College of Horticulture, NWAFU, for their technical support in this work.
Funding
This work was supported by the Program for National Key Research and Development (2018YFD1000200), the National Natural Science Foundation of China (Grant Number 32072527), and the Chinese Universities Scientific Fund (2452020007).
Conflict of interest statement. The authors declare that they have no conflicts of interest.
References
Hulme A, Wooltorton L (1957) The organic acid metabolism of apple fruits: Changes in individual acids during growth on the tree. J Sci Food Agric 8: 117–122
Author notes
Senior author
These authors contributed equally to this work.