-
PDF
- Split View
-
Views
-
Cite
Cite
Hideyuki Takahashi, Masahiro Nishihara, Chiharu Yoshida, Kimiko Itoh, Gentian FLOWERING LOCUS T orthologs regulate phase transitions: floral induction and endodormancy release, Plant Physiology, Volume 188, Issue 4, April 2022, Pages 1887–1899, https://doi.org/10.1093/plphys/kiac007
- Share Icon Share
Abstract
Perennial plants undergo a dormant period in addition to the growth and flowering phases that are commonly observed in annuals and perennials. Consequently, the regulation of these phase transitions in perennials is believed to be complicated. Previous studies have proposed that orthologs of FLOWERING LOCUS T (FT) regulate not only floral initiation but also dormancy. We, therefore, investigated the involvement of FT orthologs (GtFT1 and GtFT2) during the phase transitions of the herbaceous perennial gentian (Gentiana triflora). Analysis of seasonal fluctuations in the expression of these genes revealed that GtFT1 expression increased prior to budbreak and flowering, whereas GtFT2 expression was induced by chilling temperatures with the highest expression occurring when endodormancy was released. The expression of FT-related transcription factors, reportedly involved in flowering, also fluctuated during each phase transition. These results suggested the involvement of GtFT1 in budbreak and floral induction and GtFT2 in dormancy regulation, implying that the two gentian FT orthologs activated a different set of transcription factors. Gentian ft2 mutants generated by CRISPR/Cas9-mediated genome editing had a lower frequency of budbreak and budbreak delay in overwintering buds caused by an incomplete endodormancy release. Our results highlighted that the gentian orthologs of FRUITFULL (GtFUL) and SHORT VEGETATIVE PHASE-like 1 (GtSVP-L1) act downstream of GtFT2, probably to prevent untimely budbreak during ecodormancy. These results suggest that each gentian FT ortholog regulates a different phase transition by having variable responses to endogenous or environmental cues, leading to their ability to induce the expression of distinct downstream genes.
Introduction
Perennial plants can survive for several years by repeating the cycle of growth, flowering, and dormancy phases. By tightly regulating the transition and maintenance of these phases, perennials can grow, develop, and adapt to the different environmental conditions encountered over an entire year. Among the phases, dormancy is one of the most important features of the perennial lifestyle, e.g. bud dormancy occurs in most perennials to withstand harsh environmental conditions.
Bud dormancy is classified into the three distinct phases of paradormancy, endodormancy, and ecodormancy, each phase is affected by growth arrests in distal organs, internal bud signals, and environmental conditions, respectively (Lang, 1987). In Japanese gentians (Gentiana triflora, G. scabra, and their hybrids), overwintering buds (OWBs) are produced from underground tissues prior to winter and subsequently enter endodormancy. During winter, the phase transition from endodormancy to ecodormancy occurs; in the spring, OWBs initiate budbreak (Takahashi et al., 2014). After budbreak, Japanese gentians stay in the vegetative growth phase for several months, followed by a transition to the reproductive growth phase (Imamura et al., 2011).
Importantly, a shift from endodormancy to ecodormancy (endodormancy release) is necessary for budbreak in response to favorable conditions. Previous studies demonstrated that the bud dormancy phases are regulated by daylength, temperature, and phytohormones (Maurya and Bhalerao, 2017; Liu and Sherif, 2019). For example, short days induce growth cessation and bud set in hybrid aspen, whereas extended exposure to chilling induces endodormancy release in Japanese pear flower buds in conjunction with characteristic genetic or metabolic fluctuations (Böhlenius et al., 2006; Horikoshi et al., 2018). Among the phytohormones that play a role in bud dormancy, abscisic acid (ABA) is essential for establishing and maintaining endodormancy (Tylewicz et al., 2018; Singh et al., 2019). Other phytohormones such as gibberellins, auxins, cytokinins, and ethylene also serve as signal molecules regulating the dormancy phase transitions (Liu and Sherif, 2019). Furthermore, sugars are also involved in bud dormancy transitioning. Changes in sugar concentrations affect the expression of cell-cycle genes, and the progression or arrest of the cell cycle appears to be associated with dormancy phases (Horvath et al., 2002; Espinosa-Ruiz et al., 2004). In Japanese gentian OWBs, gentiobiose [β-d-Glcp-(1→6)-d-Glc] functions as a signal for budbreak through redox regulation. The concentration of gentiobiose significantly decreases from the endodormancy to ecodormancy stages, probably to prevent unfavorable budbreak during ecodormancy (Takahashi et al., 2014).
Notably, some orthologs of FLOWERING LOCUS T (FT) in perennial species are reported to be involved in regulating bud dormancy (reviewed in Singh et al., 2017). Generally, FT plays a central role in regulating floral initiation, which refers to the transition from the vegetative growth phase to the reproductive growth phase by integrating signals from multiple pathways to promote flowering (Teotia and Tang, 2015). In the annual Arabidopsis (Arabidopsis thaliana), CONSTANS (CO) expression is regulated by the plant’s circadian clock and daylength, and the accumulated CO protein stimulates the expression of FT (Valverde, 2011). In contrast, temperature-dependent signals affect the expression of FLOWERING LOCUS C (FLC) and SHORT VEGETATIVE PHASE (SVP), thereby suppressing the expression of two floral integrator genes, FT and SUPPRESSOR OF OVEREXPRESSION OF CO1 (SOC1; Helliwell et al., 2006; Lee and Lee, 2010). Other reports have shown that SOC1 expression is positively regulated not only by FT but also by SQUAMOSA PROMOTER BINDING PROTEIN-LIKEs (SPLs) in response to plant age and phytohormones (Lee and Lee, 2010; Yu et al., 2012). Upregulated expression of FT, SOC1, and SPLs combined with the downregulated expression of the floral repressor TERMINAL FLOWER1 (TFL1) and the activation of floral meristem identity genes, including APETALA 1 (AP1), FRUITFULL (FUL), and LEAFY (LFY), initiates flower development (Hanano and Goto, 2011; Teotia and Tang, 2015). Although the molecular basis for floral induction and development centers on FT and is conserved in various perennials, FT also regulates growth cessation, bud set, and dormancy (Maurya and Bhalerao, 2017; Singh et al., 2018). Poplar (Populus spp.) orthologs of FT, PtFT1, and PtFT2 have different functions and are regulated by other environmental cues (Hsu et al., 2011). Upregulated by low temperature, PtFT1 promotes floral initiation but can also prevent short-day-induced dormancy in flowering buds. In contrast, PtFT2 is upregulated by warm temperatures, and long-day photoperiods during vegetative growth inhibit bud set. Furthermore, several reports suggest that FT orthologs are expressed in parallel with cell cycle-, phytohormone-, and sugar-related genes to regulate dormancy phase transitions (Rinne et al., 2011; Vergara et al., 2016; Zhang et al., 2018). Although these observations indicate the possibility that FT orthologs have multiple functions or the orthologs were functionally differentiated to regulate dormancy phase transitions, these hypotheses remain to be fully confirmed.
This study investigated whether FT orthologs have diverse functions during the several phase transitions in the herbaceous perennial Gentiana. Our previous study demonstrated that gentian FT orthologs (GtFT1 and GtFT2) are associated with flowering, i.e. the expression levels of GtFT1 increased prior to floral initiation; high-levels and early expression were observed in early-flowering varieties, and the overexpression of GtFT1 hastened flowering in gentian plantlets and Arabidopsis (Imamura et al., 2011). It was questionable, however, whether GtFT2 mainly functioned as a flowering inducer because the timing for GtFT2 expression did not correspond with the flowering period for field-grown Japanese gentians. Here, we obtained direct evidence that GtFT2 regulates endodormancy release, rather than floral induction, using CRISPR/Cas9 technology. Our results also support the hypothesis that GtFT1 and GtFT2 act with different sets of transcription factors and regulate budbreak, flowering, and dormancy. We propose that FT orthologs in perennials can be functionally differentiated and may be involved individually in different phase transitions during a perennial plant’s life cycle.
Results
Seasonal expression of FT orthologs in field-grown gentians
Gentians have two dormancy phases, i.e. endodormancy (October to November) and ecodormancy (December to March), and two growth phases, i.e. vegetative growth (April to May) and reproductive growth (June or July to September; Imamura et al., 2011; Takahashi et al., 2014). In this study, we characterized the function of gentian FT orthologs during phase transitions other than floral initiation. First, we identified nucleotide sequences of GtFT1 and GtFT2 in G. triflora cv. SpB and obtained sequences that were perfectly matched to those in G. triflora shown in Imamura et al. (2011). Based on these sequences, we measured the seasonal fluctuation of GtFT1 and GtFT2 expression in leaves and shoot apical meristems (SAMs) of field-grown gentians (G. triflora cv. SpB) throughout 1 year (Figure 1A). The relative expression level of GtFT1 was low during dormancy but began to increase in leaves before the onset of budbreak (Figure 1A, inset) and continued to increase until flowering. The transcript abundance of GtFT1 in SAMs was lower than that of leaves, although a slight increase was observed from April to September. In contrast, GtFT2 in leaves and SAMs was expressed at its highest levels in December when the dormancy phase in OWBs transits from endodormancy to ecodormancy, with another slight increase in August. Therefore, it appeared that GtFT1 expression was associated with budbreak and flowering, but GtFT2 expression was associated with endodormancy release. Given that the highest expression of GtFT2 was observed during endodormancy release, we also investigated the expression of GtFT2 and GsFT2 using the OWBs of G. triflora cv. Iwate and G. scabra cv. Alta, which are early-flowering and late-flowering varieties, respectively (Figure 1B). These varieties showed a similar expression pattern to that observed in G. triflora cv. SpB, suggesting that the observed GtFT2 expression during dormancy appeared to be a common feature of gentians.
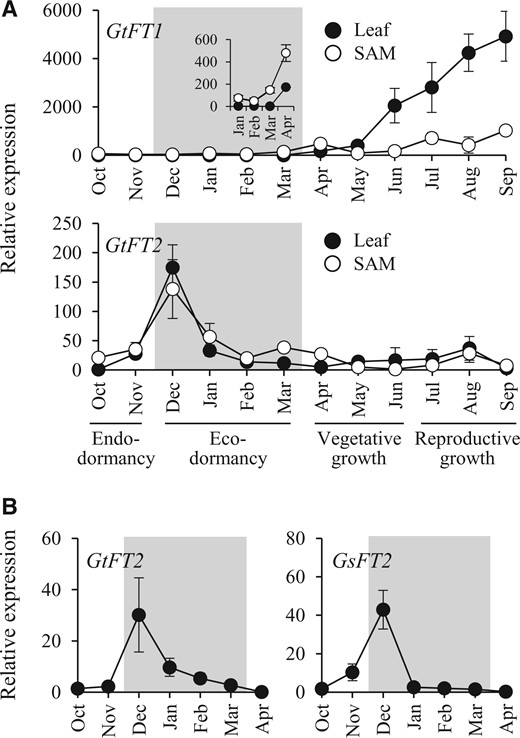
Expression of FT genes in field-grown gentian plants throughout all stages of dormancy and growth. A, Seasonal expression of GtFT1 and GtFT2 in leaves (filled circles) and SAM (open circles) in G. triflora cv. SpB. The inset shows an amplified plot of the expression from January to April. B, Expression of FT2 genes in OWBs of G. triflora cv. Iwate (GtFT2; left) and G. scabra cv. Alta (GsFT2; right). The ecodormancy period is represented by a shaded area. The relative expression level of each gene is normalized to that of Actin, with expression in leaves in October defined as 1. Each value represents the means ± sd calculated from five independent biological replicates. Five biological replicates were prepared each being a single tissue (SAM or leaf) from a plant.
Furthermore, we found that the expression of GtFT1 and GtFT2 had different responses to being chilled (Figure 2). We previously reported that chilling induces endodormancy release, i.e. gentian OWBs enter endodormancy after zero to 3 weeks incubation at 4°C but enter ecodormancy 5 weeks after incubation at 4°C (Takahashi et al., 2014). In the OWBs of G. triflora cv. SpB, the expression level of GtFT1 was unchanged during chilling, whereas the expression level of GtFT2 increased in response to chilling for 3 weeks but decreased 5 weeks after chilling when the dormancy phase transitioned to ecodormancy. These results indicated that GtFT2 had the highest expression level prior to endodormancy release.
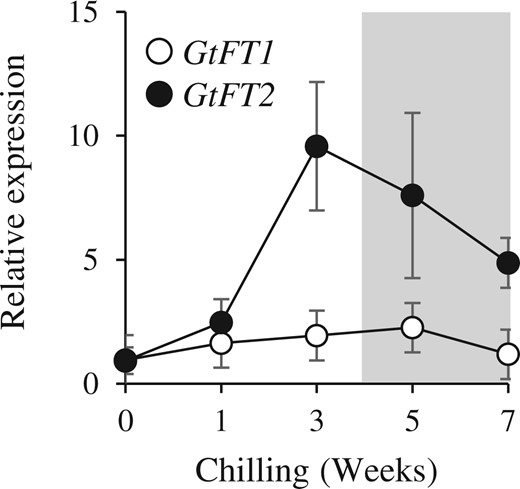
Expression of GtFT1 and GtFT2 in chilled OWBs. Field-grown OWBs of cv. SpB were harvested in October and chilled at 4°C for zero to 7 weeks. The shaded area represents the ecodormancy phase shown by Takahashi et al. (2014). The relative expression level is normalized to Actin and expression for week zero was defined as 1. Each value represents the mean ± sd calculated from three independent biological replicates. Three biological replicates were prepared each being a single OWB tissue from a plant.
Generation of ft2 mutants in gentians using the CRISPR/Cas9 system
To confirm the role of gentian FT2 during dormancy, we produced gentian ft2 mutants in cv. Albireo by CRISPR/Cas9-mediated genome editing (Figure 3). Since cv. Albireo is a hybrid cultivar and possesses two alleles derived from G. triflora and G. scabra, namely Alb-GtFT2 and Alb-GsFT2, a 20-bp sequence in the first exon of FT2 that is identical in both alleles was selected as a target sequence (Figure 3A; Supplemental Figure S1). We obtained 53 transgenic plantlets and confirmed that the mutations were generated at the target site. After sequencing the genomic region and cDNA corresponding to the FT2 locus, we selected two frame-shift mutants, denoted as ft2#35 and ft2#53. The ft2#35 mutant had 7- and 5-nucleotide (nt) deletions in the Alb-Gtft2del129–135 and Alb-Gsft2del130–134 alleles, respectively. The ft2#53 mutant had 4- and 5-nt deletions in Alb-Gtft2del131–134 and Alb-Gsft2del130–135 alleles, respectively. Since these mutations generated premature stop codons predicted to result in truncated FT2 proteins (Figure 3B), we used these mutants in further analyses. First, we investigated whether the ft2 mutations influence plantlet phenotypes and found that the ft2 mutants had similar morphologies and growth characteristics as the wild-type (WT; Supplemental Figure S2A). We also compared soil-grown WT and ft2 mutant plants but found almost no difference in their shoot growth and developmental characteristics; e.g. the timing of floral initiation was normal in the mutants (Supplemental Figure S2B).

CRISPR/Cas9-mediated mutations in the gentian FT2 gene in a hybrid gentian, G. triflora (Gt) × G. scabra (Gs). A, Nucleotide sequences derived from GtFT2 and GsFT2 alleles of the WT and mutants with small deletions in the FT2 gene (ft2#35 and ft2#53). The sgRNA target sequences are shown as white text on a black background and protospacer adjacent motif sequences are highlighted in gray. Deletions are indicated by dashes and the numbers in parentheses on the right side indicate the size of the deletions. B, Deduced amino acid sequences of the WT and ft2 mutants. Asterisks indicate the position of newly generated stop codons.
Mutations in FT2 affect budbreak but do not affect OWB formation
We also compared the morphology of OWBs and the rhizospheres of the WT and ft2 mutants (Figure 4A). The appearance of OWBs and rhizospheres was nearly identical, and there was no significant difference in the number of OWBs per plant (13.7 ± 1.5 in the WT, 13.3 ± 2.5 in ft2#35, and 12.7 ± 3.8 in ft2#53). The OWBs of the WT and ft2 mutants were chilled at 4°C for 7 weeks before being transferred to an optimal growing temperature of 20°C to induce budbreak. Three weeks after transfer, the OWBs of the WT had already sprouted and shoots began to grow, but those of ft2 mutants had just started budbreak (Figure 4B). Five weeks after the transfer, there was a noticeable difference in shoot growth between the WT and the ft2 mutants (Figure 4C). Furthermore, we compared the budbreak frequency and shoot length between WT and ft2 mutants. Although approximately 60% of the OWBs sprouted in the WT by 3 weeks after transfer, only 24% and 30% of the OWBs sprouted in the ft2#35 and ft2#53 mutants, respectively (Figure 4D). Five weeks after transfer, the budbreak frequency increased slightly in the WT and the ft2 mutants compared to 3 weeks after transfer, but the level in the ft2 mutants was significantly lower than that of the WT (Figure 4E). The budbreak frequency did not increase any further, suggesting that the ft2 mutation affected the timing of budbreak and also influenced the budbreak frequency of the OWBs. A comparison of shoot growth after budbreak revealed that the shoot length of the ft2#35 mutant tended to be shorter than the WT, whereas shoot length in the ft2#53 mutant was significantly shorter than the WT (Figure 4F).
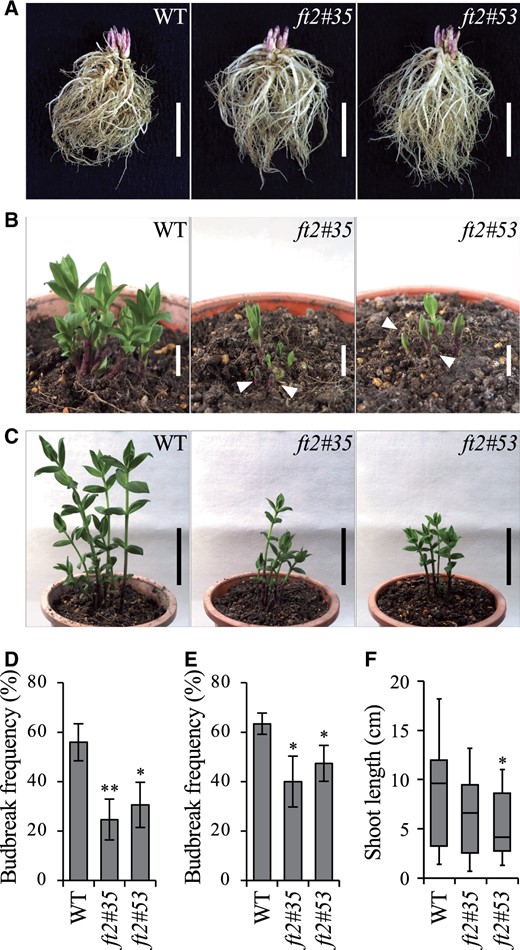
Suppression of budbreak and growth in OWBs by the ft2 mutation. After a chilling treatment for 7 weeks, OWBs were transferred to an optimal growth temperature (20°C) and budbreak and growth were measured. A, Morphology of OWBs and underground organs in the WT and ft2 mutants (ft2#35 and ft2#53). Scale bars indicate 5 cm. B, Budbreak of OWBs occurred 3 weeks after transfer. Arrowheads indicate unsprouted buds. Scale bars indicate 1 cm. C, Shoots of WT and ft2 mutants at 5 weeks after transfer. Scale bars indicate 5 cm. D, Budbreak frequency of WT (n = 41), ft2#35 (n = 40), and ft2#53 (n = 38) at 3 weeks after transfer. E, Budbreak frequency of WT (n = 41), ft2#35 (n = 40), and ft2#53 (n = 38) at 5 weeks after transfer. Each value represents the mean ± sd. F, Box and whisker plot of shoot length in the WT (n = 26), ft2#35 (n = 16), and ft2#53 (n = 18) at 5 weeks after transfer. The top and bottom of the box represent the upper and lower quartile, respectively, and lines inside box represent the median. The whiskers represent the highest and lowest values. Significant differences from WT are shown (*P < 0.05, **P < 0.01, Student’s t test).
The dormancy phase transition in gentian OWBs is affected by FT2
Because endodormancy release is necessary for budbreak, we hypothesized that the FT2 mutation suppressed the release, resulting in the delay and suppression of budbreak. In gentian OWBs, the gentiobiose concentration decreased significantly after endodormancy release, suggesting that gentiobiose can be used as a marker to distinguish between the endodormancy and ecodormancy phases (Takahashi et al., 2014). To investigate the effect of ft2 mutations on endodormancy release, we measured the gentiobiose concentrations in OWBs of the WT and ft2 mutants during endo- and ecodormancy (Figure 5). During endodormancy, no significant difference was observed in the gentiobiose concentration between the WT and ft2 mutants. Five weeks after chilling, the dormancy phase had transitioned from endodormancy to ecodormancy, and the gentiobiose concentration decreased by >70% in WT plants. In the ft2 mutants, the decrease in the gentiobiose concentration from 3 to 5 weeks after chilling was negligible, suggesting that endodormancy release was incomplete in the OWBs of ft2 mutants. We also compared the sucrose concentration in the WT and ft2 mutants because sucrose accumulation during chilling treatments has been reported in other plant species (Tabaei-Aghdaei et al., 2003). The sucrose concentration tended to increase with chilling, but there were no significant differences in sucrose content between WT plants and ft2 mutants. The one exception was that a lower sucrose concentration was observed in the ft2#53 mutant after chilling for 7 weeks, suggesting that chilling sensitivity was unchanged in this mutant.
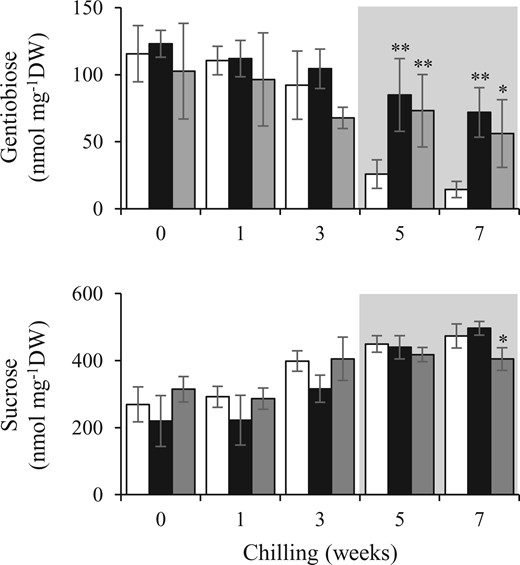
Effect of FT2 impairment on gentiobiose and sucrose concentrations in gentian OWBs during endo- and ecodormancy. The concentrations in the WT (white bars), ft2#35 (black bars), and ft2#53 (gray bars) were compared. The predicted ecodormancy phase is represented by the shaded area. Each value represents the mean ± sd calculated from five independent biological replicates. Five biological replicates were prepared each being a single OWB tissue from a plant. Significant differences from the WT are shown (*P < 0.05, **P < 0.01, Student’s t test).
The ft2 mutation affects the expression of flowering-related genes in gentian OWBs
We found that the frame-shift mutation in FT2 was caused by CRISPR/Cas9, resulting in incomplete endodormancy release and suppressed budbreak; however, the mechanism by which FT2 regulates this process remained unclear. Pérez et al. (2011) previously demonstrated that flowering-related genes in grapevine (Vitis vinifera) buds helped regulate the induction and maintenance of endodormancy with VvFT. We, therefore, investigated the expression of gentian orthologs of genes reported to be involved in flowering regulation. A search of a gentian EST library produced the candidate orthologs, GtFLC-L, GtSOC1a, GtSOC1b, GtFUL, GtSPL4, GtAP1, and GtLFY (Figure 6A). Alignments of the deduced amino acid sequences of the seven orthologs are shown in Supplemental Figure S3. Sequence information for GtSVP-L1 was provided from a previous study (Yamagishi et al., 2016). First, we determined the expression levels of flowering-related genes in the OWBs of the WT and ft2 mutants treated at 4°C for 3 or 7 weeks; for the WT, the dormancy phases at 3 and 7 weeks corresponded to endodormancy or ecodormancy, respectively (Figure 6B). Fluctuations in gene expression occurred when the dormancy phase changed; however, there were no significant difference in the expression of GtFLC-L, GtSOC1a, GtSPL4, GtAP1, and GtLFY between the WT and ft2 mutants. Although the expression level of GtSOC1b in endodormant OWBs was similar to that in ecodormant OWBs in all lines, one of the ft2 mutants had a lower expression level of GtSOC1b compared to the WT. Importantly, GtFUL and GtSVP-L1 expression levels increased during the transition from endodormancy to ecodormancy in the WT, but the increase in expression was suppressed significantly in the ft2 mutants.
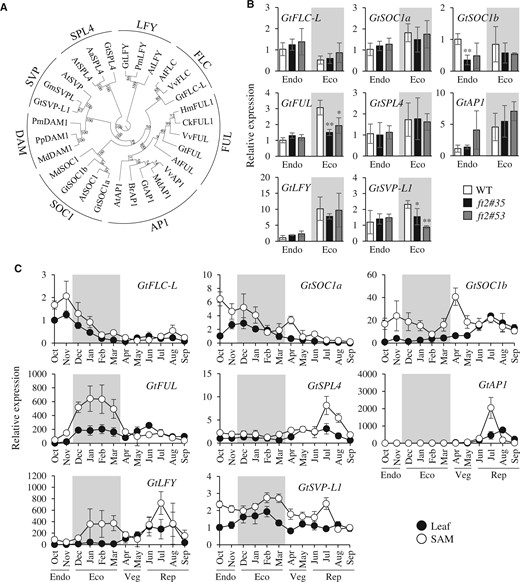
The ft2 mutation affects the expression of flowering-related genes in gentians. A, A phylogenetic tree based on the deduced amino acid sequences of gentian orthologs of FT-related genes denoted as GtFLC-L, GtSOC1a, GtSOC1b, GtSVP-L1, GtFUL, GtSPL4, GtAP1, and GtLFY and their orthologs from Arabidopsis thaliana (AtFLC, Q9S7Q7; AtSOC1, O64645; AtSVP, Q9FVC1; AtFUL, Q38876; AtSPL4, Q9S7A9; AtAP1, P35631; AtLFY, Q00958), Arabis alpina (AaSPL4, S4WUK1), Brassica rapa (BrAP1, P0DI14), Cornus kousa (CkFUL1, M4IT41), Glycine max (GmSVPL, E3NYP9), Hydrangea macrophylla (HmFUL1, B5BUX3), Malus domestica (MdAP1, Q6YNE5; MdSOC1, Q09JE1; MdDAM1, A0A1B2LP93), Prunus mume (PmLFY, A0A140FAH6; PmDAM1, A0A2H4N2C0), Pyrus pyrifolia (PpDAM1, A0A0D4ZYJ8), and Vitis vinifera (VvFLC, D1MDP4; VvFUL, D7SMN6; VvAP1, Q6E6S7). Bootstrap values above the nodes were obtained from 1,000 replicates. B, A comparison of expression levels of flowering-related genes in gentian OWBs of the WT (white bars), ft2#35 (black bars), and ft2#53 (gray bars) during endodormancy and ecodormancy. The endodormant WT expression data were normalized to 1. C, Seasonal expression of flowering-related genes in leaves (solid circles) and SAMs (open circles) of gentians, covering endodormancy (Endo), ecodormancy (Eco), the vegetative growth phase (Veg), and the reproductive growth phase (Rep). The leaf expression data for October were normalized to 1. The predicted ecodormancy phase is represented by the shaded area. In (B) and (C), each value represents the mean ± sd calculated from five independent biological replicates. Five biological replicates were prepared each being a single tissue (OWB, leaf or SAM) from a plant. Significant differences from the WT are shown (*P < 0.05, **P < 0.01, Student’s t test).
Seasonal expression of the gentian orthologs of flowering-related genes was also investigated in cv. SpB (Figure 6C). GtFLC-L expression in leaves and SAMs decreased from November to February and remained low after budbreak. The transcript level of GtSOC1a in leaves and SAMs tended to decrease from October to September, except for a transient increase in SAMs observed in April. The expression of GtSOC1b in leaves increased gradually, with the highest expression levels observed in July and a transient increase observed in SAMs in April. Interestingly, the expression levels of GtFUL increased 3.5- and 8.9-fold in leaves and SAMs, respectively, during the transition from endodormancy (November) to ecodormancy (December). In contrast, the expression level of GtFUL decreased when budbreak occurred in April. In leaves, GtFUL expression increased again in June but decreased in July. The transcript abundance of GtSPL4 and GtAP1 showed a similar trend, with expression levels in SAMs being low from endodormancy to the vegetative growth phase before increasing transiently in July when reproductive growth began. GtSPL4 expression in leaves increased slightly towards the onset of the flowering period, whereas GtAP1 expression increased transiently in August. Transcript abundance of GtLFY in SAMs increased during ecodormancy before decreasing in April and increasing again in June. In contrast, GtLFY expression in leaves was low during dormancy, before increasing gradually from March to August and then decreasing in September. The expression of GtSVP-L1 in SAMs mirrored the expression of GtLFY in SAMs; however, the expression of GtSVP-L1 in leaves was relatively higher during ecodormancy compared to that during other growth phases.
Mutation of FUL inhibits dormancy of gentian OWBs
Since the expression of GtFUL increased during ecodormancy and ft2 mutations significantly affected GtFUL expression, we generated ful mutants by CRISPR/Cas9 technology to examine the relationship between GtFUL and ecodormancy (Figure 7). We constructed a binary CRISPR/Cas9 vector containing tandem single-guide RNAs (sgRNAs) that targeted the first exon (target sequence 1) and the second exon (target sequence 2) of FUL, respectively (Supplemental Figure S4A). We obtained 27 transgenic plants of G. triflora x G. scabra cv. Albireo and selected two frame-shift mutants (ful#25 and ful#27; Figure 7A; Supplemental Figure S4, B and C). DNA sequence analysis revealed that ful#25 had 14- and 10-nt deletions in target sequence 1 of the Alb-Gtfuldel35–48 and Alb-Gsfuldel41–50 alleles, respectively, and 1-nt insertion and 3-nt deletions in target sequence 2 of the Alb-Gtfulin235 and Alb-Gsfuldel231–233 alleles, respectively. The ful#27 mutant had 4- and 14-nt deletions in target sequence 1 of the Alb-Gtfuldel42–45 and Alb-Gsfuldel32–45 alleles, respectively, and 2- and 4-nt deletions in target sequence 2 of the Alb-Gtfuldel230–231 and Alb-Gsfuldel230–233 alleles, respectively. These mutations generated premature stop codons that were predicted to result in truncated GtFUL and GsFUL proteins (Supplemental Figure S4B). We found that vegetative growth was inhibited in both ful mutants; in particular, stem growth and flowering were strongly inhibited (Figure 7B). Furthermore, a comparison of OWB appearance revealed that the number and size of OWBs were lower in the ful mutants than in the WT (Figure 7C). Most importantly, the OWBs of the ful mutants had already sprouted when the OWBs of the WT were still in the endodormancy phase. These results suggested that GtFUL had multiple functions in flowering and dormancy, although the detailed mechanisms underlying these are currently unknown (Figure 8).
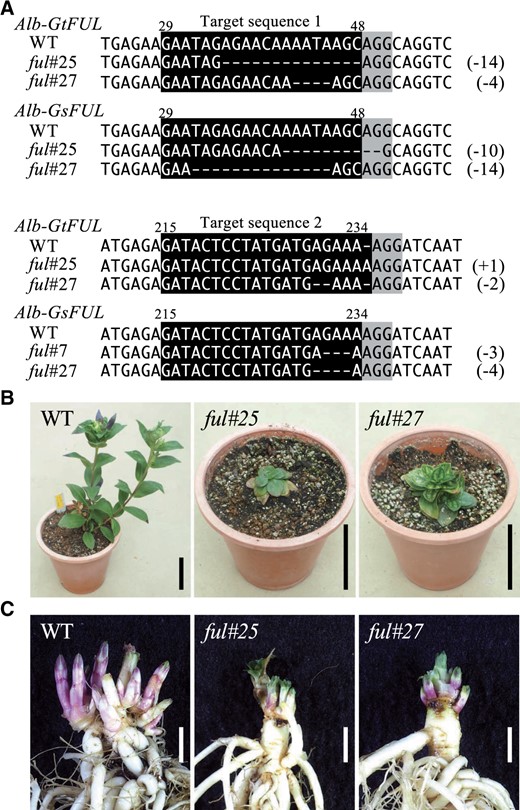
CRISPR/Cas9-mediated genome editing of FUL in gentian plants. A, The DNA sequence of FUL in WT, ful#25, and ful#27 plantlets. The sgRNA target sequences are shown as white text on a black background and protospacer adjacent motif sequences are highlighted in gray. Deletions are indicated by dashes and the numbers in parentheses on the right side indicate the size of the deletions. B, Photographs of 4-month-old WT, ful#25, and ful#27 plantlets. Scale bars indicate 5 cm. C, Comparison of phenotypes of OWBs of WT, ful#25, and ful#27. Scale bars indicate 1 cm.
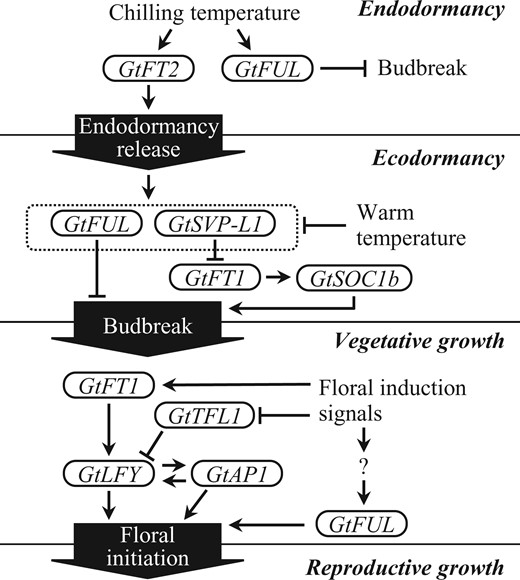
A schematic model for genetic interactions of gentian FT orthologs and related genes that regulate developmental phase transitions in gentians.
Discussion
Possible functional differentiation of gentian FT orthologs
Previously, we showed that the timing of GtFT1 expression matched the flowering time of field-grown gentian plants (Imamura et al., 2011). That is, GtFT1 expression began to increase approximately 1 month before floral initiation, and the overexpression of GtFT1 in gentian plantlets or Arabidopsis induced early flowering. These results indicated that GtFT1 might act as a flowering promoter; however, we demonstrated here that the increase in GtFT1 expression started prior to budbreak in OWBs. The relationship between FT orthologs and budbreak has been reported in several perennial species (Kotoda et al., 2010; Niu et al., 2015; Singh et al., 2018). Additionally, Varkonyi-Gasic et al. (2013) revealed that FT expression began to increase before budbreak in kiwifruit, suggesting that the increased expression of FT promotes budbreak and subsequent flowering. This observation is noteworthy since Japanese gentian species are in the vegetative growth phase for over 2 months after budbreak, and budbreak can be clearly distinguished from flowering. GtFT1 expression in gentian increased in two stages: one stage occurred prior to budbreak and the other stage was a further increase prior to floral initiation. Therefore, GtFT1 possibly is multifunctional in inducing budbreak and floral initiation.
In contrast to GtFT1, the highest expression of GtFT2 was observed when endodormancy release occurred. There was no effect of daylength on GtFT1 and GtFT2 expressions, and it is also not known as to which environmental factors affect the expression of GtFT1 and GtFT2 (Imamura et al., 2011). We found here that chilled OWBs induced GtFT2 expression, but did not affect GtFT1 expression. The difference in inducing factors between GtFT1 and GtFT2 agrees with a previous finding (Hsu et al., 2011). Chilling-inducible FT orthologs involved in endodormancy release have been identified in perennials, such as kiwifruit (Actinidia spp.) and poplar (Rinne et al., 2011; Varkonyi-Gasic et al., 2013; Singh et al., 2018). In perennials, DORMANCY-ASSOCIATED MADS-BOX (DAM) is well studied among the FT-related genes that regulate bud dormancy (Yamane et al., 2021). Since DAM genes are upregulated during dormancy induction and maintenance and then negatively regulate the expression of FT orthologs, DAMs are one of the main factors responsible for regulating dormancy in several perennial species (Falavigna et al., 2019). We did not find any DAM orthologs from the gentian expressed sequence tag (EST) library that was constructed by using multiple organs from various developmental stages. The possibility remains that the orthologs were expressed in other periods or organs that were not used for EST library construction. Although further investigations are still needed, it is possible that chilling-induced suppression of the expression of DAM orthologs or genes with similar function is involved in the upregulation of GtFT2.
Importantly, we previously revealed that the overexpression of GtFT2 induced early flowering in gentian and Arabidopsis (Imamura et al., 2011), whereas GtFT2 is also apparently involved in endodormancy release as observed in this study. These results indicate that gentian FT paralogs are capable of inducing flowering when host plants are in the vegetative growth phase; however, based on differences in the timing and location of their expression, the FT paralogs seem to have a variety of functions. In addition, we found candidate genes that may play roles in phase transitions under the control of GtFT1, i.e. GtSOC1b was expressed in SAMs prior to budbreak, but GtLFY and GtAP1 were expressed in SAMs prior to floral initiation (Figure 6). Many reports have demonstrated that FT activates floral meristem identity genes such as LFY and AP1 as well as the MADS transcription factor SOC1 to promote flowering (reviewed in Teotia and Tang, 2015). This finding implies that GtFT1 activates different transcription factors depending on the season. Although further studies are necessary to confirm these regulatory processes, our results highlight the possibility that GtFT1 activates phase-specific sets of transcription factors to regulate the phase transitions suitable for the environment.
Gentian FT2 accelerates endodormancy release and affects ecodormancy by controlling GtFUL and GtSVP-L1 expression
In this study, we produced two ft2 mutants (ft2#35 and ft2#53) using the CRISPR/Cas9 system optimized for gentian (Tasaki et al., 2019) to obtain direct evidence that GtFT2 affects endodormancy release. The mutation was predicted to lead to a truncated GtFT2 protein lacking approximately 130 amino acids in an essential region for evaluating FT function (Abe et al., 2005; Hanzawa et al., 2005). Consequently, the function of the GtFT2 gene in these mutants was expected to be disrupted. We found that the ft2 mutants showed no differences in growth or flowering time compared to the WT, indicating that the primary function of FT2 is not floral induction, although it may play a supplementary role. Furthermore, since there were no differences in the appearance and number of OWBs or in the growth of underground organs of the ft2 mutants compared to the WT, it appeared that FT2 functions mainly after the initiation of endodormancy. Our results showed that the ft2 mutations impaired budbreak in OWBs. Budbreak occurrence in the ft2 mutants was lower than that of WT plants at both 3 and 5 weeks after being transferred to optimal growth temperatures. These findings indicate that some of the OWBs in ft2 mutants lost the ability to sprout, and, even among those that did sprout, budbreak was delayed. Consequently, we hypothesize that an abnormal dormancy transition occurred in the OWBs of the ft2 mutants. In gentian OWBs, dormancy phases are easily distinguished because the gentiobiose concentration decreases markedly after endodormancy release (Takahashi et al., 2014). Despite the apparent decrease in gentiobiose concentration in the OWBs of WT plants, our experiments documented that the decrease was significantly suppressed in the OWBs of the ft2 mutants. This result suggests that endodormancy release was not completed successfully in the ft2 mutants. Our results also showed that the gentiobiose concentration decreased gradually in the chilled ft2 mutants, implying that the process of endodormancy release was not arrested but proceeded slowly. These results collectively indicate that GtFT2 functions as an accelerator for endodormancy release in gentian OWBs, and that the delay in endodormancy release due to the GtFT2 mutation resulted in delayed budbreak.
We also found that the ft2 mutations repressed the transcript abundance of GtFUL and GtSVP-L1, indicating that endodormancy release is necessary to increase the expression levels of GtFUL and GtSVP-L1. Seasonal expression analysis in field-grown gentian plants revealed that the expression levels of GtFUL and GtSVP-L1 increased during endodormancy and were further elevated after endodormancy release but decreased concurrently with the onset of budbreak. Additionally, OWBs of the ful mutants exhibited budbreak even though the dormancy phase did not change to ecodormancy. To confirm the role of GtFUL expression, we treated the WT OWBs with chilling and found that the expression of GtFUL increased and was maintained at a certain level during endodormancy (Supplemental Figure S5), implying that GtFUL suppressed budbreak during both endodormancy and ecodormancy phases and that the lack of GtFUL leads to extremely early budbreak. With regard to GtSVP-L1, previous study revealed that the SVP ortholog, SVP-like (SVL), acts as dormancy promoter and that decreased expression of SVL induced early budbreak in hybrid aspen (Singh et al., 2018). On the basis of these findings, we speculated that the expression of GtFUL and GtSVP-L1 was increased to prevent untimely budbreak during dormancy, and decreased when receiving budbreak signals such as warm temperatures (Figure 8). This hypothesis is supported by studies showing that FUL and SVP also regulate growth arrest and dormancy (Balanzà et al., 2018; Singh et al., 2018). Although FUL is known to be involved in several developmental processes, including flowering and fruit development (Gu et al., 1998; Melzer et al., 2008), FUL suppresses the expression of SMALL AUXIN UPREGULATED RNA 10 (SAUR10), a member of the SAUR family of genes that regulates plant growth through auxin, and promoted the proliferative arrest of the inflorescence meristem to terminate indeterminate flower production in Arabidopsis (Bemer et al., 2017; Balanzà et al., 2018). In contrast, SVP promotes dormancy in hybrid aspen buds under ABA-mediated photoperiodic control, and the overexpression of SVP2 prevents budbreak in kiwifruit (Wu et al., 2017; Singh et al., 2019). Notably, the regulation of budbreak by SVP orthologs is accomplished through FT orthologs. Singh et al. (2018) demonstrated that the overexpression of an SVL gene repressed budbreak in hybrid aspen through the repression of FT expression, whereas RNAi-mediated suppression of SVL upregulated FT expression, resulting in early budbreak. Since GtFT1 and GtSVP-L1 showed the opposite expression pattern before budbreak in our study, it is possible that GtSVP-L1 repressed GtFT1 expression, and then budbreak was induced. Additionally, because SVP represses gibberellin synthesis during Arabidopsis flowering (Andrés et al., 2014), the negative regulation of gibberellins by SVP and SVL may also contribute to dormancy maintenance in perennial buds. In addition to reports that FUL and SVP are involved in FT and phytohormone regulation, the overexpression of CsFUL downregulated cell division and cell cycle-related genes in cucumber (Zhao et al., 2019). SVL also affects the cell cycle by regulating EARLY BUD BREAK 1 and CYCLIND3.1 genes in hybrid aspen (Azeez et al., 2021). The cell cycle of dormant buds is typically arrested at the G1 phase, and progression from the G1 stage to the S stage is promoted by gibberellin and CYCLIND3.1 during budbreak (Horvath et al., 2002; Azeez et al., 2021). It is, therefore, possible that GtFUL and GtSVP-L1 repress budbreak through sequential and/or simultaneous regulation of GtFT1, the cell cycle, and phytohormones.
Conclusion
In this study, we documented that two gentian FT orthologs function at different developmental phases: namely, GtFT1 functions at the beginning and end of the vegetative growth phase, whereas GtFT2 functions during the endodormancy phase (Figure 8). Our study supports the notion that GtFT1 promotes budbreak and floral initiation in concert with other transcription factors. Analysis of ft2 mutants created by CRISPR/Cas9-mediated genome editing revealed that mutations in GtFT2 suppressed the transition to the dormancy phase, resulting in a lower budbreak frequency and budbreak delay. This finding indicated that GtFT2 functions as an accelerator of endodormancy release. The GtFT2 mutation also suppressed the expression of GtFUL and GtSVP-L1, two genes considered to repress untimely budbreak during ecodormancy. Our results also highlight the idea that gentian bud dormancy is regulated by signal transmission from one FT ortholog to another FT ortholog through transcription factors. Furthermore, duplicated FT paralogs in gentians may be differentiated by their responsiveness to endogenous and environmental cues and their ability to regulate downstream genes required to set the developmental phases suitable for growth or survival.
Materials and methods
Plant materials
Three-year-old Gentiana triflora cv. SpB, Iwate, and G. scabra cv. Alta plants grown in an agricultural field in Iwate Prefecture were used to study gene expression in gentians. For seasonal expression analysis of G. triflora cv. SpB, OWBs were harvested every month from October 2011 to March 2012, and leaves and SAMs of G. triflora cv. SpB were harvested every month from April 2011 to September 2012. Five OWBs, leaves, and SAMs were harvested from five different field-grown plants. The leaves and SAMs of the harvested OWBs were separated before analysis. OWBs of G. triflora cv. Iwate and G. scabra cv. Alta were harvested from field-grown plants every month from October 2010 to April 2011. For the chilling treatment, OWBs of cv. SpB harvested in October 2011 were transferred to 4°C and sampled at 0, 1, 3, 5, and 7 weeks after initiating the treatment. All field samples were harvested at 10:00 to 10:30 a.m. The samples were frozen in liquid nitrogen, lyophilized using a freeze dryer (FDU-2210, EYELA), and stored at −20°C until use. For genome editing, G. triflora x G. scabra cv. Albireo was used. Plantlets were grown on solid Murashige and Skoog (MS) medium containing 0.2% (w/v) gellan gum at 20 °C with a 16-h/8-h light/dark photoperiod at a light intensity of 40 µmol m−2 s−1.
Vector construction and plant transformation
The ft2 and ful mutants were generated by CRISPR/Cas9-mediated genome editing using the gentian cultivar reported by Tasaki et al. (2019). Binary CRISPR/Cas9 vectors were constructed based on the pSbar-pcoCas9 vector with a plant codon-optimized Cas9, SpCas9 (pcoCas9), and a gene for bialaphos-resistance. A sgRNA expression cassette containing a 20-bp nt sequence targeting the FT2 and FUL genes was introduced into pSbar-pcoCas9 as a BstXI–AscI fragment to produce pSbar-pcoCas9-AtU6-26p-FT2-gRNA and pSbar-pcoCas9-AtU6-26p-FUL-gRNA vectors (Supplemental Figure S6) that were then transformed into Agrobacterium tumefaciens strain EHA101 by electroporation. Plant transformation was achieved using the leaf disc method optimized for gentian species, as reported by Mishiba et al. (2005). Briefly, leaf sections excised from gentian plantlets (G. triflora x G. scabra cv. Albireo) were co-cultivated for 5 d with A. tumefaciens carrying a binary CRISPR/Cas9 vector. After washing, leaf sections were placed on half-strength solid MS medium containing 0.5 mg L−1 bialaphos, 500 mg L−1 cefotaxime, 10 mg L−1 thidiazuron, and 1 mg L−1 1-naphthaleneacetic acid. Adventitious shoots induced from calli dedifferentiated from leaf sections were transferred to half-strength solid MS medium containing 0.75 mg L−1 bialaphos, 500 mg L−1 cefotaxime, and 3% (w/v) sucrose for rooting. Transgenic plantlet selections were identified by polymerase chain reaction (PCR) using MightyAmp DNA polymerase (Takara) with specific primers for pcoCas9 (Supplemental Table S1) according to the manufacturer’s instructions.
Mutation detection
Genomic DNA was extracted from the leaf tissues of transgenic plantlets using an innuPREP Plant DNA Kit (Analytik Jena) according to the manufacturer’s instructions and used as a template. The genomic sequence, including the target sequence, was amplified using PrimeSTAR Max DNA Polymerase (Takara Bio) and the primers shown in Supplemental Table S1. The purified PCR product was cloned using a ZeroBlunt TOPO PCR Cloning Kit (Thermo Fisher Scientific). DNA was sequenced using an ABI PRISM Big Dye Terminator Cycle Sequencing Kit with an ABI 3100 DNA sequencer (Applied Biosystems). A UPGMA dendrogram was generated using CLC Sequence Viewer 8.0 software (CLC Bio). Nucleotides and deduced amino acid sequences were aligned using Genetyx-Mac ver. 19 software (Genetyx Corporation).
Quantification of sugar concentrations
Sugar extraction was performed according to the method of Takahashi et al. (2014). Briefly, lyophilized tissue samples were pulverized in a Micro Smash M100 (TOMY) cell disruptor and homogenized with ice-cold 50% (v/v) methanol containing 10 μM 1,4-piperazineethanesulfonate and methionine sulfone as internal standards for quantification. Homogenates were then centrifuged at 20,000g for 5 min, the supernatant liquid was filtered through a 3-kDa-cutoff filter (Millipore) by centrifuging at 16,000g for 60 min, and the filtrates were used for analysis. Sugars were quantified by liquid chromatography/quadrupole time‐of‐flight mass spectrometry (Agilent Technologies) with a Hypercarb column (4.6 × 100 mm, 5 µm; Thermo Scientific; Takahashi et al., 2017). Accuracy was verified with known concentrations of reference standard compounds using Agilent MassHunter Quantitative Analysis Software (Rev. B.05.02).
EST library construction
Normalized cDNA library of G. triflora was synthesized from a mixture of total RNA extracted from leaf and SAM of vegetative and reproductive growth phases, OWBs of endodormancy and ecodormancy phases, and flowers of G. triflora, sequenced by Roche 454 FLX Titanium instrument and assembled using a GS De Novo Assembler (Newbler) v2.5.3 software (Roche Applied Science). Preparation of the library, sequencing, and sequence assembly was performed as custom service by Generis, Inc (Yokohama, Kanagawa, Japan). Sequences of gentian orthologs were searched by local blast search against the cDNA library using the protein sequences of Arabidopsis or grape FLC, SOC1, FUL, SPL4, AP1, and LFY (Supplemental Figure S3) as queries using Genetyx-Mac version 19 software (Genetyx Corporation).
Molecular cloning and reverse transcription-quantitative PCR analysis
RNA extraction, first-strand cDNA synthesis, and reverse transcription-quantitative polymerase chain reaction (RT-qPCR) analysis were carried out according to the methods described in Takahashi et al. (2014). Briefly, total RNA was extracted using an RNAs-ici!-P kit (Rizo Inc.), and incubated with RNase-free DNase I (Wako) to eliminate genomic DNA. First-strand cDNA was synthesized from 500 ng of total RNA using a TaKaRa RNA PCR kit (AMV) Ver.3.0 (Takara) with an oligo(dT) primer according to the manufacturer’s instructions. The first-strand cDNA containing a full-length ORF was amplified by PCR, cloned into a pCR-Blunt vector (Thermo Fisher Scientific), and sequenced using an ABI PRISM Big Dye Terminator Cycle Sequencing kit with an ABI 3100 DNA sequencer (Applied Biosystems). For phylogenetic analysis, amino acid sequences were aligned with a gap open cost 10.0 and a gap extension cost 1.0, and phylogenetic tree was constructed by the UPGMA method using CLC Sequence Viewer v8.0 (CLC bio; Supplemental Figure S7). For RT-qPCR analysis, cDNA was synthesized from 500 ng of the total RNA using a High-Capacity cDNA Reverse Transcription kit (Applied Biosystems) according to the manufacturer’s instructions and used as the template. RT-qPCR was conducted using a KAPA SYBR FAST ABI Prism qPCR Kit (Kapa Biosystems) on a StepOnePlus Real-Time PCR System (Applied Biosystems). The expression levels of corresponding genes were calculated by the ΔΔCT method (Livak and Schmittgen, 2001). The gentian actin (ACT) gene was used as an internal control and to normalize the relative expression levels of all samples. Primers used in this study are listed in Supplemental Table S1.
Statistical methods
All statistical analyses were performed using the Statistical Package for the Social Sciences (SPSS v.10.0). Statistical analysis was performed with Student’s t test and a difference at P < 0.05 was considered as significant.
Accession numbers
Sequence data from this article can be found in DDBJ databases under the following accession numbers: GtFT1 (AB605176), GtFT2 (AB605177), GtFLC-L (LC577833), GtSOC1a (LC577834), GtSOC1b (LC577835), GtFUL (LC577836), GtSPL4 (LC577837), GtAP1 (LC577838), GtLFY (LC577839), GtSVP-L1 (LC049617), and ACT (LC189205).
Supplemental data
The following materials are available in the online version of this article.
Supplemental Figure S1. Genomic structure and nucleotide sequence of the gentian FT2 gene.
Supplemental Figure S2. Phenotypes of gentian ft2 mutants.
Supplemental Figure S3. Alignments of the deduced amino acid sequences of FLC, SOC1, FUL, SPL4, AP1, and LFY in gentian, Arabidopsis, and grape.
Supplemental Figure S4. CRISPR/Cas9-mediated mutations in the gentian FUL gene.
Supplemental Figure S5. Effect of chilling on the expression of GtFUL in WT overwintering buds.
Supplemental Figure S6. Schematic diagrams of the T-DNA region of the binary vectors used for genome editing in this study.
Supplemental Figure S7. Sequence alignment and phylogenetic tree based on the deduced amino acid sequences of GtFUL and its homologs from Arabidopsis thaliana (AtFUL), Cornus kousa (CkFUL1), Hydrangea macrophylla (HmFUL1), and Vitis vinifera (VvFUL) using the UPGMA method.
Supplemental Table S1. A list of gene accession numbers and primers used in this study.
H.T. and M.N. designed the research; H.T., M.N., and C.Y. performed the research; H.T. and K.I. analyzed the data; H.T., M.N., and K.I. wrote the paper.
The author responsible for distribution of materials integral to the findings presented in this article in accordance with the policy described in the Instructions for Authors (https://dbpia.nl.go.kr/plphys/pages/general-instructions) is: Hideyuki Takahashi ([email protected]).
Acknowledgments
The authors wish to thank Dr Murat Aycan, Ms Sayaka Fujisaki, Ms Ayumi Obara, Ms Yuko Kanno, and Ms Rie Washiashi for their technical assistance.
Funding
This study was supported by a SUNBOR grant from the Suntory Foundation for Life Sciences, JSPS KAKENHI (Grant Numbers 15H04454 and 19K06039), and supported in part by the administration of an individual commissioned project (Development of New Varieties and Breeding Materials in Crops by Genome Editing) from the Ministry of Agriculture, Forestry, and Fisheries, Japan (Grant Number 19190722).
Conflict of interest statement. None declared.
References
Author notes
Senior author.