-
PDF
- Split View
-
Views
-
Cite
Cite
Xingxuan Bai, Gangming Zhan, Shuxin Tian, Huan Peng, Xiaoyu Cui, Md Ashraful Islam, Farhan Goher, Youzhi Ma, Zhensheng Kang, Zhao-Shi Xu, Jun Guo, Transcription factor BZR2 activates chitinase Cht20.2 transcription to confer resistance to wheat stripe rust, Plant Physiology, Volume 187, Issue 4, December 2021, Pages 2749–2762, https://doi.org/10.1093/plphys/kiab383
- Share Icon Share
Abstract
The brassinosteroid pathway promotes a variety of physiological processes in plants and the brassinosteroid insensitive1-ethylmethane sulfonate suppressor (BES)/brassinazole-resistant (BZR) functions as one of its key regulators. We previously showed that the BES/BZR-type transcription factor TaBZR2 mediates the drought stress response in wheat (Triticum aestivum) by directly upregulating the transcriptional activity of glutathione S-transferase 1. However, the function of TaBZR2 in plants under biotic stresses is unknown. In this study, we found that transcript levels of TaBZR2 were upregulated in response to inoculation with wheat stripe rust fungus (Puccinia striiformis f. sp. tritici, Pst) and treatment with flg22 or an elicitor-like protein of Pst, Pst322. Wheat lines overexpressing TaBZR2 conferred increased resistance, whereas TaBZR2-RNAi lines exhibited decreased resistance to multiple races of Pst. TaBZR2 targeted the promoter of the chitinase gene TaCht20.2, activating its transcription. Knockdown of TaCht20.2 in wheat resulted in enhanced susceptibility to Pst, indicating the positive role of TaCht20.2 in wheat resistance. Upon Pst infection in vivo, the overexpression of TaBZR2 increased total chitinase activity, whereas RNAi-mediated silencing of TaBZR2 reduced total chitinase activity. Taken together, our results suggest that TaBZR2 confers broad-spectrum resistance to the stripe rust fungus by increasing total chitinase activity in wheat.
Introduction
Bread wheat (Triticum aestivum L.) is a cereal crop that is widely grown throughout the world. Wheat stripe rust, caused by the basidiomycetous fungus Puccinia striiformis f. sp. tritici (Pst), has become one of the most important biotic threats to wheat production worldwide (Chen et al., 2014; Schwessinger, 2017). The most economical, effective, and environmentally sound strategy to control this disease is to breed wheat-resistant varieties (Fisher et al., 2012). However, the rapid virulence variation of Pst leads to resistance breakdown of the existing resistant wheat cultivars, which then leads to the constant epidemic of the disease (Chen et al., 2014). Since the naming of Chinese yellow rust 1 (CYR1) in 1957 (Wang et al., 1963), a total of 34 Pst races have been officially named and the currently prevalent Pst races in China are CYR32, CYR33, and CYR34 (Liu et al., 2017). Therefore, a deep understanding of the molecular mechanism of the Pst–wheat interaction will enable us to develop strategies such as rational utilization of broad-spectrum resistance (BSR) genes to durably control stripe rust (Chen et al., 2014; Brown, 2015; Li et al., 2020). However, little work has been reported about wheat BSR to stripe rust fungus.
Brassinosteroid (BR) is a plant-specific steroid hormone with much higher physiological activity than auxin (indoleacetic acid, IAA), gibberellins (GA), cytokinin (CTK), abscisic acid (ABA), and ethylene (ET; Nolan et al., 2017; Song, 2017). BRI1-EMS suppressor (BES)/brassinazole-resistant (BZR) transcription factors are key components of the BR signaling pathway (Li et al., 2010). Numerous studies on BES/BZRs have contributed substantial progress to our knowledge of signal transduction pathways regulating plant growth and development (Oh et al., 2012; Qiao et al., 2017). In rice, OsBZR1 represses frizzy panicle gene (FZP) expression and thereby promotes spikelet development and increases rice yield (Bai et al., 2017). Arabidopsis AtBES1 directly binds to the BRI1-like receptor genes 3 (AtBRL3) promoter region and modulates its transcription in different subsets of cells of the root apex (Salazar-henao et al., 2016). AtBZR1 has a BR signaling-independent regulatory role in anther development, and it regulates locule development by affecting sporocyteless (SPL) expression in Arabidopsis thaliana (Chen et al., 2019). In soybean, phosphatase 2C-1 (GmPP2C-1) facilitates accumulation of dephosphorylated GmBZR1, and promotes seed size/weight and increases production (Lu et al., 2017). In addition, BZR/BESs participate in the light signaling pathway. In Arabidopsis, AtBZR1 interacts with phytochrome-interacting factor 4 (AtPIF4) to form a heterodimer and participates in photomorphogenesis. Dephosphorylated AtBES1 interacts with UV Resistance Locus 8 (AtUVR8) to regulate photomorphogenesis. AtBES1-BR enhanced expression 1 (AtBEE1)-Flowering locus T module is a recently discovered signaling pathway in regulating photoperiodic flowering. The blue light-mediated Cryptochrome 1 (AtCRY1)-BR Insensitive 2 (AtBIN2)–AtBZR1 module adjusts the crosstalk between blue light and BR signals (Liang et al., 2018; Wang et al., 2019).
A few studies have further indicated that BES/BZRs participate in tolerance to abiotic and resistance to biotic stress in plants. AtWRKY54 directly interacts with AtBES1 to cooperatively regulate the expression of target genes and promotes drought tolerance (Chen et al., 2017). AtBZR1 positively regulates freezing tolerance via C-repeat binding factor (CBF)-dependent and CBF-independent pathways in Arabidopsis (Li et al., 2017). Responsive to desiccation 26 (AtRD26) can interact with AtBES1 protein and antagonize AtBES1 transcriptional activity, they can coordinate plant growth and drought tolerance in Arabidopsis (Ye et al., 2017). Under low phosphorus stress, AtBES1 substantially accumulates to maintain normal root growth and enhances tolerance in Arabidopsis (Singh et al., 2014). Differential transcript abundance levels of multiple BrBZRs against high salt, drought, and low temperature stresses were also observed in expression profiling of Brassica rapa (Saha et al., 2015). With respect to BES/BZRs involved in biotic stress response, AtBES1 is phosphorylated by mitogen-activated protein kinase 6 (MPK6) and enhances Arabidopsis resistance against Pseudomonas syringae pv. tomato DC3000 (Kang et al., 2015). AtBES1 directly targeted the terminator regions of Plant defensin 1.2a/1.2b (PDF1.2a/PDF1.2b) and suppressed their expression, and AtBES1 gain-of-function mutant is highly susceptible to Spodoptera exigua and Botrytis cinerea (Liao et al., 2020). Moreover, AtBZR1 may regulate the jasmonic acid signaling pathway and enhance plant resistance to insects (Miyaji et al., 2014). However, the molecular mechanism of action of BES/BZRs in cereal plants under biotic stress has not been reported.
Our previous study showed that BES/BZR-type transcription factor TaBZR2 functions positively in drought stress response by activating transcriptional activity of glutathione S-transferase 1 (TaGST1) (Cui et al., 2019). In the present study, we further found that TaBZR2 confers BSR to Pst by activating TaCht20.2 transcription, thereby increasing chitinase activity in wheat during Pst infection. Chitinases are a class of pathogenesis-related proteins involved in plant disease resistance (Zha et al., 2016; Rawat et al., 2017). Combined with our previous report, we conclude that TaBZR2 positively regulates wheat resistance to stripe rust through a different pathway than the one by which it enhances drought tolerance. Our results provide insights toward understanding the roles of BES/BZR-type transcription factors in plant resistance.
Results
TaBZR2 transcription is significantly induced by Pst infection, flg22, and Pst322
Our previous work has shown that transcript levels of TaBZR2 are induced by drought and exogenous BR (Cui et al., 2019). To determine the roles of TaBZRs in disease resistance, we initially analyzed the gene transcript levels by the time series dual RNA-seq data using wheat plant inoculated with Pst (Accession number CNSA: CNP0001524, https://db.cngb.org/cnsa/). Fragments per kilobase of exon per million mapped reads data of TaBZRs indicated that TaBZR2 was upregulated in the early stages of the incompatible and compatible interactions, whereas TaBZR1/3/4 showed nonsignificant changes (Supplemental Figure S1 and Supplemental Table S1). Thus, TaBZR2 was determined for further characterization. Reverse transcription quantitative polymerase chain reaction (RT-qPCR) was further performed to verify TaBZR2 transcription identified from the RNA-seq analysis (Figure 1A) and TaBZR1 was used a negative control (Supplemental Figure S2). During an incompatible interaction, TaBZR2 transcripts were upregulated as early as 3-h post inoculation (hpi), with peak expression at 18 hpi, and then transcript levels declined to pre-inoculation levels at 48–216 hpi. During a compatible interaction, TaBZR2 was also induced from 6 to 48 hpi (Figure 1A). However, transcript levels of TaBZR1 were hardly changed (Supplemental Figure S2).
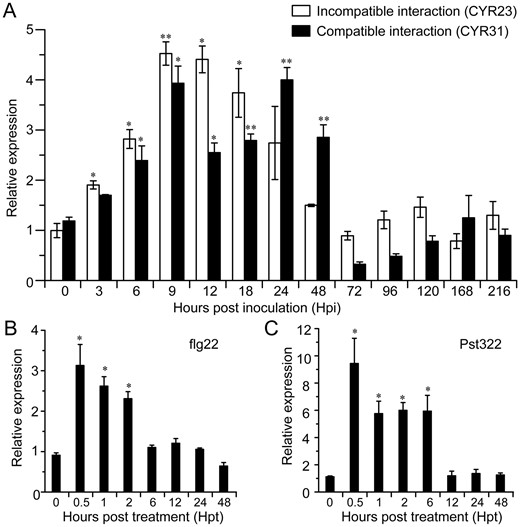
Transcript profiles of brassinazole-resistant 2 gene (TaBZR2) in wheat (Triticum aestivum, Suwon 11) leaves in response to Pst infection, flagelin 22 (flg22), and an elicitor-like protein of Pst (Pst322) treatment. A, The transcript levels of TaBZR2 in wheat leaves were induced during wheat–Pst interaction. Wheat leaves inoculated with CYR23 or CYR31 were sampled for RT-qPCR analysis and the leaves treated with distilled water were used as a control. B, The transcription of TaBZR2 in wheat response to flg22 treatment. C, The transcription of TaBZR2 in wheat response to Pst322 treatment. Wheat leaves treated with distilled water were included as a control. RT-qPCR values were normalized for elongation factor 1 alpha gene (TaEF-1α). Data are means ± se (n = 3). Each time point sample was compared with 0 h by Student’s t test (*P < 0.05; **P < 0.01).
Previous studies have shown that Pst322 induces programmed cell death in both host and non-host plants and functions as an elicitor-like protein with a broad spectrum activity (Wang et al., 2012; Qi et al., 2019). To explore whether TaBZR2 is involved in wheat immunity, we next evaluated the transcript levels of TaBZR2 in response to treatment with flg22 and Pst322. When wheat seedlings were treated with flg22, the transcript levels of TaBZR2 were significantly induced at 0.5- to 2-h post treatment (hpt), with an approximate three-fold change in expression (Figure 1B). When we used the bacterial type III secretion system (TTSS)-mediated overexpressing Pst322 in wheat plants, the transcript levels of TaBZR2 were significantly induced at 0.5–6 hpt, with an approximate six- to nine-fold increase in expression (Figure 1C).
Overexpression of TaBZR2 confers BSR to Pst in wheat
To investigate the possible role of TaBZR2 in defense against rust fungus infection in wheat, we developed transgenic wheat plants using the “Fielder” wheat variety as background in which TaBZR2, driven by the maize Ubiquitin promoter, was overexpressed. We chose two stable T3 transgenic lines (TaBZR2-OE9 and TaBZR2-OE11) from our previous study (Cui et al., 2019), and they were further confirmed by polymerase chain reaction (PCR) and real-time quantitative PCR (RT-qPCR) assays (Supplemental Figure S3, A and B, and Supplemental Table S4). No significant difference was observed between the TaBZR2-OE plants and Fielder plants under normal growth conditions (Supplemental Figure S3C). To verify whether TaBZR2 function is specific to an individual Pst race, we used the CYR20, CYR22, CYR23, CYR25, CYR29, CYR31, CYR32, CYR33, and CYR34 races to examine the resistance of TaBZR2-OE lines. It is worth noting that the current prevalent Pst races in China are CYR32, CYR33, and CYR34 (Liu et al., 2017). We found that both Fielder plants and transgenic lines expressed disease-resistance phenotypes to CYR20 (Figure 2A), CYR22 (Figure 2B), CYR23 (Figure 2C), and CYR25 (Figure 2D). Their phenotypes were not obviously different, and there was no significant difference in fungal biomass (Figure 2, A–D). The Fielder plants and transgenic lines were only a few fungal uredia surrounding the necrotic areas were observed on leaves inoculated with CYR29 (Figure 2E) and susceptible to CYR31 (Figure 2F), CYR32 (Figure 2G), CYR33 (Figure 2H), and CYR34 (Figure 2I). Compared with Fielder plants, fungal biomass was significantly reduced in TaBZR2-OE lines (Figure 2, E–I).
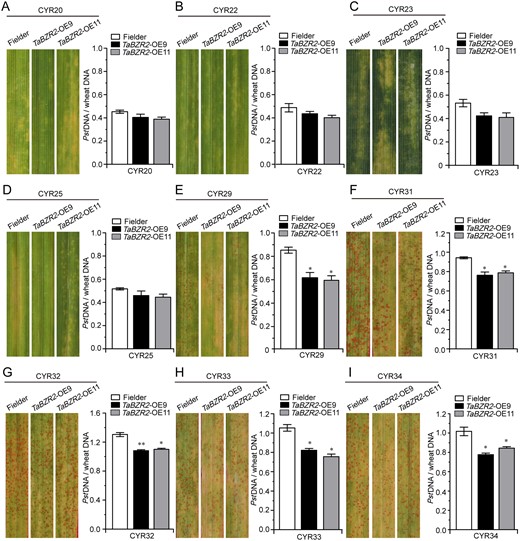
Overexpression of TaBZR2 enhances wheat resistance to Pst infection. Foliar parts of TaBZR2 overexpression (TaBZR2-OE) lines (OE9 and OE11) and Fielder inoculated with Pst races (A) Chinese yellow rust 20 (CYR20), (B) CYR22, (C) CYR23, (D) CYR25, (E) CYR29, (F) CYR31, (G) CYR32, (H) CYR33, and (I) CYR34. Ratio of fungal to wheat nuclear content determined using the contents of fungal elongation factor 1 (PstEF1) and wheat TaEF-1α genes, respectively. Genomic DNA was extracted from three different plants at 7 dpi. Data are means ± se (n = 3). Asterisks indicate significant differences between transgenic plants and Fielder plants at the same time points using Student’s t test (*P < 0.05; **P < 0.01).
PR1 is considered to be a marker for infection resistance in plants to improve disease resistance (Van loon and Van strien, 1999). PR2 has an antibacterial effect due to its hydrolytic enzyme function (Mauch et al., 1988). To confirm the increased resistance in TaBZR2-OE plants, the expression of pathogenesis-related (PR) genes was examined by RT-qPCR, and we found that transcript levels of TaPR1 and TaPR2 were significantly upregulated in TaBZR2-OE lines (Supplemental Figure S4, A–I). In addition, a burst of ROS occurs during the incompatible interaction between wheat and Pst (Wang et al., 2010), so we found that catalase 3 (TaCAT3; Hawku et al., 2021) was downregulated in TaBZR2-OE plants during the incompatible interaction (Supplemental Figure S4, A–D).
To further understand how TaBZR2 participates in wheat resistance, a histological examination of Pst growth was performed by analyzing the length of hyphae, the number of haustorial mother cells, and infection areas. We found that the length of hyphae (Supplemental Figure S5, A and B), the number of haustorial mother cells (Supplemental Figure S5, A and C) and infection areas (Supplemental Figure S5, A and D) between TaBZR2-OE plants and Fielder plants hardly changed at 48 hpi. The infection areas per infection site were obviously smaller in TaBZR2-OE plants compared with Fielder plants at 120 hpi (Supplemental Figure S5, A and D). Next, H2O2 accumulation around infection sites was evaluated. H2O2 production in TaBZR2-OE plants were significantly increased compared with Fielder plants (Supplemental Figure S6, A and B). Overall, these results suggest that overexpression of TaBZR2 confers BSR to Pst in wheat.
Suppression of TaBZR2 reduces wheat resistance to Pst infection
To further investigate the function of TaBZR2 in response to Pst, we chose two RNAi-mediated stable T2 (TaBZR2-Ri3 and TaBZR2-Ri7) knockdown plants from our previous study (Cui et al., 2019), and they were further confirmed by PCR and RT-qPCR assays (Supplemental Figure S7, A and B and Supplemental Table S4). No significant difference was observed between the TaBZR2-RNAi plants and Fielder plants under normal growth conditions (Supplemental Figure S7C). At 14-d post inoculation (dpi), hypersensitive response (HR) symptoms appeared on all leaves inoculated with CYR20 (Figure 3A), CYR22 (Figure 3B), CYR23 (Figure 3C), and CYR25 (Figure 3D) and a few fungal uredia surrounding the necrotic areas were observed on leaves of TaBZR2-RNAi plants. Compared with Fielder plants, fungal biomass was significantly increased in RNAi plants (Figure 3, A–D). Moreover, the Fielder plants and RNAi lines exhibited susceptibility to CYR29 (Figure 3E), CYR31 (Figure 3F), CYR32 (Figure 3G), CYR33 (Figure 3H), and CYR34 (Figure 3I). Compared with Fielder plants, fungal biomass in RNAi plants was significantly increased (Figure 3, E, G–I). In contrast to TaBZR2-OE plants, TaPR1 and TaPR2 were expressed at lower levels than those in Fielder plants (Supplemental Figure S4, A–I), while the TaCAT3 was upregulated in TaBZR2-RNAi plants during the incompatible interaction (Supplemental Figure S4, A–D).

Silencing of TaBZR2 reduces wheat resistance to Pst infection. Foliar parts of TaBZR2-RNAi (TaBZR2-Ri3/Ri7) and Fielder inoculated with Pst isolate (A) Chinese yellow rust 20 (CYR20), (B) CYR22, (C) CYR23, (D) CYR25, (E) CYR29, (F) CYR31, (G) CYR32, (H) CYR33, and (I) CYR34. Ratio of fungal to wheat nuclear content determined using the contents of fungal PstEF1 and wheat TaEF-1α genes, respectively. Genomic DNA was extracted from three different plants at 7 dpi. Data are means ± se (n = 3). Asterisks indicate significant differences between transgenic plants and Fielder plants at the same time points using Student’s t test (*P < 0.05; **P < 0.01).
As in the previous step, we investigated Pst growth. We found that the length of hyphae (Supplemental Figure S8, A and B), the number of haustorial mother cells (Supplemental Figure S8, A and C) and infection areas (Supplemental Figure S8, A and D) in TaBZR2-RNAi plants and Fielder plants hardly changed at 48 hpi. The infection areas per infection site were obviously larger in TaBZR2-RNAi plants compared with Fielder plants at 120 hpi (Supplemental Figure S8, A and D). After infection, H2O2 accumulation around infection sites in RNAi plants were significantly decreased compared to Fielder plants (Supplemental Figure S9, A and B). These results suggest that reduction in TaBZR2 expression compromises resistance in response to Pst infection.
Sequence analysis and transcript profiles of TaCht20.2
To further explore the disease resistance mechanism of TaBZR2, we performed RNA-Seq assays to evaluate the differential gene expression between TaBZR2-OE and Fielder plants challenged by Pst infection. Differentially expressed genes (DEGs) were selected with a relative change threshold of two-fold (P < 0.05, false discovery rate <0.01). Gene ontology (GO) analysis revealed that the DEGs between TaBZR2-OE and Fielder plants were significantly enriched in “chitin binding” and “chitinase activity” during wheat–Pst interaction (Supplemental Figure S10). We found that seven chitinase genes may be regulated by TaBZR2 (Supplemental Table S2, yellow label and Supplemental Table S3). Sequence analysis revealed that all seven chitinases contain a GH19 (Glyco_hydro_19) domain. To explore the differences among wheat chitinases and putative orthologs from other plant species, a phylogenetic tree was constructed by amino acid sequence alignment using MEGA7 (Kumar et al., 2016). We identified 77 chitinases containing the GH19 domain and highlighted two clusters. Compared with the traditional chitinase classification (Taira et al., 2009), most of the wheat chitinases in cluster I belong to the traditional Class I, but TaCht12.1, TaCht13.2, TaCht13.3, TaCht14, TaCht16.1, TaCht16.2, and TaCht16.3 belong to Class II. Most of the wheat chitinases in cluster II belong to the traditional Class II, but TaCht8.2 and TaCht6 belong to Class I. Then we named wheat chitinases from TaCht1 to TaCht23 with reference to the rice name (Supplemental Figure S11).
To validate which of the seven chitinases are regulated by TaBZR2, we used TaBZR2-OE lines to detect their expression during Pst infection. We found that all seven chitinases were upregulated by Pst infection, but TaCht20.2-5B and TaCht19-2A in TaBZR2–OE lines were significantly upregulated compared to those in Fielder plants (Supplemental Figure S12). Because the upregulation of TaCht20.2-5B is much higher than TaCht19-2A, we selected TaCht20.2-5B for further research.
According to the sequences identified in the genome of Chinese Spring wheat, the full-length cDNA of TaCht20.2, encoding a putative protein composed of 251 amino acid residues with a molecular weight of 27.1 kDa and an isoelectric point (pI) of 5.68, was obtained from wheat cv Suwon11 by RT-PCR. During incompatible and compatible interactions, TaCht20.2 transcripts were upregulated at 18 hpi, with peak expression at 48 hpi, and transcript levels gradually declined to pre-inoculation levels at 96–168 hpi (Supplemental Figure S13).
TaBZR2 functions as a positive regulator of TaCht20.2 expression by binding to its promoter and activating transcription
Previous studies have indicated that BES/BZR transcription factors regulate the expression of BR-responsive genes by binding to the E-box (CANNTG) in BR-induced genes, and the BRRE (CGTGT/CG) element in BR-repressed genes (He et al., 2005; Yin et al., 2005; Yu et al., 2011). We found that TaBZR2 significantly induces TaCht20.2 (Supplemental Figure S12). Coincidentally, we detected four E-box cis-elements in the TaCht20.2 promoter (Figure 4A). Thus, we used electrophoretic mobility shift assay (EMSA) to investigate whether TaBZR2 can directly bind to the TaCht20.2 promoter in vitro. The EMSAs indicated that the TaBZR2–His fusion protein bound to all of cis-elements in the TaCht20.2 promoter, whereas no such binding was observed for the control His protein (Figure 4B). Further, the observed binding to the biotin-labeled target sequences was substantially reduced when unlabeled competitor target DNA sequences were added, and no binding was detected when the mutated biotin-labeled target sequences were added (Figure 4B).

TaBZR2 directly regulates the expression of chitinase20.2 (TaCht20.2). A, The diagram shows the structure of the TaCht20.2 promoter (normal and mutated). The E-box, 5′-untranslated region (UTR), and initiation codon (ATG) were distinguished with the black, gray, and white boxes, respectively. B, EMSA of TaBZR2 binding to the promoter of TaCht20.2. Biotin-labeled TaCht20.2 probes (normal and mutated) were incubated with His or TaBZR2-His protein, and 20 × competitor fragments were added to analyze the specificity of binding. C, TaBZR2 increases TaCht20.2 promoter activity in wheat protoplasts. TaBZR2 were co-transfected with either TaCht20.2 promoter or mutated TaCht20.2 promoter. The firefly LUC /REN ratio is shown and indicates the activity of the transcription factors on the expression level of the promoters. Data are means ± se (n = 3). Asterisks indicate significant differences between transformed plants and control at the same time points using Student’s t test (*P < 0.05).
Having determined that TaBZR2 binds to the TaCht20.2 promoter in vitro, we then used a wheat protoplast transient expression system to assess whether this binding can drive TaCht20.2 gene expression in vivo. A pGreen II 0800 vector harboring a Luciferase (LUC) reporter gene driven by the TaCht20.2 promoter (∼2,000 bp) was co-transformed into wheat protoplasts transfected with an empty pJIT16318 vector, or a pJIT16318-TaBZR2 vector. Compared with the empty-vector (control) samples, the protoplasts expressing TaBZR2 exhibited significantly increased expression of the reporter (Figure 4C). To further test if the activation effect of TaBZR2 on the TaCht20.2 is through binding to the E-box, the TaCht20.2 promoter containing the mutated E-box was inserted into pGreen II 0800 vector and co-expressed with pJIT16318-TaBZR2 vector into wheat protoplasts. The results demonstrated that the mutation disrupted TaBZR2-mediated activation of the TaCht20.2 promoter (Figure 4C), indicating that the TaBZR2 transcription factor is a positive regulator of the TaCht20.2 expression.
Suppression of TaCht20.2 reduces wheat resistance to Pst infection
To characterize the function of TaCht20.2 in the wheat defense response to Pst, barley stripe mosaic virus (BSMV)–virus-induced gene silencing (VIGS), a rapid and effective approach for analyzing gene function in wheat and barley, was used (Scofield et al., 2005), and many reports have shown that this system is effective for analyzing the Pst–wheat interaction (Liu et al., 2019; Qi et al., 2019). Two specific fragments of TaCht20.2 cDNA were selected for the silencing experiments. The mild chlorotic mosaic symptoms appeared on BSMV-inoculated plants, and BSMV:TaPDS as infected plants exhibited strong photobleaching at 10 dpi (Figure 5A), indicating that the BSMV-mediated gene silencing system performed correctly. The third leaf of all wheat plants was then inoculated with urediospores of Pst races CYR23 and CYR31. At 14 dpi with Pst, HR symptoms appeared on all leaves after inoculation with the incompatible strain CYR23 and a few fungal uredia surrounding the necrotic areas were observed on leaves of the TaCht20.2-knockdown plants. In contrast, all leaves infected with the compatible strain CYR31, exhibited normal disease development (Figure 5A). To test the silencing efficiency of TaCht20.2, RT-qPCR was used to assay the relative transcript level of TaCht20.2 at 0, 24, 48, and 120 hpi during incompatible and compatible interaction. Compared with BSMV:γ (control) plants, the transcript level in the BSMV:TaCht20.2-1as/2as (TaCht20.2-knockdown) plants were reduced by 47%–67% (Figure 5B). Compared with control plants, fungal biomass in knockdown plants were significantly increased after inoculation with CYR23 (Figure 5C).
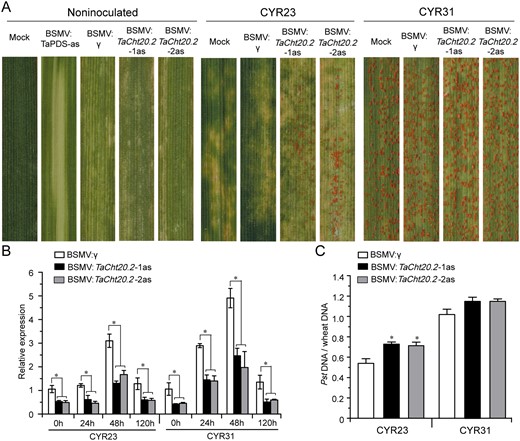
Silencing of TaCht20.2 reduces wheat resistance against Pst infection. A, Mild chlorotic mosaic symptoms were observed on leaves inoculated with BSMV:γ, BSMV:phytoene desaturase (TaPDS)-antisense (as) and BSMV:TaCht20.2-1/2as at 10 dpi. Mock wheat leaves were treated with 1 × FES (viral inoculation buffer). Photos show fourth leaves of TaCht20.2-knockdown plants inoculated with urediospores of the avirulent race CYR23 or the virulent race CYR31. Typical leaves were photographed at 14 dpi. B, Relative expression of TaCht20.2 during the interaction between TaCht20.2-knockdown plants and CYR23 and CYR31. The RT-qPCR values were normalized to those for TaEF-1α and are presented as fold changes relative to those in plants with BSMV:γ treatment at time 0. C, Ratio of fungal to wheat nuclear content determined using the contents of fungal PstEF1 and wheat TaEF-1α genes, respectively. Genomic DNA was extracted from three different plants at 7 dpi. Data are means ± se (n = 3 in B and C) Asterisks indicate significant differences between BSMV:TaCht20.2-1/2as plants and BSMV:γ at the same time points using Student’s t test (*P < 0.05)
In addition, we found that the length of hyphae (Supplemental Figure S14, A and B), the number of haustorial mother cells (Supplemental Figure S14, A and C) and infection areas (Supplemental Figure S14, A and D) in knockdown plants and control plants hardly changed at 48 hpi, and the infection areas per infection site were obviously larger in knockdown plants compared with control plants at 120 hpi (Supplemental Figure S14, A and D). After Pst infection, H2O2 accumulation around infection sites was significantly decreased in knockdown plants compared to control plants after Pst infection (Supplemental Figure S15, A and B) and transcript level of TaCAT3 were significantly increased in all knockdown plants (Supplemental Figure S15C). Taken together, these results indicate that the silencing of TaCht20.2 diminishes the wheat defense response against Pst infection.
TaBZR2 increases total chitinase activities during Pst infection in vivo
As the previous results showed that TaBZR2 induces the expression of chitinase genes during Pst infection, we wanted to know whether TaBZR2 can affect the total chitinase activities in wheat. We found that total chitinase activities were substantially elevated at 48 hpi. Moreover, total chitinase activity levels in the two TaBZR2-OE lines were significantly higher than those in Fielder plants (Figure 6). In contrast, total chitinase activity levels in the two TaBZR2-RNAi lines were substantially lower relative to those in Fielder plants (Figure 6). In addition, transcript levels of the TaCht20.2 in TaBZR2-OE plants increased to higher levels than those in Fielder plants inoculated with other seven races, while in the TaBZR2-RNAi lines were downregulated (Supplemental Figure S16, A–G). Therefore, TaBZR2 increased total chitinase activities that ultimately increased resistance to Pst infection.
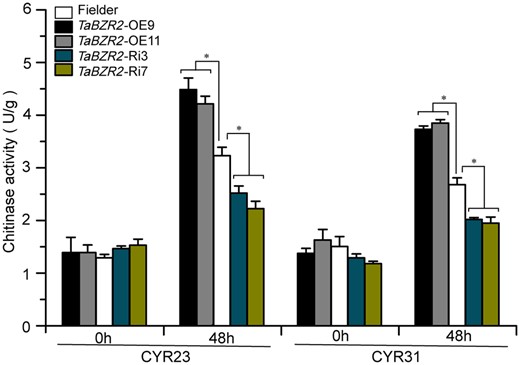
Measure of total chitinase activities in TaBZR2 overexpression (TaBZR2-OE), TaBZR2-RNAi, and Fielder inoculated with CYR23 and CYR31. The Fielder was used as control. The samples used for the assay were collected at 0 h and 48 hpi. U indicates units of enzymes activity. Asterisks indicate significant differences between transformed plants and control at the same time points using Student’s t test (*P < 0.05).
TaGST1 does not have a major role during Pst infection
Our previous study indicated that TaBZR2 directly binds to the E-box cis-element in the TaGST1 promoter region and activates its expression in drought resistance, which functions positively in scavenging drought-induced superoxide anions (O2−; Cui et al., 2019). In this study, TaBZR2 can positively regulate the H2O2 accumulation around infection sites, and it can negatively regulate the transcript levels of TaCAT3 during wheat–Pst interaction. So we further explored the function of TaGST1 during Pst infection. Transcript levels of TaGST1 were induced by Pst infection (Supplemental Figure S17). Further, using the BSMV-VIGS system we selected one specific fragment of TaGST1 for the silencing experiments. BSMV:TaPDS infected plants exhibited strong photobleaching at 10 dpi (Supplemental Figure S18A), indicating that the BSMV-mediated gene silencing system functioned correctly. The third leaf of all wheat plants were then inoculated with urediospores of Pst race CYR23 and CYR31. At 14 dpi, there was no obvious phenotypic difference between BSMV:TaGST1-as and BSMV:γ plants during incompatible and compatible interaction (Supplemental Figure S18A). The fungal biomass also supported the phenotypic results (Supplemental Figure S18B). The silencing efficiency of TaGST1 was tested by RT-qPCR assay, compared with control plants, the transcript levels in the BSMV:TaGST1-as plants were reduced by 54%–73% (Supplemental Figure S18C).
To determine whether TaBZR2 activates TaGST1 during Pst infection, the TaGST1 transcript levels in TaBZR2-OE and TaBZR2-RNAi plants were measured during Pst infection. We found no difference in TaGST1 transcript levels during incompatible and compatible interaction (Supplemental Figure S19). These results suggested that TaGST1 does not have a major role during wheat–Pst interaction.
Discussion
BES/BZRs are specific transcription factors of the BR signaling pathway, which regulate plant growth and development by mediating BR signaling. In recent years, several studies have shown that BZR1 also plays an important role in light signal and stress responses (Saha et al., 2015; Fan et al., 2018), and engages in signal crosstalk with ABA, GA, IAA, and ET (Oh et al., 2014; Tang et al., 2011, 2018; Liu et al., 2018). This indicates that the BES/BZRs transcription factors perform multiple functions in plants. TaBZR2 positively regulates drought tolerance by mediating the crosstalk between BR and drought signaling pathways in wheat (Cui et al., 2019). Exogenous BR improves wheat tolerance to salt stress (Liu et al., 2018). However, it has been previously reported that AtBES1 negatively regulates its drought tolerance in Arabidopsis (Chen et al., 2017). In this article, TaBZR2 is positively involved in the resistance to the stripe rust fungus by increasing chitinase activity, but AtBES1 negatively regulates its resistance against Spodoptera exigua and Botrytis cinerea (Liao et al., 2020). Based on the phylogenetic tree of BZRs (Cui et al., 2019), we found that the BZRs derived from monocots clustered separately from those of dicots, suggesting a potential functional diversity between dicot and monocot plants. We speculate that BZRs in Arabidopsis and wheat may have acquired different functions during evolution. AtBES1 was directly phosphorylated by MPK6 and involved in resistance to pathogens under the induction of pathogen-associated molecular pattern (Kang et al., 2015), and phosphorylated AtBES1 was shown to accumulate in the cytoplasm (Wang et al., 2002; Yin et al., 2002). In our study, Pst, flg22, and Pst322 induced an increase in the expression of TaBZR2 (Figure 1) and the transcription of the downstream gene TaCht20.2 is activated (Figure 4). Moreover, drought and exogenous BR treatments increased the nuclear accumulation of TaBZR2 protein (Cui et al., 2019). Therefore, we speculate that Pst, flg22, and Pst322 can induce the nuclear accumulation and dephosphorylation of TaBZR2 protein and the resistance signaling pathways of BZRs in Arabidopsis and wheat may differ in response to biotic stress.
Chitinases are potential candidates for enhancing plant resistance toward fungal pathogens (Kumar et al., 2018). Research on wheat chitinase is very helpful in breeding programs for developing wheat varieties that are resistant to powdery mildew and stripe rust fungi (Anguelova-merhar et al., 2002; Mohammadi et al., 2002). However, in recent years, research on chitinase in wheat mainly aims to clone a single chitinase gene, and there is a lack of subsequent studies on chitinase in wheat (Liao et al., 1994; Li et al., 2001). The presence of complex heterohexaploidy in common wheat and the possibility that the wheat genome contains multiple copies of chitinases may account for the lack of progress. A total of 77 chitinases containing the GH19 domain were systematically identified in this study (Supplemental Figure S11), and TaBZR2 was able to induce the upregulation of TaCht20.2 and TaCht19 based on RT-qPCR analyses (Supplemental Figure S12). Furthermore, we found that TaBZR2 can upregulate total chitinase activity and improve disease resistance (Figure 6). Therefore, we speculated that TaBZR2 may also regulate other members in addition to TaCht20.2 and TaCht19. Our previous study has reported that TaBZR2 directly binds to TaGST1 promoter region and activates its expression in drought tolerance (Cui et al., 2019). GST is a superfamily of proteins with multiple functions and participates in many abiotic stress responses, such as salt stress, drought stress, low temperature stress, heavy metal pollution, and herbicide toxicity (Chi et al., 2011). In addition, in this study, our data indicated that TaGST1 does not play a major role in wheat resistance to Pst infection (Supplemental Figures S18 and S19). Therefore, we speculated that TaBZR2 can bind TaGST1 promoter region, but does not activate its transcription during wheat–Pst interaction.
This is direct evidence demonstrating that the roles of TaBZRs are associated with chitinases during the Pst–wheat interaction. Additionally, the TaBZR2-OE wheat plants expressed BSR to multiple races of Pst. Combined with a previous report that TaBZR2 transcription factor contributes to drought tolerance in wheat (Cui et al., 2019), we speculate that the concentration of TaBZR2 in plant cells is affected by Pst infection and it positively regulates TaCht20.2 expression by binding to its promoter and activating transcription. As a result, the chitinase activity is increased in vivo. Moreover, TaBZR2 may increase the expression of TaPRs and increase the content of H2O2 through other signaling pathways (Figure 7). Hence, we speculate that the concentration of TaBZR2 in plant cells is affected by drought and positively regulates TaGST1 expression by binding to its promoter and activating transcription. As a result, drought-induced O2− content is significantly reduced. Furthermore, TaBZR2 may also increase the expression of TaLEA3, TaDHN3, TaP5CS, TaPOD21, and TaSAPK3 through other signaling pathways (Cui et al., 2019; Figure 7). Therefore, we suggest that TaBZR2 confers wheat resistance to stripe rust and drought tolerance through different signaling pathways.
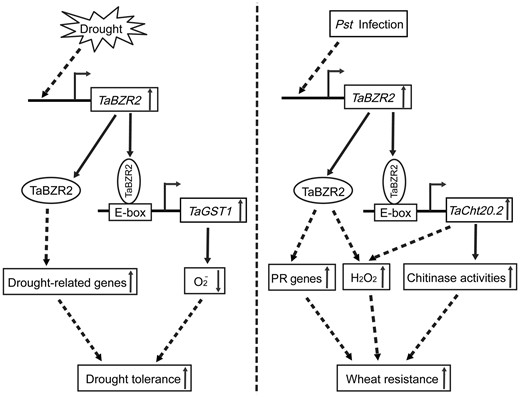
Working model of possible molecular mechanism of brassinazole-resistant 2 (TaBZR2)-mediated resistance and tolerance processes. The concentration of TaBZR2 in plant cells is affected by drought, and it positively regulates glutathione S-transferase 1 gene (TaGST1) expression by binding to the promoter (bent arrow) and activating transcription. As a result, drought-induced O2− content is significantly reduced. Furthermore, TaBZR2 may also increase the expression of drought-related genes through other signaling pathways (Left). After Pst infection, transcription of TaBZR2 is induced and positively regulates expression of TaCht20.2 by binding to its promoter (bent arrow) and activating transcription. As a result, the chitinase activity is increased in vivo. Additionally, TaBZR2 may also increase the expression of pathogenesis-related genes (TaPRs) and increase the H2O2 content through other signaling pathways (Right). In short, TaBZR2 improves wheat tolerance to drought and resistance to Pst infection. The left panel has been reported. Solid arrows indicate direct effects and dotted arrows indicate indirect effects.
Materials and methods
Gene transcription analysis, fungal pathogens, and treatments
The transcript level of all TaBZR genes was performed by time series dual RNA-Seq data. We sequenced two groups of wheat–rust interaction combination named NIL_R vs. CYR32 and NIL_S vs. CYR32 and selected the time points at 0, 18, 24, 48, 96, and 168 hpi. The specific method is the same as our previous study (Guo et al., 2018). Wheat plants used for molecular analysis were grown in a greenhouse at 25°C/23°C day/night temperatures, long-day conditions (16-h light/8-h dark photoperiod). The wheat cultivar Suwon 11 was used to amplify cDNA sequences of TaBZR2, TaCht20.2, and TaGST1. It is noteworthy that the sequences of TaBZR2 and TaGST1 are identical to the previously reported Kenong199 sequence (Cui et al., 2019). Pst races CYR20, CYR22, CYR23, CYR25, CYR29, CYR31, CYR32, CYR33, and CYR34, were used in this study. Inoculation procedure is the same as a previous study (Wang et al., 2007).
Suwon 11, carrying the YrSu gene, is resistant to CYR23 infection but highly susceptible to CYR31 (Cao et al., 2002). The second leaves inoculated with CYR23 or CYR31 or treated with sterile distilled water (control) were harvested at 0, 3, 6, 9, 12, 18, 24, 48, 72, 96, 120, 168, and 216 hpi for RNA extraction. Time points were selected based on a previous study (Wang et al., 2007). For flg22 treatments, 10-d-old wheat seedlings were sprayed with 500-mM flg22 dissolved in distilled water. Mock control plants were sprayed with distilled water. Samples were collected at 0, 0.5, 1, 2, 6, 12, 24, and 48 hpt for RNA extraction and RT-qPCR. Methods of gene cloning, sequence analysis of DNA, RNA extraction, cDNA synthesis, and RT-qPCR were performed as described in a previous study (Bai et al., 2021).
Fielder is a cultivated variety of spring wheat from USA, we used it as a background for transgenic experiments because of its high success rate of transformation in wheat (Chen, 2007; Hayta et al., 2019). Fielder contains Yr6 and Yr20 genes (Chen, 2007) and shows an incompatible interaction (avirulent) with Pst races CYR20, CYR22, CYR23, CYR25, and CYR29, and compatible interaction (virulent) with Pst races CYR31, CYR32, CYR33, and CYR34 (Bai et al., 2021).
Bacterial TTSS-mediated overexpression in wheat plants
The pEDV6:Pst322 construct was introduced into Pseudomonas fluorescens strain EtHAn by electroporation. For transient expression in wheat cells, bacterial cells carrying Pst322 were cultured overnight in King's B (KB) medium with appropriate antibiotics at 28°C (Yin and Hulbert, 2011), then collected and resuspended in an infiltration medium (10 mM MgCl2). The P. fluorescens strain EtHAn was diluted to an optical density (OD)600 of 0.6 and infiltrated into the second leaves of wheat cultivar Suwon 11 with a syringe without a needle. The infiltrated wheat plants were maintained in a growth chamber at 25°C and the wild-type strain EtHAn was used as a control. Samples were collected at 0, 0.5, 1, 2, 6, 12, 24, and 48 hpt for RNA extraction and RT-qPCR.
RNA extraction and histological observation
TaBZR2-OE and TaBZR2-RNAi transgenic wheat plants used were the same as previously reported (Cui et al., 2019). The second leaf of T3 generation TaBZR2-OE and T2 generation TaBZR2-RNAi plants were inoculated with urediospores of Pst. These leaves were collected at 0, 24, 48, and 120 hpi for RNA isolation and for histological observation. The phenotypes were photographed at 14 d after inoculation with Pst. Finally, the inoculated leaves were collected at 7 d to measure the biomass ratio by RT-qPCR (Qi et al., 2019).
H2O2 accumulation was detected by staining with diaminobenzidine (DAB; Thordal-christensen et al., 1997). The leaves inoculated with CYR23 were then sampled at 24, 48, 120 hpi for histochemical observation. The DAB staining was observed under white light. To obtain high-quality images of Pst infection structures in wheat leaves, wheat germ agglutinin conjugated to Alexa-488 (Invitrogen) was used as described (Ayliffe et al., 2011). Stained tissues were examined under blue light excitation (excitation wavelength 450–480 nm, emission wavelength 515 nm). Infection sites (30–50) from three leaves were examined to record the H2O2 areas, number of haustorial mother cells, hyphal length, and infection areas of hyphae in infected wheat per infection unit. The presence of a substomatal vesicle was defined as an established infection unit. Hyphal length of Pst was measured from the base of the substomatal vesicle to the apex of the longest infection hypha. All microscopic observations were conducted with a model BX-51 microscope (Olympus, Tokyo, Japan).
RNA-Seq assays
TaBZR2-OE9 plants and Fielder plants were grown in a greenhouse at 25°C/23°C day/night temperatures, long-day conditions (16-h light / 8-h dark photoperiod) for 2 weeks. The second leaves inoculated with CYR31 were harvested at 0 and 24 hpi for RNA extraction, and three biological replicates were used. The RNA-seq analysis was performed by the Novogene bioinformatics technologies Co. Ltd (Beijing). Total RNA was extracted from the samples using TRIzol reagent (Invitrogen, USA), and RNA sequencing was conducted on Illumina Novaseq platform. RNA sequencing data were analyzed as previously described (Mortazavi et al., 2008). DEGs were selected using DESeq2 (1.16.1) with a relative change threshold of two-fold (P < 0.05; Love et al., 2014). GO categories were identified using the clusterProfiler (3.4.4; Yu et al., 2012). The genome annotation and functional categorization are based on the National Center for Biotechnology Information nonredundant protein sequences (https://ftp.ncbi.nlm.nih.gov/blast/db/FASTA/).
EMSA
The coding sequence (CDS) of TaBZR2 was inserted into the pET-32a vector. The His and His–TaBZR2 fusion proteins were expressed in Escherichia coli (BL21) and purified with the 6× His-Tagged Protein Purification Kit (CWBIO, China) according to the manufacturer’s protocol. The biotin-labeled probes used in this assay were synthesized (Sangon Biotech, China), and the sequences are listed in Supplemental Table S2. Double-stranded DNA was obtained by boiling water for 2 min, and annealing at room temperature in water. The EMSA was performed using the Chemiluminescent EMSA Kit (Beyotime Biotechnology, China) according to the manufacturer’s instructions. In brief, 2 mg of purified fusion protein His-TaBZR2 or His protein were added to the binding reaction. The binding reaction was conducted at 25°C for 30 min in a Thermostatic metal bath (Coyote, USA). The mixture was separated on 4% polyacrylamide mini gel, and then the DNA was transferred to a nylon membrane (Millipore, USA). The signal was visualized with a BeyoECL Moon working solution in Chemiluminescent EMSA Kit.
Transcriptional activation assays in wheat protoplasts
For the transcriptional activation assay, the promoter fragment of TaCht20.2 was cloned from Suwon 11 and inserted into LUC reporter plasmid pGreen II 0800, which contained a Renilla luciferase (REN) under the control of the CaMV 35S promoter used as an internal control. The sequence of the mutated cis-elements was synthesized by Sangon Biotech. The effector plasmids and the reporter plasmids were co-transformed into protoplasts by polyethylene glycol (PEG)-mediated transformation. After culturing for 16–24 h at 25°C, the activities of LUC and REN were separately determined using a Dual-Luciferase Reporter Assay System (Promega, USA).
VIGS
Two specific cDNA fragments of TaCht20.2 and one specific fragment of TaGST1 with NotI and PacI restriction sites were obtained by RT-PCR for VIGS analysis as previously described (Holzberg et al., 2002). We confirmed that the fragments showed nonsimilarity with any other genes by BLAST (https://urgi.versailles.inra.fr/blast/) and siFi21 analysis of the wheat genome databases. The capped in vitro transcripts of BSMV-RNA were prepared from linearized plasmids containing the tripartite BSMV genome using the Message T7 in vitro transcription kit (Ambion, Austin, TX, USA), following the manufacturer’s instructions. VIGS assays were performed according to a previous study (Liu et al., 2018).
Analysis of total chitinase activities
The second leaf of T3 generation TaBZR2-OE and T2 generation TaBZR2-RNAi wheat plants were inoculated with urediospores of CYR23 and CYR31. These leaves were collected at 0 and 48 hpi to measure total chitinase activities, which were assayed with a commercial kit (Solarbio, BC0820) following the manufacturer’s guidelines.
Accession numbers
Sequence data from this article can be found in the Ensembl Plants (https://plants.ensembl.org/index.html) under accession numbers: TaBZR2 (TraesCS3A02G139000, TraesCS3B02G156600, TraesCS3D02G139300), TaCht20.2 (TraesCS5B02G403700), TaGST1 (TraesCS3A02G488200, TraesCS3B02G536100, TraesCS3D02G483000). The sequencing data from this study have been submitted to the CNSA (https://db.cngb.org/cnsa/) of CNGBdb with accession code CNP0001524.
Supplemental data
The following materials are available in the online version of this article.
Supplemental Figure S1. The transcript levels of brassinazole-resistant genes (TaBZRs) in compatible and incompatible interaction between wheat and Pst.
Supplemental Figure S2. Transcript profiles of TaBZR1 in wheat leaves in response to Pst infection.
Supplemental Figure S3. Molecular analysis of the brassinazole-resistant 2-overexpression (TaBZR2-OE) transgenic wheat plants.
Supplemental Figure S4. Relative transcript levels of pathogenesis-related genes (TaPR1, TaPR2) and catalase 3 gene (TaCAT3) in TaBZR2-OE and TaBZR2-RNAi lines (TaBZR2-Ri3/Ri7) inoculated with Pst.
Supplemental Figure S5. Growth of CYR31 race was decreased in TaBZR2-OE plants.
Supplemental Figure S6. Observations of H2O2 accumulation in TaBZR2-OE plants that were inoculated with CYR23 race.
Supplemental Figure S7. Molecular analysis of the TaBZR2-RNAi transgenic wheat plants (TaBZR2-Ri3/Ri7).
Supplemental Figure S8. Growth of CYR23 race was increased in TaBZR2-RNAi plants.
Supplemental Figure S9. Observations of H2O2 accumulation in TaBZR2-RNAi plants that were inoculated with CYR23 race.
Supplemental Figure S10. Functional categorization analysis of TaBZR2 target genes in molecular function during wheat–Pst infection.
Supplemental Figure S11. Phylogeny of TaCht20.2 and its orthologs.
Supplemental Figure S12. Relative transcript levels of (A) TaCht20.2-5B, (B) TaCht19-2A, (C) TaCht23.1-1A, (D) TaCht1.1-3B, (E) TaCht15.1-2A, (F) TaCht15.1-2B, and (G) TaCht23.2-5D in TaBZR2–OE plants inoculated with CYR23 and CYR31.
Supplemental Figure S13. Transcript profiles of TaCht20.2 in wheat leaves in response to Pst infection.
Supplemental Figure S14. Growth of CYR23 race was increased in TaCht20.2-knockdown plants.
Supplemental Figure S15. Observations of H2O2 accumulation in TaCht20.2-knockdown plants that were inoculated with CYR23 race.
Supplemental Figure S16. Relative transcript levels of TaCht20.2 in TaBZR2-OE and TaBZR2-RNAi lines inoculated with (A) Chinese yellow rust 20 (CYR20), (B) CYR22, (C) CYR25, (D) CYR29, (E) CYR32, (F) CYR33 and (G) CYR34.
Supplemental Figure S17. TaGST1 in wheat leaves in response to Pst infection.
Supplemental Figure S18. Silencing of TaGST1 has no obvious phenotypic effect during incompatible (CYR23) and compatible (CYR31) interactions.
Supplemental Figure S19. Relative transcript levels of TaGST1 in TaBZR2-OE and TaBZR2-RNAi plants inoculated with CYR23 and CYR31.
Supplemental Table S1. FPKM of all the TaBZRs in RNA-seq data.
Supplemental Table S2. Detailed information of TaCht20.2 and its putative orthologs.
Supplemental Table S3. FPKM of seven TaChts in RNA-seq data.
Supplemental Table S4. Primers used in this study.
†These authors contributed equally (X.B., G.Z.).
J.G. and Z.X. designed experiments. X.B., G.Z., S.T., H.P., M.I., and F.G. performed the experiments. X.C. generated the transgenic wheat. X.B. did the phylogenetic analysis. Z.K. and Y.M. supervised the experiments. X.B., J.G., and Z.X. wrote the manuscript.
The author responsible for distribution of materials integral to the findings presented in this article in accordance with the policy described in the Instructions for Authors (https://dbpia.nl.go.kr/plphys/pages/general-instructions) is: Jun Guo ([email protected]).
Acknowledgment
We thank Professor Larry Dunkle from Purdue University, USA for critical reading of the manuscript.
Funding
This study was financially supported by the National Natural Science Foundation of China (31620103913 and 31972224), Key Research and Development Program of Shaanxi (2021ZDLNY01-01), National Key R&D Program of China (2018YFD0200402), Natural Science Basic Research Program of Shaanxi (2020JZ-13), and the 111 Project from the Ministry of Education of China (B07049).
Conflict of interest statement. The authors declare that they have no conflicts of interest.
References
Author notes
Senior author.