-
PDF
- Split View
-
Views
-
Cite
Cite
Deyong Ren, Yuchun Rao, Haiping Yu, Qiankun Xu, Yuanjiang Cui, Saisai Xia, Xiaoqi Yu, He Liu, Haitao Hu, Dawei Xue, Dali Zeng, Jiang Hu, Guangheng Zhang, Zhenyu Gao, Li Zhu, Qiang Zhang, Lan Shen, Longbiao Guo, Qian Qian, MORE FLORET1 Encodes a MYB Transcription Factor That Regulates Spikelet Development in Rice, Plant Physiology, Volume 184, Issue 1, September 2020, Pages 251–265, https://doi.org/10.1104/pp.20.00658
- Share Icon Share
Abstract
Rice (Oryza sativa) spikelets have a unique inflorescence structure, and the mechanisms regulating their development are not yet fully understood. Moreover, approaches to manipulate spikelet development have the potential to increase grain yield. In this study, we identified and characterized a recessive spikelet mutant, namely more floret1 (mof1). The mof1 mutant has a delayed transition from the spikelet to the floral meristem, inducing the formation of extra lemma-like and palea-like organs. In addition, the main body of the palea was reduced, and the sterile lemma was enlarged and partially acquired hull (lemma and/or palea) identity. We used map-based cloning to identify the MOF1 locus and confirmed our identification by complementation and by generating new mof1 alleles using CRISPR-Cas9 gene editing. MOF1 encodes a MYB domain protein with the typical ethylene response factor-associated amphiphilic repression motifs, is expressed in all organs and tissues, and has a strong repression effect. MOF1 localizes to the nucleus and interacts with TOPLESS-RELATED PROTEINs to possibly repress the expression of downstream target genes. Taken together, our results reveal that MOF1 plays an important role in the regulation of organ identity and spikelet determinacy in rice.
Genetic changes in plant height and tiller number produced remarkable increases in grain yield during the Green Revolution. Altering inflorescence morphology is another target to produce further increases in yield. For example, rice (Oryza sativa) inflorescence has a unique structure among grain crops, with a panicle composed of determinate spikelets that each produces one floret and thus one grain. By contrast, wheat (Triticum aestivum) spikelets produce more than one floret, an observation suggesting that altering rice spikelet determinacy could improve grain yield (Ren et al., 2020). However, our understanding of rice inflorescence development and the mechanisms that specify spikelet structure and determinacy needs to be improved to enable such approaches.
In plants, the MYB gene family encodes a large family of transcription factors that bind MYB cis-acting elements and exist in almost all plants; several MYB transcription factors participate in floral development (Dubos et al., 2010). For example, Arabidopsis (Arabidopsis thaliana) AtMYB26 determines anther dehiscence, and the atmyb26 insertion mutant causes male sterility (Steiner-Lange et al., 2003). AtMYB103 regulates pollen and tapetal development in addition to trichome formation (Higginson et al., 2003). Mutation of AtMYB32 produces partially male-sterile flowers, probably due to disruption of the phenylpropanoid pathway (Preston et al., 2004).
AtMYB24 is mainly expressed in flowers and regulates anther formation. Overexpression of AtMYB24 leads to plant dwarfing and abnormal flower development, in particular the production of abnormal pollen grains and undivided anthers (Yang et al., 2007). Ectopic expression of AtMYB21 in the constitutive photomorphogenic1 (cop1) mutant of Arabidopsis causes abnormal carpel, narrowed petals and leaves, and shorter stems, suggesting that AtMYB21 plays an important role in petal and carpel development (Shin et al., 2002). AtMYB80 affects pollen development by regulating programed cell death in the tapetum (Phan et al., 2011).
In rice, MYB transcription factors also play an important role in floral development (Smita et al., 2015). For example, OsGAMYB is required for the development of floral organs and pollen. After gibberellic acid treatment, the osgamyb insertion mutant showed defective floral organs and pollen. OsGAMYB is mainly expressed in the aleurone layer, inflorescence apex region, stamen, and anther tapetum cells (Kaneko et al., 2004). ANTHER INDEHISCENCE1 (AID1) is expressed in leaves and panicles, and its loss of function induces pollen defects, reduced tillering, and delayed flowering (Zhu et al., 2004). OsMYB103 regulates anther development, and corresponding RNA-interference plants have defective tapetum and microspores (Zhang et al., 2010a). CARBON STARVED ANTHER (CSA) is associated with pollen and seed development, and the csa mutant exhibits a decrease in sugar content in floral organs, causing male sterility (Zhang et al., 2010b; Zhu et al., 2015).
Most of the above-mentioned genes belong to the class MYB-R2R3, and only a few MYB-1R genes have been reported. For example, CIRCADIAN CLOCK ASSOCIATED1 (CCA1)-like genes play an important role in maintaining the circadian rhythm of plants and mutation of CCA1 in Arabidopsis results in delayed flowering (Wang and Tobin, 1998). CAPRICE (CPC)-like genes are closely related to cell morphogenesis, and CPC is involved in regulating cell differentiation of root hairs (Wang and Tobin, 1998). In rice, SHALLOT-LIKE1 (SLL1) affects leaf shape by regulating programmed cell death in the abaxial surface sclerenchyma cells of leaves. The sll1 mutants fail to form sclerenchyma cells on the abaxial surface of the leaf and produce extremely inward curled leaves (Zhang et al., 2009). Recently, ABNORMAL HULL2 (AH2, another name for SLL1) was shown to determine hull fate and affect grain yield and quality, and the ah2 mutant produces degraded paleae, defective epidermal cells of the hull, and small grains (Ren et al., 2019a).
In this study, we identified the more floret 1 (mof1) mutant that exhibits abnormal spikelet morphology. The mof1 mutant produced an extra lemma and palea-like organ, suggesting that the transformation from the spikelet meristem to the floral meristem was delayed. In addition, the main body of the palea (mbp) was degraded and the sterile lemma was partially converted to the lemma or mbp-like organ with the fused identity of the sterile lemma and lemma/mbp. MOF1 encodes a nuclear-localized MYB domain protein with transcriptional repression activity that MOF1 interacts with TOPLESS-RELATED PROTEINs (OsTPRs) to possibly repress the expression of downstream target genes. These results indicate that MOF1 plays an important role in the regulation of organ identity and spikelet determinacy. Moreover, the mof1 mutant provides broader insight into the role of MYB transcription factors in spikelet development. The findings in this study reveal that the presence of two or more florets is possible in a single rice spikelet, which will lay the foundation for increasing the grain number per spikelet.
RESULTS
Phenotypic Characterization of the mof1 Mutant
In the wild type, the spikelet consists of two external glume-like organs (a pair of rudimentary glumes and a pair of sterile lemmas) and one terminal floret (Fig. 1, A and B). The floret has two external organs (a lemma and a palea) and three sets of internal organs: two lodicules (whorl 2 organs) adjacent to the lemma margins, six stamens (whorl 3), and one pistil with two stigmas (whorl 4; Fig. 1, A–E). The lemma and palea are much larger than the sterile lemmas, and the lemma is slightly wider than the palea (Fig. 1, A, B, and E). The palea has two parts: two marginal transparent regions of the palea (mtrp) and the mbp (Fig. 1, B, C, and E). The lemma and mbp have a similar silicified adaxial epidermis with trichomes and protrusions, and the mtrp has a nonsilicified smooth adaxial epidermis lacking trichomes and protrusions (Fig. 1, C and E). The lemma and palea have five and three vascular bundles, respectively, whereas the sterile lemma has a smooth outer epidermis and only one vascular bundle (Figs. 1, D and E, and 2, B–D).

Phenotypes of spikelets in the wild type and the mof1 mutant. A and B, Spikelet of the wild type (WT). C, Epidermal surface of the wild-type palea. D, Epidermal surface of the sterile lemma in the wild type. E, Histological observations of florets in the wild type. F to J, Group I mof1 spikelets. F and G, mof1 spikelet with an extra lemma-like organ and palea-like organs. H, Epidermal surface of palea-like organs in G. I, Epidermal surface of the sterile lemma in the mof1 spikelet with an extra lemma-like organ and palea-like organs. J, Histological observations of the mof1 spikelet with an extra lemma-like organ and palea-like organs. K to O, Group II mof1 spikelets. K and L, mof1 spikelet with degraded paleae. M, Epidermal surface of degraded paleae in the mof1 spikelet. N, Epidermal surface of the sterile lemma in the mof1 spikelet with degraded paleae. O, Histological observations of the mof1 spikelet with degraded paleae. P, Structural diagram of wild-type and mof1 spikelets. Q, Percent of spikelets in the wild type and mof1 mutant. ASL, all mof1 florets with abnormal sterile lemmas; DP, mof1 florets with a degraded palea (group II spikelets); dp, degraded palea; ell, extra lemma-like organ; isl, inner sterile lemma; le, lemma; lo, lodicule; OASL, mof1 florets with only abnormal sterile lemmas; osl, outer sterile lemma; pa, palea; pal, palea-like organ; pi, pistil; st, stamen; TLP, mof1 florets with two paleae and lemmas (group I spikelets). Red stars represent vascular bundles. Red arrows represent vascular bundles. Red braces indicate the mbp. Bars = 1 mm (A–C, F, G, L, M, and K) and 100 µm (D, E, I, J, N, and O).
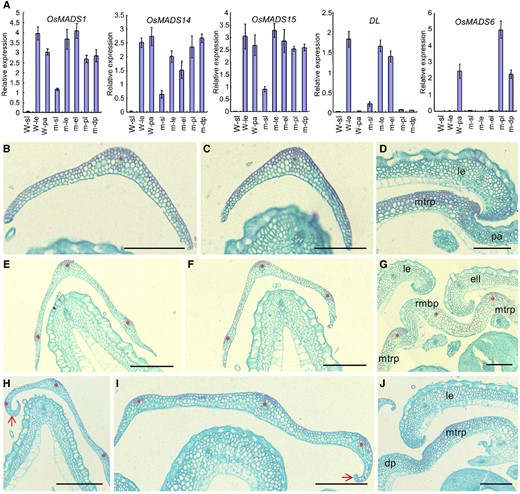
Histological observations and expression analysis of floral identity genes in lateral organs of the wild type (W) and the mof1 mutant. A, Expression analysis of OsMADS1, OsMADS6, OsMADS14, OsMADS15, and DL in lateral organs of the wild type and the mof1 mutant. Lateral organs, the sterile lemma in the wild type, the lemma in the wild type; the palea in the wild type, the sterile lemma in the mof1 mutant, the lemma in the mof1 mutant, the degraded palea in the mof1 mutant, the extra lemma in the mof1 mutant, and the palea-like organ in the mof1 mutant. RNA was isolated from lateral organs of the wild type and mof1 spikelets. Error bars indicate sd. At least three replicates were performed, from which the mean value was used to represent the expression level. B, Transverse section of the outer sterile lemma in the wild type. C, Transverse section of the inner sterile lemma in the wild type. D, Partial transverse section of the wild-type floret. E to G, Group I mof1 spikelets. E, Transverse section of the outer sterile lemma in the mof1 floret with an extra lemma-like organ and palea-like organs. F, Transverse section of the inner sterile lemma in the mof1 floret with an extra lemma-like organ and palea-like organs. G, Partial transverse section in the mof1 floret with an extra lemma-like organ and palea-like organs. H to J, Group II mof1 spikelets. H, Transverse section of the outer sterile lemma in the mof1 floret with degraded paleae. I, Transverse section of the inner sterile lemma in the mof1 floret with degraded paleae. J, Partial transverse section in the mof1 floret with degraded paleae. dp, Degraded palea; ell, extra lemma-like organ; isl, inner sterile lemma; le, lemma; M, mof1 mutant osl, outer sterile lemma; pa, palea; pal, palea-like organ. Red stars represent vascular bundles. Red arrows indicate the inner hook structure, which is similar to that of the wild-type lemma. Bars = 100 µm. Data are means ± sd (n = 3).
In the vegetative stage, the mof1 mutant showed no defects and resembled the wild-type plants. By contrast, at the heading stage, mof1 spikelets showed severe, pleiotropic defects (Fig. 1, F–O). We categorized these defects into two groups based on the presence of two lemmas and two palea-like organs (group I; Fig. 1, P and Q) and a degraded palea (group II; Fig. 1Q). The group I mof1 spikelets developed an extra lemma-like organ and two palea-like organs in the position occupied by the normal palea (Fig. 1, F–H). Interestingly, each palea-like organ bore a reduced mbp and two mtrps with three vascular bundles, which resembled that of the wild-type palea (Fig. 1, H–J). The mbp and two marginal transparent regions of the palea (mtrps) exhibited a similar texture with that of the wild-type palea (Figs. 1, H–J, and 2G).
In group II mof1 spikelets, the palea width was reduced to various degrees (Fig. 1, K–O). Further investigation showed that the mbp was degraded, but the mtrp retained its normal size (Figs. 1, M–O, and 2I). In a few cases, mof1 spikelets lacked the palea; instead, two mrp-like structures were observed in the corresponding position. The mrp-like structures had a smooth, nonsilicified epidermis without protrusions and trichomes, which were similar to those of the wild-type palea (Figs. 1, N and O, and 2J).
We next investigated the expression levels of the organ identity/marker genes, LEAFY HULL STERILE1/OsMADS1 (LHS1/OsMADS1), OsMADS14, OsMADS15, MOSAIC FLORAL ORGANS1/OsMADS6 (MFO1/OsMADS6), and DROOPING LEAF (DL) in the extra and defective organs of mof1 spikelets (Ohmori et al., 2009; Ren et al., 2013). In group I mof1 spikelets, abundant OsMADS14, OsMADS15, and LHS1/OsMADS1 transcripts were detected in the original lemmas, the extra lemma-like organs, and the palea-like organs (Fig. 2A). DL expression was found in the original lemmas and extra lemma-like organs of group I mof1 spikelets, whereas MFO1/OsMADS6 expression was not detected (Fig. 2A). By contrast, the DL signal was not detected, and increased MFO1/OsMADS6 expression was observed in the palea-like organs of group-I mof1 spikelets (Fig. 2A). However, MFO1/OsMADS6 expression was not affected in the reduced paleae of group II mof1 spikelets (Fig. 2A).
These results suggest that the extra lemma-like organ acquired the normal lemma identity in group I mof1 spikelets, and each reduced palea-like organ of group I mof1 spikelets and the reduced palea of group II mof1 spikelets retained the normal mtrp identity. However, the increased transcripts of MFO1/OsMADS6 may result from the relative abundance of mtrp structures in the palea-like organs of group I mof1 spikelets. These findings reveal that the development of the mbp is disturbed in mof1 spikelets, but the mtrp develops normally.
Further, a closeup view showed that the sterile lemma of most mof1 spikelets had three virescent lines (i.e. vascular bundles), and its upper surface was rougher than that of the wild type (Figs. 1, D, E, I, J, L, and N–Q, and 2. B, C, E, F, H, and I). The upper epidermis of the sterile lemma bore a few trichomes on the abaxial side in the wild type, whereas the sterile lemma bore more trichomes on the whole upper epidermis than that of the mof1 mutant (Fig. 1, D, I and N). Also, the lengths and widths of the sterile lemmas were distinctly increased in the mof1 spikelets (Supplemental Fig. S1E). Upon closer inspection, more vascular bundles and wider sterile lemmas were observed in group I and group II mof1 spikelets (Figs. 1, I, J, L, and N–Q, and 2, B, C, E, F, H, and I). These abnormal sterile lemmas showed inner hook structures, resembling that of the wild-type lemma (Figs. 1, I, J, L, and N–Q, and 2). In the mof1 sterile lemmas, OsMADS14, OsMADS15, LHS1/OsMADS1, and DL were ectopically expressed; however, DL signals were weak (Fig. 2A). These results suggest that the mof1 sterile lemmas acquire partial hull (i.e. lemma and/or palea) identity.
Next, we investigated the floral organs of the inner three whorls in the wild type and mof1 mutant. In the florets with extra lemmas and paleae, some florets induced more lodicules, stamens, pistils, and the remaining floral meristem, but their identities were not changed (Supplemental Fig. S1, A, C, D, and F). In the florets with a degenerated palea, the stamen number of some florets was reduced, but it retained its identity (Supplemental Fig. S1, B and F).
Abnormal Early Spikelet Development in the mof1 Mutant
To investigate the abnormal development of mof1 spikelets in detail, we monitored early spikelets using scanning electron microscopy. At spikelet stage 4 (Sp4, formation of the lemma and palea primordia), the wild-type spikelets developed the lemma and palea primordia, whereas some mof1 florets (group I mof1 florets) produced extra lemma-like organ primordia, two smaller palea primordia, and enlarged floral meristems (Fig. 3, A and E). Other florets (group II mof1 florets) initiated reduced palea primordia (Fig. 3I). During stages Sp5 to Sp6 (formation of the lodicule and stamen primordia), the wild-type florets initiated six stamen primordia, whereas the stamen number was varied, and the defective palea primordia continuously grew in the mof1 florets bearing extra lemma and palea-like organs or a reduced palea (Fig. 3, B, F, and J). At stages Sp7 to Sp8 (formation of the pistil and ovule), the pistil primordia formed, and the lemma and palea further developed in the wild type (Fig. 3, C and D). By contrast, obvious extra lemma-like and palea-like organs were found in the mof1 florets with extra lemmas and/or defective paleae (Fig. 3, G, H, K, and L). We next investigated the sterile lemma at stages Sp4 to Sp8. No obvious defects were found at stage Sp4 in the wild type and mof1 mutant (Fig. 3, A and I). However, the mof1 spikelets developed longer sterile lemmas compared with those of the wild type during stages Sp5 to Sp8 (Fig. 3, B–D and J–L). These results indicate that the development of the sterile lemma was disturbed until Sp5 in the mof1 mutant, and were consistent with the observed defects in spikelets at the heading stage.
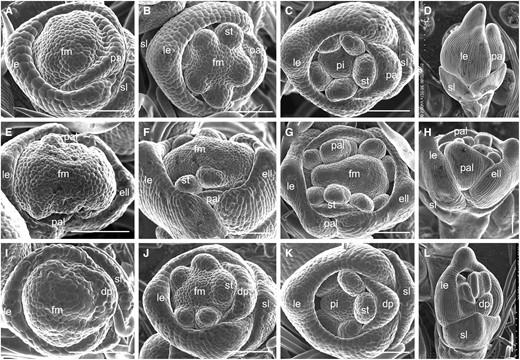
Early spikelets in the wild type and the mof1 mutant. A to D, Wild-type spikelets. A, Sp4. B, Sp5 to Sp6. C, Sp7. D, Sp8. E to H, Group I mof1 spikelet with an extra lemma-like organ and palea-like organs. E, Sp4. F, Sp5 to Sp6. G, Sp7. H, Sp8. I to L, Group II mof1 spikelet with degraded paleae. I, Sp4. J, Sp5 to Sp6. K, Sp7. L, Sp8. dp, Degraded; ell, extra lemma-like organ; le, lemma; pa, palea; palea; pal, palea-like organ; pi, pistil; st, stamen; sl, sterile lemma. Bars = 100 µm.
Molecular Cloning of MOF1
To determine the causal gene for the mof1 mutation, we performed reciprocal crosses between the mof1 mutant and japonica cultivar, Wuyunjing 7 (cv W7). All F1 plants from both cross combinations exhibited a normal phenotype, similar to that of the wild type. In the F2 progeny, the ratio of the wild-type plants to mutant plants was 3:1, fitting the Mendelian segregation ratio of a single, recessive gene. These results imply that the mof1 phenotype is controlled by a single, recessive, nuclear gene.
Twenty plants showing the mof1 phenotype were chosen from the F2 population of a cross between cv W7 and the mof1 mutant, and the MOF1 locus was preliminarily located between the simple sequence repeat markers M1 and M8 on the long arm of chromosome 4 (Fig. 4A). By fine mapping, we further mapped the MOF1 locus to a 60-kb physical interval using the remaining 3802 recessive plants and 10 polymorphic markers (Fig. 4A). By sequencing the open reading frames of the eight predicted genes within this region (http://www.ricedata.cn/), we found that the first open reading frame (LOC_Os04g47890), encoding a MYB transcription factor, differed between the wild type and the mof1 mutant. The LOC_Os04g47890 gene contains five introns and six exons and encodes a protein with 307 amino acids. We found a single-base C deletion in the fourth exon, which is predicted to produce a truncated protein due to a coding frame shift and a premature translation stop (Fig. 4A).
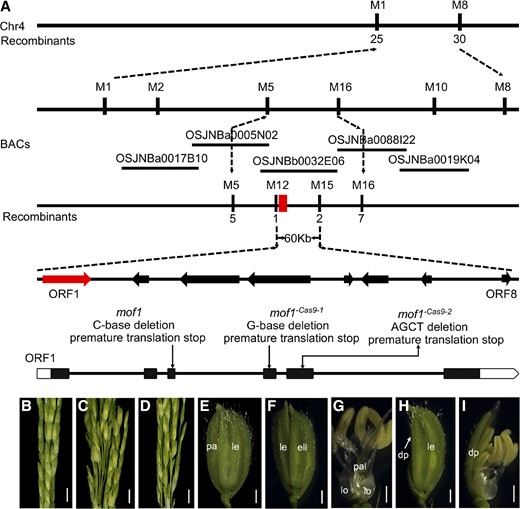
Map-based cloning of MOF1. A, Map position of the MOF1 locus. B, Wild type. C, mof1 mutant. D, Transgenic complementation plants rescue the defective spikelets of the mof1 mutant. E, cv ZH11 spikelet. F to I, Spikelet of the CRISPR/Cas9 transgenic plant. F and G, mof1 Cas9-1 spikelet with an extra lemma-like organ and palea-like organs. H and I, mof1 Cas9-1 spikelet with degraded paleae. dp, Degraded palea; ell, extra lemma-like organ; le, lemma; lo, lodicule; pa, palea; pal, palea-like organ. Bars = 1 cm (B–D) and 1 mm (E–I).
To test whether the mutation of LOC_Os04g47890 caused the mutant phenotype, we performed a complementation assay by transforming a 6.7-kb genomic DNA fragment from the wild type covering the entire LOC_Os04g47890 sequence into the mof1 mutant. Fifty-two transgenic plants were generated from the transformation, among which 11 individuals had the transgene; these individuals showed the wild-type phenotype, suggesting that the mof1 mutant phenotypes in these transgenic plants were completely rescued (Fig. 4, B–D).
Furthermore, we used CRISPR-Cas9 gene editing to knock out LOC_Os04g47890 in plants of the japonica cultivar Zhonghua 11 (cv ZH11). Two mutants (named mof1 −Cas9-1 and mof1 −Cas9-2) had a single-base G deletion in the third exon and four-base AGCT deletion in the second exon, respectively, resulting in a frame shift, thereby forming a truncated protein, and the plants produced similar phenotypes to those of the mof1 mutant (Fig. 4, E–I; Supplemental Fig. S1, G and H). Taken together, these results confirm that LOC_Os04g47890 is the MOF1 gene, which is responsible for the phenotype defects in the mof1 mutant.
MOF1 Encodes a MYB Transcription Factor
A phylogenetic analysis revealed that MOF1 and its orthologs from various dicot and grass species formed a MOF1-like clade, with rice MOF1 belonging to the grass subclade (Fig. 5A). A protein sequence analysis showed that most MOF1-like proteins had a highly conserved MYB domain and two ethylene response factor-associated amphiphilic repression (EAR) motifs belonging to the LxLxL subclass. The EAR motifs were located at the N terminus and C terminus of all the MOF1-like proteins (Supplemental Fig. S2). MOF1-like proteins from grasses also contained another highly conserved domain, with several unique motifs and conserved amino acids (Supplemental Fig. S3). These results implied that the MOF1-like proteins from grasses may have different evolutionary functions to those in dicots. Our phylogenetic analysis also showed that the known MYB domain gene SLL1/AH2 has a distant evolutionary relationship with the MOF1-like genes (Fig. 5A).
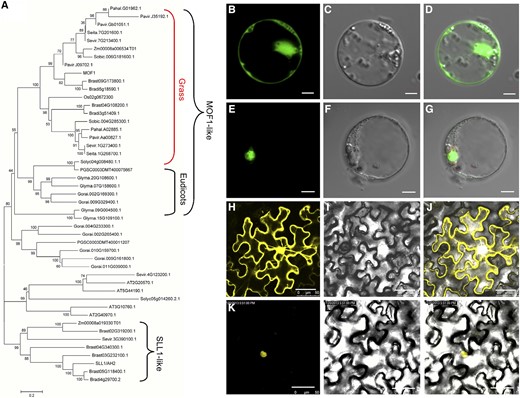
Phylogenetic tree and subcellular localization of the MOF1 protein. A, Phylogenetic tree analysis. The phylogenetic tree was performed using the neighbor-joining method, and bootstrap support values calculated from 1000 replicates are given at the branch nodes. At, Arabidopsis thaliana; Bradi, Brachypodium distachyon; Brast, Brachypodium stacei; Glyma, Glycine max; Gorai, Gossypium raimondii; Pavir, Panicum virgatum; Pahal, Panicum hallii; PGSC, Solanum tuberosum; Seita, Setaria italica; Sevir, Setaria viridis; Sobic, Sorghum bicolor; Solyc, Solanum lycopersicum; Zm, Zea mays. B to D, Single GFP protein in rice protoplasts. E to G, MOF1-GFP in rice protoplasts. H to J, Single YFP protein in tobacco (Nicotiana tabacum). K to M, MOF1-YFP in tobacco. Bars = 5 µm (B–G) and 50 µm (H–M).
Next, we investigated the subcellular localization of MOF1. Two plasmids containing the full-length MOF1 protein sequence fused to yellow fluorescent protein (YFP) or GFP were transiently expressed in tobacco epidermal cells and rice protoplasts. In cells expressing YFP or GFP only, the yellow or green fluorescent signals were observed in the whole cell, whereas the yellow or green fluorescence was exclusively visible in the nuclei of tobacco epidermal cells or rice protoplasts expressing MOF1-YFP or MOF1-GFP (Fig. 5, B–M). These results imply that MOF1 encodes a nuclear protein, possibly functioning as a transcription regulator, consistent with the well-known MYB proteins in rice and Arabidopsis.
Expression Patterns of MOF1
We examined the temporal and spatial expression of MOF1 to investigate how it functions at the molecular level. First, reverse transcription quantitative PCR (RT-qPCR) analysis showed that MOF1 was expressed in all organs and tissues including the root, leaf, leaf sheath, culm, panicles of various developmental stages, sterile lemma, lemma, palea, lodicule, stamen, and pistil. Notably, MOF1 transcripts were more abundant in the lemma, palea, and young panicles (less than 0.5 cm long) relative to other tissues or floral organs (Fig. 6A).
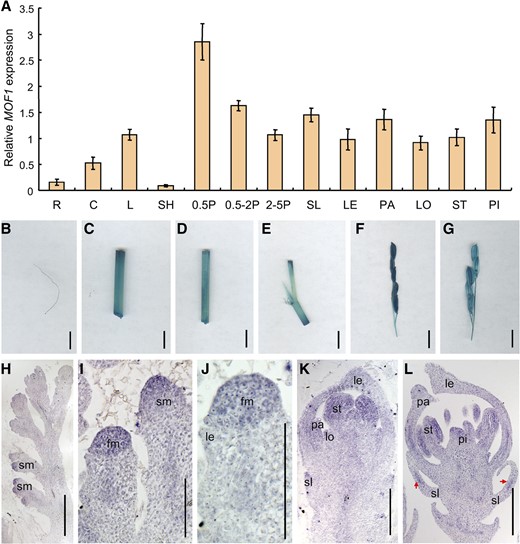
Expression pattern of MOF1. A, MOF1 expression in different tissues and organs as detected by RT-qPCR. B, GUS staining of root. C, GUS staining of culm. D, GUS staining of leaf. E, GUS staining of leaf sheath. F and G, GUS staining of panicles in different developmental stages. H to L, In situ hybridization in panicles and flowers of the wild type using a MOF1 antisense probe. R, Root; C, culm; L, leaf; SH, sheath; 0.5P, 0.5-cm panicle; 0.5-2P, 0.5 to 2 cm panicle; 2-5P, 2- to 5-cm panicle. fm, Floral meristem; le and LE, lemma; lo and LO, lodicule; pa and PA, palea; pi and PI, pistil; sl and SL, sterile lemma; sm, spikelet meristem; st and ST, stamen. Red arrows indicate the signals. Bars = 1 cm (B–G) and 50 µm (H–L). Data are means ± sd (n = 3).
Second, the MOF1 promoter-β-glucuronidase (GUS) vector (pMOF1-GUS) was constructed and transformed into cv ZH11 calli (japonica cultivar). GUS signals were detected in the root, leaf, leaf sheath, culm, and panicles, consistent with the RT-qPCR results (Fig. 6, B–G). Next, we further analyzed the expression of MOF1 in developing panicles and spikelets by in situ hybridization. Strong MOF1 signals were found in the spikelet meristem (Fig. 6, H and I). When the lemma and palea primordia were forming, MOF1 was highly expressed in the lateral organs (i.e. the sterile lemma, lemma, and palea) and the floral meristem (Fig. 6, J and K). Subsequently, MOF1 expression was primarily detected in all lateral organs, and the lodicule and stamen (Fig. 6, K and L). Notably, the MOF1 signals were localized to the middle region of the sterile lemma primordia, whereas it was uniformly expressed in the lemma and palea primordia (Fig. 6L).
The mof1 Mutation Altered the Expression of Organ Identity-Related Genes
To reveal the regulatory mechanism of MOF1, we detected the expression levels of organ identity genes (DL, OsMADS6, LHS1, LONG STERILE LEMMA [G1], and OsMADS2) during the early stages of floral/spikelet development. The RT-qPCR analysis showed that the expression levels of DL, OsMADS6, and OsMADS2 were up-regulated in the mof1 young panicles (Supplemental Fig. S3A). G1 transcripts showed a slight decrease in abundance, and no obvious alteration of LHS1 expression was observed in the mof1 young panicles (Supplemental Fig. S3A).
Furthermore, we investigated the expression of the floral organ identity genes DL, OsMADS6, and OsMADS2 by in situ hybridization. In the wild type, the DL signals were only found in the lemma primordia during stages Sp4 to Sp6 (Fig. 7, A and B). Following this, DL was expressed in the midrib of the lemma and the pistil during stages Sp7 and Sp8 (Fig. 7, C and D). In the mof1 florets bearing an extra lemma-like organ and two palea-like organs (group I mof1 florets), the DL signals were visible in the extra lemma-like organ, the original lemma, and the pistil (Fig. 7, E–H). In the mof1 florets with a degraded palea (group II mof1 florets), the DL expression pattern was similar to that in the wild type (Fig. 7, I–L). During stages Sp4 to Sp7, OsMADS6 expression was detected in the floral meristem, mrp, lodicule, and pistil, and no obvious differences were detected in the wild type and the mof1 mutant (Fig. 8, A–C, E–G, and I–K). Until Sp8, in group I mof1 florets, OsMADS6 signals were found in the four mtrps of the two palea-like organs (Fig. 8, D and H). However, OsMADS6 transcripts were only detected in the two mtrps in wild-type and group II mof1 florets (Fig. 8, D and L). Signals for OsMADS2 were found in two lodicules in the wild type, whereas its signals were visible in four lodicules at stages Sp7 and Sp8 (Supplemental Fig. S3, B–E). These results indicate that the group I mof1 florets develop four lodicules, an extra lemma, and two reduced palea-like organs, which are derived from paleae. All abnormal paleae retain the normal mtrp identity in the mof1 florets.
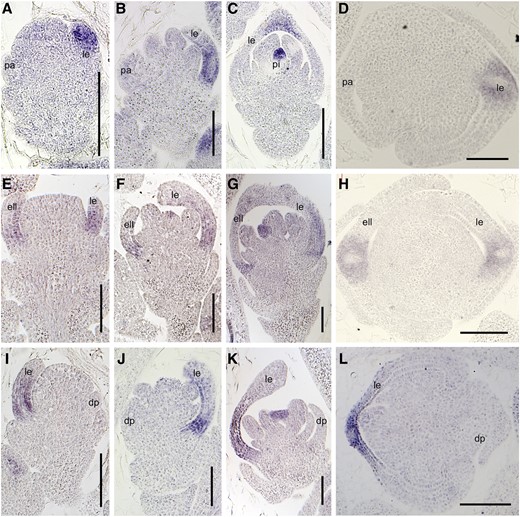
Expression of DL in the wild type and the mof1 mutant. A to D, Wild-type spikelet. A, Sp4; B, Sp5 to Sp6; C, Sp7; D, Sp8. E to H, Group I mof1 spikelet with an extra lemma-like organ and two reduced paleae. E, Sp4; F, Sp5 to Sp6; G, Sp7; H, Sp8. I to L, Group II mof1 spikelet with a reduced palea. I, Sp4; J, Sp5 to Sp6; K, Sp7; L, Sp8. ell, Extra lemma-like organ; esl, elongated sterile lemma; le, lemma; pa, palea; pal, palea-like organ; sl, sterile lemma. Bars = 50 µm.
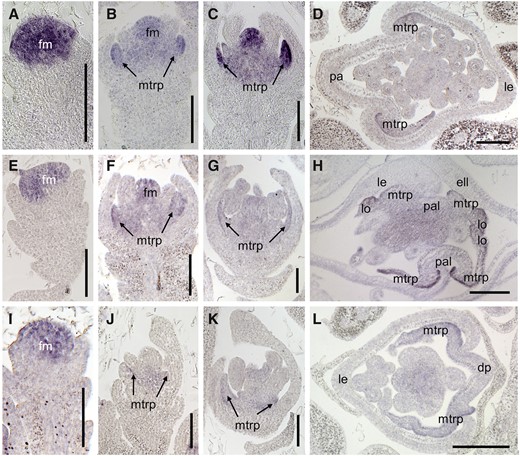
Expression of OsMADS6 in the wild type and the mof1 mutant. A to D, Wild-type spikelet. A, Sp4; B, Sp5 to Sp6; C, Sp7; D, Sp8. E to H, Group I mof1 spikelet with an extra lemma-like organ and two reduced paleae. E, Sp4; F, Sp5 to Sp6; G, Sp7; H, Sp8. I to L, Group II mof1 spikelet with a reduced palea. I, Sp4; J, Sp5 to Sp6; K, Sp7; L, Sp8. ell, Extra lemma-like organ; fm, floral meristem; le, lemma; lo, lodicule; pa, palea; pal, palea-like organ. Bars = 50 µm.
Analysis of MOF1 Transcriptional Regulation Activity
As MOF1 is predicted to encode a putative MYB transcription factor, we investigated its transcriptional regulation activity using yeast assays and a dual luciferase assay system in rice protoplasts. For the yeast assays, we fused MOF1 and two controls, namely the rice transcription factors FRIZZY PANICLE (FZP) and AH2, to the GAL4 DNA-binding domain (BD). To this end, we constructed the fused plasmids MOF1-BD, FZP-BD, and AH2-BD, which were then transformed into yeast cells. The empty pGBKT7 vector and the AH2-BD vector were used as negative controls, and the FZP-BD vector was used as a positive control (Ren et al., 2018a, 2019a). The yeast cells expressing FZP-BD could grow on medium without Trp, adenine, and His, whereas the yeast cells expressing MOF1-BD, AH2-BD, and pGBKT7 could not survive in the absence of Trp, adenine, and His (Fig. 9A). These results suggest that MOF1 does not have transcriptional activation activity and may function as a transcriptional repressor.
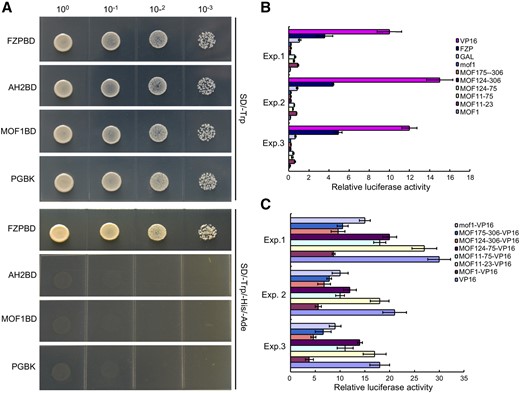
Transcriptional activity analysis of MOF1 protein. A, Transcriptional activation investigation of MOF1 in yeast cells. The positive control was pGBKT7-FZP (FZPBD), and the negative control was pGBKT7-AH2 (AH2BD). B and C, Relative luciferase activities in rice protoplasts cotransfected with reporter and different effectors. MOF11–23, MOF11–75, MOF124–75, MOF124–306, and MOF175–306 represent the truncated MOF1 proteins. B, All luciferase activities are expressed relative to the value of GAL4 BD alone. C, All luciferase activities are expressed relative to the value of VP16. Data are means ± sd (n = 3).
To further explore MOF1 transcriptional regulation activity, the coding region of MOF1, several truncated fragments, and the coding region of mof1 were fused to the GAL4 DNA-BD vector driven by the 35S promoter from Cauliflower mosaic virus. The FZP-GAL and VP16 vectors were used as positive controls, whereas the fused AH2-GAL and empty GAL vectors were used as negative controls. All fused vectors were transformed into rice protoplasts and transiently expressed. Compared with the empty GAL vector, the FZP-GAL and VP16 vectors showed higher transcriptional activity. By contrast, the full-length MOF1 and mof1 proteins and the truncated MOF1 proteins (MOF11–75, MOF124–75, MOF124–306, and MOF175–306) had a lower transcriptional activity than that of the empty GAL vector, the FZP-GAL vector, and the VP16 vector (Fig. 9B). However, the truncated MOF124–75 and MOF124–306 proteins showed decreased transcriptional activity compared with that of other MOF1 proteins and the mof1 protein (Fig. 9B). The transcriptional activity of the truncated MOF11–23 protein was similar to that of the empty GAL vector (Fig. 9B).
To explain the variable capacity of transcriptional activity in these truncated MOF1 proteins, we analyzed the protein motifs in full-length MOF1. Our analysis showed the presence of two predicted repression motifs, EAR1 and EAR2 (LxLxL), which were located in the N-terminal and C-terminal region of the MOF1 protein, respectively, whereas the EAR2 motif within the C-terminal of the mof1 protein was destroyed. Among the truncated proteins, MOF124–306 contained two EAR motifs (EAR1 and EAR2), MOF175–306 contained one EAR motif (EAR2), MOF11–75 and MOF124–75 each contained one EAR motif (EAR1), and MOF11–23 had no EAR motif. These analyses were consistent with the different transcriptional activities of the different MOF1 proteins and indicate that the EAR1 motif has a slight repressive effect, whereas the EAR2 motif has a strong repressive effect.
To further confirm the actual repression capacity, we inserted the full-length sequence of MOF1, its truncated versions, and the full-length sequence of mof1 into a vector in frame with the transcriptional activator VP16 and then expressed all the constructs in rice protoplasts. MOF1-VP16, MOF11–75-VP16, MOF124–75-VP16, MOF124–306-VP16, MOF175–306-VP16, and mof1-VP16 showed lower transcriptional activity than that of VP16, and MOF11–23-VP16 had similar transcriptional activity as that of VP16 (Fig. 9C). MOF1-VP16, MOF124–306-VP16, and MOF175–306-VP16 exhibited a lower transcriptional activity compared with MOF11–75-VP16, MOF124–75-VP16, and mof1-VP16 (Fig. 9C). These findings support the previous results and imply that the EAR2 motif in MOF1 has a stronger repression effect than that of the EAR1 motif. Taken together, our results reveal that MOF1 is an active transcriptional repressor and its suppression effect is mainly contributed by the EAR motifs.
MOF1 Interacts with the OsTPR Corepressors
Since MOF1 had the typical EAR motifs, we tested whether it interacts with the TPR corepressor proteins. Rice has three TPR proteins, namely OsTRP1/Os01g15020, OsTRP2/ABERRANT SPIKELET AND PANICLE1 (OsTRP2/ASP1), and OsTRP3/Os03g14980. To explore the interaction of MOF1 with the three rice TPR proteins, we first performed yeast two-hybrid assays. In these assays, MOF1 directly interacted with OsTRP1, OsTRP2, and OsTRP3 in yeast cells (Fig. 10A). Next, we carried out a pull-down test with the purified fusion proteins in vitro. The results showed that MOF1 can interact with the three rice TRP proteins (Fig. 10B). These findings suggest that MOF1 interacts with OsTPRs to possibly repress the expression of downstream target genes.
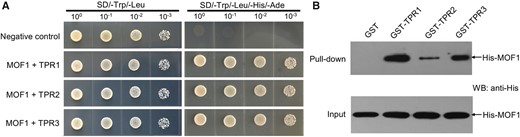
MOF1 interacts with OsTPRs. A, MOF1 interacts with OsTPR1, OsTPR2, and OsTPR3 by yeast two-hybrid test. B, Glutathione S-transferase (GST) pull-down experiments showing interactions of MOF1 with OsTPR1, OsTPR2, or OsTPR3. MOF1 was fused with His.
DISCUSSION
MOF1 Regulates Spikelet Meristem Determinacy
Our findings revealed that some mof1 spikelets produced two florets, and spikelet meristem determinacy was lost in the mof1 mutant. Similarly, other studies showed that some spikelets produced two florets or an extra lemma-like organ (secondary floret) in the tongari-boushi1 (tob1), multifloret spikelet1 (mfs1), supernumerary (snb), and empty glume1/double floret1 (eg1/df1) mutants, the snb osids1 double mutant, and OsMADS22-overexpressing plants (Sentoku et al., 2005; Lee et al., 2007; Li et al., 2009; Lee and An, 2012; Tanaka et al., 2012; Ren et al., 2013, 2018b). Additionally, the HD-ZIP III protein LATERAL FLORET1 (LF1) induces the initiation of lateral floral meristems by activating the expression of OSH1 (ORYZA SATIVA HOMEOBOX1/HOMEOBOX PROTEIN KNOTTED-1-LIKE6), and the mutation of LF1 results in one or two fertile lateral florets in addition to one terminal fertile floret (Zhang et al., 2017). A recent study reported that FLORAL ORGAN NUMBER4 (FON4) is involved in the regulation of spikelet determinacy (Ren et al., 2019b). In the fon4-7 mutant, some spikelets developed two florets in which two lemmas, two reduced paleae, and four lodicules were also observed, similar to the mof1 phenotype. Also, other fon4-7 spikelets generated a secondary floret bearing only a lemma. These findings suggest that MOF1, together with FON4, LF1, TOB1, MFS1, SNB, OsIDS1, and OsMADS22, affect the timing of the transition from the spikelet meristem to the terminal floral meristem, and their mutations or ectopic expression induce spikelet meristem indeterminacy. These genes appear to function at different times in the specification of meristem determinacy, as the mof1, fon4, lf1, tob1, and mfs1 mutants and OsMADS22-overexpressing plants lost spikelet meristem determinacy after the formation of sterile lemmas, whereas the snb and osids1 mutants lost spikelet meristem determinacy before the presence of rudimentary glumes, suggesting that MOF1, FON4, LF1, TOB1, MFS1, and OsMADS22 function later than SNB and OsIDS1.
Spikelet meristem determinacy has implications for grain yield. In wheat and oat (Avena sativa), the spikelet meristem exhibits indeterminacy and develops multiple florets, forming more than one seed in a single spikelet (Ren et al., 2020). The findings in this and previous studies reveal that the presence of two or more florets is possible in a single rice spikelet, which will lay the foundation for increasing the floret/grain number per spikelet.
MOF1 Specifies Palea Identity
The lemma and palea are unique grass structures, and their identity and origin are controversial. The lemma is often proposed to be a bract-like organ, and the palea is generally considered to be a prophyll. Previous studies showed that the lemma and palea may be first-whorl floral organs in grass (Xiao et al., 2003; Preston et al., 2009; Zheng et al., 2015). However, increasing evidence has indicated that the rice palea is not equivalent to the lemma as the first-whorl floral organ, and the mtrp is the homologous organ of the sepal, whereas the mbp is not. First, the palea has three vascular bundles and two components: the mbp and mtrp, whereas the lemma possesses five vascular bundles and is larger than the palea. And the cellular characteristics of the mbp resemble that of the lemma but are different from that of the mtrp. Second, the mutants of Arabidopsis B-class genes failed to produce sepals and instead produced petals, which were equivalent to lodicules. Third, the mbp of the palea was incurved and reduced, and the mtrp was converted to the ovary-like organ in the deformed floral organ 1/curved chimeric palea1 (dfo1/ccp1) mutant and OsMADS58 overexpression plants, whereas the lemma largely retained its original identity (Zheng et al., 2015). Moreover, the rice B-class mutant osmads16 and OsMADS2 OsMADS4 double RNA-interference plants displayed a transformation of the lodicules into the mtrp-like organs but not the mbp-like organs. These results reveal that the mtrp is homologous to the sepal but not the mbp and support the notion that the lemma and palea are not from the same whorl of floral organs in rice.
In addition, the mtrp is converted to the mbp or lemma-like structures in the mfo1 and chimeric floral organ1 (cfo1) mutants, whereas in the ah2, fon4-7, retarded palea1 (rep1), depressed palea1 (dp1), mfs1, and degenerative palea (dep) mutants, the mbp is reduced or lost, causing the palea to be replaced by two mtrp-like organs (Ohmori et al., 2009; Yuan et al., 2009; Wang et al., 2010; Jin et al., 2011; Ren et al., 2013, 2019a, 2019b). Intriguingly, in our mof1 mutant, in those spikelets with two lemmas and two palea-like organs, the mbp was degenerated. However, the abnormal bop exhibited similar outer cellular structures, which resembled that of the lemma, and the mtrp retained its size and identity. Together with the results of other studies, our phenotypic observations of the mof1 palea imply that the rice palea is a congenital fusion of the mbp and the mtrp, which have different identities and origins. These findings suggest that the mbp and mtrp are mainly regulated by two regulatory pathways. MOF1, AH2, FON4, DP1, REP1, MFS1, and DEP control the mbp identity, whereas MFO1 and CFO1 determine the mtrp identity.
MOF1 Determines Sterile Lemma Fate
The sterile lemma of grasses is a specific glume-like organ (Li et al., 2009; Ren et al., 2018a). In this study, the sterile lemma of the mof1 mutant was widened and had a chimeric upper surface with epidermal features of both the sterile lemma and the hull (lemma and palea) in the wild type. Compared with the wild type, the abnormal sterile lemma of the mof1 mutant exhibited more vascular bundles and trichomes, which were present in the lemma or mbp of the wild type. OsMADS1, OsMADS14, and OsMADS15, the marker genes of the hull (lemma and palea), are not expressed in the sterile lemma of the wild type, whereas their transcripts are detected in the abnormal sterile lemma in the mof1 mutant. DL and OsMADS6 are used as the marker genes for the identity of the lemma and palea, respectively, and only weak DL signals were found in the sterile lemma of the mof1 mutant. These findings implied that the sterile lemma is prone to transform into the lemma/mbp-like organ and acquire the lemma/mbp identity in part. Previous studies showed that OsMADS34, ASP1, EG1, FON4, and G1 function in the development of the sterile lemma (Li et al., 2009; Yoshida et al., 2009, 2012; Gao et al., 2010; Hong et al., 2010; Lin et al., 2014). In mutants of these genes, the sterile lemma continuously grows and undergoes a homeotic transformation into the lemma-like organ. Interestingly, the sterile lemma in some asp1 mutant spikelets was a chimeric organ with the identities of both the sterile lemma and the lemma, which was similar to the mof1 phenotype. These results suggest that OsMADS34, ASP1, EG1/DF1, FON4, and G1 repress the development of the lemma-like organ at sites where the sterile lemmas are present. Together with these studies, MOF1 affected the identity of the sterile lemma through suppression of the development of the lemma or mbp, and its mutation induced an incomplete transformation from the sterile lemma to the lemma or mbp.
One hypothesis has proposed that the putative spikelet in Oryza spp. ancestors has three fertile florets: one terminal floret and two lateral florets (Zhang et al., 2017; Ren et al., 2020). During evolution, the two lateral florets degenerated into sterile florets that contain only sterile lemmas derived from a morphological variation of the remaining lemma. Although loss-of-function mutation of OsMADS34, ASP1, EG1/DF1, FON4, and G1/ELE1 led to the transformation of the sterile lemmas into the lemma-like organ, the identity of the elongated sterile lemma in the asp1, eg1, and g1 mutants has not been confirmed by molecular evidence, whereas the identity has been confirmed in the osmads34 and fon4 to fon7 mutants. Interestingly, Oryza grandiglumis produces a lemma-like sterile lemma (Yoshida et al., 2009). In our study, the sterile lemma of the mof1 mutant was partially converted to a lemma or mbp-like organ. To some extent, these results support the hypothesis that the sterile lemmas are derived from the reduced lateral florets and the view that the lemma, mbp, and sterile lemma are homologous organs in rice (Kellogg, 2009; Lin et al., 2014; Ren et al., 2018a).
MATERIALS AND METHODS
Plant Materials
The recessive mutant mof1 was obtained from an M2 population of ‘Shuhui 527’ (Oryza sativa ssp. indica) mutagenized with ethylmethane sulfonate. All plants used for phenotypic analysis were grown under natural conditions in rice (Oryza sativa) paddies at the China National Rice Research Institute, Hangzhou (Zhejiang Province) and in Lingshui (Hainan Island), China.
Isolation of MOF1
To isolate the MOF1 gene, the mof1 mutant was crossed with a japonica cv W7. The 3822 F2 plants displaying the mutant phenotype were used as the mapping population. Gene mapping was performed using simple sequence repeat markers obtained from publicly available rice databases and genomic comparisons between Nipponbare and 9311. To construct a complementation vector, the 6.7-kb genomic sequence of MOF1 was cloned into the expression vector PCA1301. The construct was then introduced into mof1 calli using the Agrobacterium tumefaciens-mediated transformation method. To generate MOF1-knockout plants (mof1 Cas9), the MOF1-Cas9 vector was introduced into cv ZH11 calli. The target sequences are presented in Supplemental Table S1.
Cytological and Molecular Tests
Paraffin sectioning and scanning electron microscopy were performed as previously described (Ren et al., 2018a; Liang and Zhou, 2018). In brief, fresh specimens were observed using a scanning electron microscope (HITACHI S-3500) with a −30°C cool stage. For the paraffin sectioning, the plant specimens were fixed in 50% FAA solution (3.7% [v/v] formaldehyde, 50% [v/v] alcohol, 0.9 m acetic acid), dehydrated in ethanol, infiltrated with xylene, then embedded in paraffin (Sigma-Aldrich). The expression of the floral organ identity genes was detected in different organs using RT-qPCR and in situ hybridization. The RT-qPCR was performed as described by Ren et al. (2013). For in situ hybridization of the paraffin-embedded tissues, the probe synthesis, section preparation, hybridization, and immunological observation were all performed as described previously (Ren et al., 2013). The primer sequences are presented in Supplemental Table S1.
Transcription Activity Analysis of MOF1
The coding sequences of MOF1, FZP, and AH2 were inserted into the pGBKT7 plasmid containing the GAL4 DNA-BD. FZP-BD and AH2-BD were used as a positive and negative control in the study, respectively. MOF1-BD, FZP-BD, and AH2-BD were transformed into yeast strain AH109, and their transcriptional activity was analyzed using synthetic dropout medium (SD)/-Trp plates and SD/-Trp/-His/-Ade plates.
Furthermore, the transcriptional activity of MOF1 was analyzed using a dual luciferase reporter assay system in rice protoplasts. The constructs encoding full-length MOF1 and mof1 proteins and the truncated MOF1 proteins (MOF11–75-VP16, MOF124–75-VP16, MOF124–306-VP16, and MOF175–306-VP16) were transformed into rice protoplasts and cultured in the dark at 28°C for 14 h, after which the relative luciferase activity was measured using a GloMax 20-20 luminometer (Promega). The primers are shown in Supplemental Table S1.
Pull-Down Assay
We fused the coding sequence of MOF1 into the pMAL-c2x vector to construct the plasmid His-MOF1. The coding regions of TPR1, TPR2, and TPR3 were inserted into the pGEX4T-1 vector to construct GST-TPR1, GST-TPR2, and GST-TPR3, respectively. These plasmids were transformed into Escherichia coli BL21 competent cells, and isopropylthio-β-galactoside was used to induce the production of the proteins. For the in vitro GST pull-down assay, GST or GST fusion proteins coupled to glutathione sepharose 4 fast flow beads were incubated with His or His-LRG1 at 4°C for 30 min. The His and GST fusion proteins were detected using anti-His and anti-GST antibodies, respectively. The primers are shown in Supplemental Table S1.
Accession Numbers
Sequence data from this article for the cDNA and genomic DNA of MOF1, OsTRP1, OsTRP2, and OsTRP3 can be found in the GenBank/EMBL data libraries under accession numbers LOC_Os04g47890, LOC_Os01g15020, LOC_Os08g06480, and LOC_Os03g14980, respectively.
Supplemental Data
The following supplemental materials are available.
Supplemental Figure S1. Phenotypes of mof1 florets.
Supplemental Figure S2. Expression of organ identity genes.
Supplemental Figure S3. Protein sequence alignment of MOF1-like genes.
Supplemental Table S1. Primers used in the study.
LITERATURE CITED
Author notes
This work was supported by the National Key Research and Development Program of China (grant no. 2016YFD0100902–07), the Zhejiang Natural Science Foundation (grant no. LY18C130007), the National Natural Science Foundation of China (grant nos. 91735304 and 31801336), the Central Public-Interest Scientific Institution Basal Research Fund of China National Rice Research Institute (grant no. 2017RG001–4), the National Science and Technology Major Project (grant no. 2016ZX08009003–003–008), the Zhejiang Province Outstanding Youth Fund (grant no. LR19C130001), the National GMO New Variety Breeding Program of PRC (grant no. 2016ZX08011–001), and the Zhejiang Provincial “Ten Thousand Talent Program” Project (grant no. 2018R52025).
These authors contributed equally to the article.
Senior authors.
Author for contact: qianqian188@hotmail.
The author responsible for distribution of materials integral to the findings presented in this article in accordance with the policy described in the Instructions for Authors (www.plantphysiol.org) is: Qian Qian ([email protected]).
Q.Q. and D.R. designed the research; D.R., H.Y., Q.X., Y.C., X.Y., S.X., and H.L. performed the experiments; J.H., D.X., D.Z., G.Z., Z.G., L.Z., and L.G. analyzed the data; Q.X., Y.C., X.Y., S.X., and H.L. conducted the fieldwork; D.R., H.Y., and Y.R. wrote the manuscript.