-
PDF
- Split View
-
Views
-
Cite
Cite
Yubing He, Lang Yan, Chennan Ge, Xue-Feng Yao, Xiang Han, Rongchen Wang, Lizhong Xiong, Liwen Jiang, Chun-Ming Liu, Yunde Zhao, PINOID Is Required for Formation of the Stigma and Style in Rice, Plant Physiology, Volume 180, Issue 2, June 2019, Pages 926–936, https://doi.org/10.1104/pp.18.01389
- Share Icon Share
Abstract
The stigma is the entry point for sexual reproduction in plants, but the mechanisms underlying stigma development are largely unknown. Here, we disrupted putative auxin biosynthetic and signaling genes to evaluate their roles in rice (Oryza sativa) development. Disruption of the rice PINOID (OsPID) gene completely eliminated the development of stigmas, and overexpression of OsPID led to overproliferation of stigmas, suggesting that OsPID is a key determinant for stigma development. Interestingly, ospid mutants did not display defects in flower initiation, nor did they develop any pin-like inflorescences, a characteristic phenotype observed in pid mutants in Arabidopsis (Arabidopsis thaliana) and maize (Zea mays). We constructed double mutants of OsPID and its closest homolog, OsPIDb, yet the double mutants still did not develop any pin-like inflorescences, indicating that either ospid is compensated by additional homologous genes or OsPID has different functions in rice compared with PID in other organisms. We then knocked out one of the NAKED PINS IN YUC MUTANTS (NPY) genes, which cause the formation of pin-like inflorescences in Arabidopsis when compromised, in the ospid background. The ospid osnpy2 double mutants developed pin-like inflorescences, which were phenotypically similar to pid mutants in Arabidopsis and maize, demonstrating that the roles of OsPID in inflorescence development are likely masked by redundant partners. This work identified a key determinant for stigma development in rice and revealed a complex picture of the PID gene in rice development. Furthermore, the stigma-less ospid mutants are potentially useful in producing hybrid rice.
Pollen grains land and germinate on the stigma, which is therefore the entry point for male gametes reaching the female ovules during plant sexual reproduction. The stigma provides an essential environment and initial nutrients for pollen germination (Zinkl et al., 1999). The stigma also provides the first checkpoint for pollen recognition and guidance for pollen tube growth. Despite the obvious importance of stigmas in plant sexual reproduction, very little is known about the molecular mechanisms by which stigmatic tissue initiates and patterns. Previous studies of stigma development have focused on self-incompatibility and yield-related traits (Yu et al., 2017). For example, it is known that the stigma exsertion rate, stigma length, and breadth greatly affect the effectiveness of pollination (Liu et al., 2015; Zhou et al., 2017). Extensive studies on natural variation, in terms of stigma morphology, have been conducted in rice (Oryza sativa) in an attempt to identify better parental lines for hybrid rice breeding and to identify major quantitative trait loci related to stigma development (Dang et al., 2016). At the molecular level, genes expressed during stigma development have been identified using either enhancer-trapped transgenic lines, transcriptomics, or proteomics profiling (Li et al., 2007; Wang et al., 2014). However, the exact roles of these differentially and specifically expressed genes in stigma development have not been determined. Several mutants with defective stigma have been reported, but the genes responsible for the phenotypes have not been cloned (Yu et al., 2017).
Plants with defective stigma may have commercial value in the production of hybrids (Hanna and Powell, 1974; Yokoo, 1984). Very rarely, rice grains are produced from cross-pollination because both male and female floral organs are produced in the same flower. Traditionally, hybrid rice seed production has relied on male sterility systems to prevent self-pollination (Richharia et al., 1962; Lin and Yuan, 1980). However, the traditional methods have some disadvantages and are usually labor intensive. The two parental lines (one of them is male-sterile) need to be planted in close proximity in an orderly fashion so that cross-pollination can be achieved. The grains from the two parental lines have to be harvested separately because only the grains from the male-sterile parent are hybrids, making mechanical harvesting a challenge. Industrialization inevitably leads to a reduction in the number of farmers and an increase in labor costs. Therefore, new hybrid schemes are needed to simplify both planting and harvesting during hybrid production in rice. Identification of female-sterile rice mutants can potentially make it feasible to eliminate transplanting of seedlings during hybrid production. Rather, two parents, one of which is male-sterile and the other is female-sterile, can be directly sown to the fields in hybrid production. Any grains produced in this new scheme will be hybrids because either parent alone is not able to produce grains. Therefore, the new scheme may greatly reduce the labor required for planting and harvesting. However, very few rice mutants are suitable for such purposes (Qu et al., 2012).
Auxin is essential for almost all developmental processes in plants (Zhao, 2018). To elucidate the roles of auxin in regulating agriculturally important traits, we used reverse genetic approaches to disrupt rice genes that are homologous to the auxin biosynthesis/signaling-related genes identified and characterized in Arabidopsis (Arabidopsis thaliana) and in other plants. In Arabidopsis and maize (Zea mays), disruption of the protein kinase PINOID (PID) causes severe developmental defects (Bennett et al., 1995; Christensen et al., 2000; McSteen and Hake, 2001). Loss-of-function mutations of pid in Arabidopsis and maize lead to defects in flower initiation (Bennett et al., 1995; Christensen et al., 2000; McSteen and Hake, 2001; McSteen et al., 2007). The Arabidopsis pid mutants develop striking pin-like inflorescences (thus the name of PINOID; Bennett et al., 1995). Occasionally, Arabidopsis pid mutants produce flowers with multiple petals, but the flowers are completely sterile. Maize plants carrying mutations in the PID gene BARREN INFLORESCENCE2 (BIF2) also formed naked pin inflorescences (McSteen and Hake, 2001; McSteen et al., 2007), a phenotype even more severe than those observed in Arabidopsis. The bif2 mutants are defective in maintaining all axillary meristems, including branch, spikelet, and floral meristems, resulting in the formation of fewer branches, fewer flowers, and fewer floral organs. It has been proposed that PID is involved in auxin signaling/polar auxin transport (Christensen et al., 2000; Benjamins et al., 2001; Friml et al., 2004) by phosphorylating PIN auxin efflux carriers and by regulating PIN polarity (Michniewicz et al., 2007).
Here, we screened a rice TILLING population (Jiang et al., 2013) for mutations in OsPID, which shares the highest sequence similarity with the Arabidopsis PID (Morita and Kyozuka, 2007). Two mutant alleles of ospid mutants (ospid-1 and ospid-2) were identified from the screen. We also generated additional ospid alleles using the clusters of regularly interspaced short palindromic repeat (CRISPR)-associated protein 9 (Cas9)-based gene-editing technology. Phenotypic analysis showed that, surprisingly, none of the ospid mutants developed any pin-like inflorescences. Instead, these ospid mutants never formed any stigmas, a phenotype that was not observed in either Arabidopsis or maize pid mutants. Moreover, ospid mutants did not display pleiotropic phenotypes. The vegetative growth and development of ospid mutants were normal, suggesting potential applications of the female-sterile ospid plants in hybrid production. Because PID has been extensively studied in other systems, the identification of the unique function of OsPID in stigma development provides great resources for studying the conservation and diversification of gene functions.
RESULTS
Disruption of OsPID Completely Abolishes Stigma Formation
We screened the TILLING collection for mutations in the OsPID gene to study its role in rice development. We identified two ospid mutant alleles and named them ospid-1 and ospid-2 (Fig. 1A). ospid-1 contained a G-to-A substitution 490 bp downstream of the ATG start codon, resulting in an E164K conversion in the protein sequence. The ospid-2 mutant had a C-to-T conversion 959 bp downstream of the start codon, leading to an S320L substitution (Fig. 1A). E164 is a highly conserved amino acid residue in the kinase motif III (Fig. 1A; Supplemental Fig. S1), suggesting that E164K is likely to be a strong allele. S320 is located in the kinase motif VIII, which is also conserved among PID and other AGC kinases (AGC is the collective name for cAMP-dependent protein kinase A [PKA], cGMP-dependent protein kinase G [PKG], and phospholipids-dependent protein kinase C [PKC]; Fig. 1A; Supplemental Fig. S1).
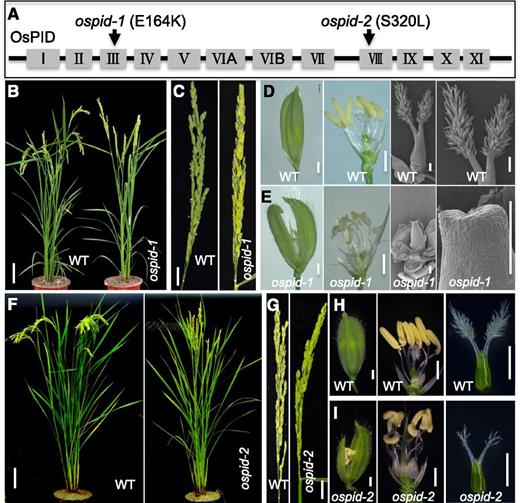
OsPID is required for stigma and style development in rice. A, Schematic presentation of OsPID and the mutations conferred by ospid-1 and ospid-2. The Roman numerals (I, II, III, IV, V, VIA, VIB, VII, VIII, IX, X, XI) represent the 12 conserved kinase domains of the OsPID protein. The black arrows refer to the locations of mutations. B, The ospid-1 plant fails to produce grains. The wild-type (WT) plant has bent panicles due to grain weight, but ospid-1 only has sterile straight panicles. C, Closeup views of the panicles of the wild type (left) and ospid-1 (right). D, A floret of the wild type with closed glume, six stamens, and two protruding and hairy stigmas on the pistil. E, The floret of ospid-1 with opened glume, more than six stamens, and bald pistil with no stigma. F, Wild-type (left) and ospid-2 (right) adult plants. Note that panicles of ospid-2 are not bent. G, Wild-type (left) and ospid-2 (right) panicles. H, Overall morphology of a wild-type floret (left), floret with glume removed (middle), and pistil (right). I, Morphology of ospid-2 flowers and floral organs. Note that the stigmas of ospid-2 are less hairy than wild-type stigmas. Bars = 10 cm in B and F, 2 cm in C and G, 1 mm in the left two images of D and E and in H and I, and 200 μm in the right two images of D and E.
No apparent differences between the wild type and ospid-1 were observed during vegetative growth, but ospid-1 displayed severe defects in setting grains (Fig. 1, B and C). At the anthesis stage, six stamens and a carpel with two protruding stigmas were visible in the wild-type flowers, and the palea and lemma were closed after fertilization (Fig. 1D). In contrast, in the ospid-1 flowers, the palea and lemma were often not completely closed (Fig. 1E). The most striking phenotype of ospid-1 was the complete lack of stigmas and styles (Fig. 1E) and consequently no grains produced (Fig. 1E). Vegetative growth was also unaffected in ospid-2 as compared with the wild type (Fig. 1F). Unlike ospid-1, ospid-2 had residual stigmas and styles. The stigmas of ospid-2 had much fewer stigma hairs (Fig. 1I) than the wild type (Fig. 1H), and ospid-2 showed a large reduction in grain setting compared with the wild type (Fig. 1G).
Although E164K is likely a strong loss-of-function allele, we generated null mutants of ospid to further confirm the phenotypes. We constructed the null alleles of ospid using CRISPR/Cas9-based gene-editing technology (Fig. 2). We obtained two ospid-c alleles (c stands for CRISPR). ospid-c1 harbored a 47-bp deletion and ospid-c2 contained a 4-bp deletion (Fig. 2A). Both ospid-c1 and ospid-c2 exhibited stigma-less phenotypes as observed in ospid-1 (Fig. 2, C and D), supporting the essential roles of OsPID in stigma formation.
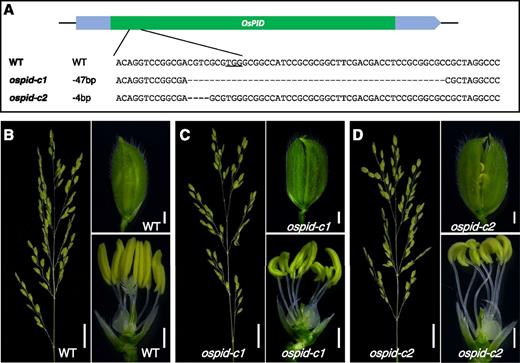
Generation of null alleles of ospid using CRISPR/Cas9 gene-editing technology. A, Schematic description of the CRISPR target and the mutations generated. The underlined area is the PAM site of the target sequence. The ospid-c1 mutant contains a 47-bp deletion in the OsPID coding region. ospid-c2 had a 4-bp deletion. B to D, A panicle, a floret, and a floret with glume removed are shown in the wild type (WT; B), ospid-c1 (C), and ospid-c2 (D). Note that both ospid-c1 and ospid-c2 lacked stigmas and styles. The ospid-c mutants also had more stamens. Bars = 2 cm in the left images of B to D and 1 mm in the right two images of B to D.
Overexpression of OsPID Leads to the Formation of Extra Stigmas
To further confirm that the stigma-less phenotype of ospid mutants was actually caused by the disruption of OsPID functions, we introduced a 12-kb genomic fragment, which included the promoter, 5′ and 3′ untranslated regions, and the open reading frame (ORF) of OsPID, into the ospid-1 mutant background. Phenotypic analyses of the pOsPID::OsPID transgenic plants revealed a complete restoration of stigma development (Fig. 3A) and fertility (Supplemental Fig. S2). Moreover, we noticed that some flowers in the complementation lines developed more than two stigmas (Fig. 3A). It is likely that the extra stigma phenotype was caused by elevated OsPID expression, as overexpression of OsPID under the control of the CaMV35S promoter leads to the development of more than two stigmas (Morita and Kyozuka, 2007). We next overexpressed OsPID under the control of the maize Ubiquitin promoter. As shown in Figure 3B, many flowers in the OsPID overexpression lines developed three stigmas and some even had four stigmas (Fig. 3, C and D). Our results clearly demonstrate that OsPID plays an essential role in stigma development.
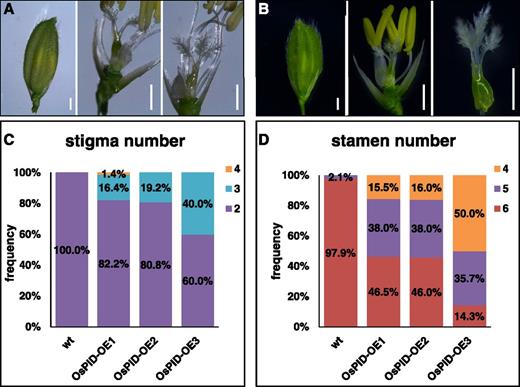
Overexpression of OsPID stimulates the formation of stigmas and represses the formation of stamens. A, Florets of ospid-1 plants complemented with an OsPID genomic fragment. A floret with palea and lemma closed (left), a floret with two stigmas (middle), and a floret with three stigmas (right) are shown. B, OsPID overexpression plants developed more than three stigmas and fewer than six stamens. C, Quantitative analysis of stigma numbers in wild-type (wt) and OsPID-overexpressing (OsPID-OE) flowers. Data shown are from three independent OsPID overexpression lines. The inset graphic markers 2, 3, and 4 refer to stigma numbers. D, Overexpression of OsPID leads to fewer stamens. The inset graphic markers 4, 5, and 6 refer to stamen numbers. Total numbers of the flowers analyzed in C and D are 47, 73, 52, and 25 for the wild type, OsPID-OE1, OsPID-OE2, and OsPID-OE3, respectively. Bars = 1 mm in A and B.
OsPID Is Involved in the Formation of Other Floral Organs
Although a lack of style and stigma was the most striking defect of ospid mutants (Figs. 1 and 2), we noticed that disruption of OsPID actually affected the formation of other floral organs as well. In particular, the number of stamens in ospid mutants was increased (Fig. 1, C and D) as compared with the six stamens in the wild-type flowers. Interestingly, overexpression of PID led to a reduction of stamens. More than half of the OsPID overexpression lines contained fewer than six stamens. Some flowers only formed four stamens (Fig. 3). We also found that 26 out of 65 ospid-1 florets contained glume-like structures that were not observed in any of the wild-type florets (n = 80; Supplemental Fig. S3). Many ospid-1 florets (25/65) had two lodicules or lodicule-like structures (Supplemental Table S1; Supplemental Fig. S3). Although ospid mutants had more stamens, they actually produced fewer pollen grains, but the pollen grains appeared to be viable (Supplemental Fig. S3).
Initiation of Branches and Flowers Is Normal in Ospid Mutants
The most striking phenotypes of pid mutants in Arabidopsis and maize is the failure of flower initiation and the development of pin-like inflorescences (Bennett et al., 1995; McSteen et al., 2007). In maize, PID is involved in almost all of the lateral organ development by maintaining normal axillary meristems. However, disruption of rice PID appeared not to affect flower initiation. The number of florets (spikelets) per panicle was similar to that of the wild type (Supplemental Fig. S4). Moreover, the number of primary branches per panicle and the number of secondary branches per primary branch were not significantly reduced in the ospid-1 mutant when compared with the wild type (Supplemental Fig. S4). ospid-1 was completely sterile, whereas ospid-2 had about 30% fertility (Supplemental Fig. S4).
OsPID Is Not Compensated by Its Closest Homolog OsPIDb
We then addressed whether the failure of ospid to develop pin-like inflorescences was caused by the compensatory effects of PID homologs, as rice has two genes (OsPID and OsPIDb) that are closely related to the Arabidopsis PID (Fig. 4A). Although maize also has two copies of PID (Fig. 4A), the maize pid single mutants phenocopy the Arabidopsis pid mutants. We generated knockout mutants of OsPIDb using CRISPR/Cas9-based gene-editing technology. ospidb-c1 had a 1-bp deletion and ospidb-c2 contained a single T insertion (Fig. 4B), and both mutations were expected to cause frame shift and premature termination in translation. These single ospidb mutants were indistinguishable from wild-type plants (Fig. 4, C and D; Supplemental Fig. S5). We then generated ospid-1 and ospidb double mutants and found that the double mutants were similar to the ospid-1 single mutant (Fig. 4, E and F; Supplemental Fig. S6), suggesting that the lack of pin phenotype was not caused by the genetic redundancy between OsPID and OsPIDb.
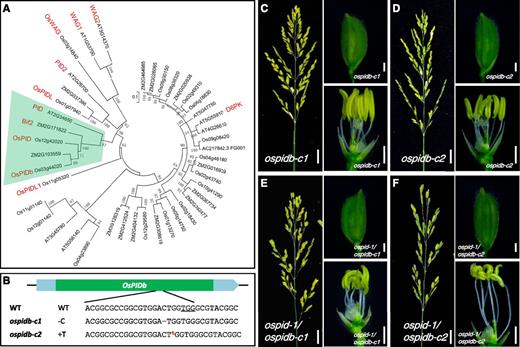
Generation of null mutants of OsPIDb and genetic interactions between ospid and ospidb. A, Phylogenetic analysis of PID and related kinases from rice, maize, and Arabidopsis. The multisequence alignment and phylogenetic tree were constructed using MEGA5. The protein sequences are shown in Supplemental Figure S1. The PID subfamily is highlighted with light green shading. PID, PID2, WAG1, WAG2, and D6PK are five homologous proteins in Arabidopsis. Bif2 is the PID ortholog in maize. OsPID, OsPIDb, OsPIDL, OsPIDL1, and OsWAG are the five closest PID homologs in rice. The numbers next to the branches refer to the percentages of replicate trees in which the associated taxa clustered together in the bootstrap test (1,000 replicates). B, Generation of two null alleles of ospidb mutants using CRISPR gene-editing technology. The target sequence and PAM site (underlined) are shown. Both ospidb-c1 and ospidb-c2 had 1-bp changes. The dash represents a C deletion, and t in red and superscript refers to a T insertion. WT, Wild type. C and D, A panicle and a floret of ospidb single mutants ospidb-c1 (C) and ospidb-c2 (D). Note that the panicle, floret, stamen, and stigma appear normal. E and F, Analysis of the double mutants ospid-1 ospidb-c1 (E) and ospid-1 ospidb-c2 (F). Both sets of double mutants are very similar to the ospid-1 single mutant. Bars = 2 cm in the left images of C to F and 1 mm in the right two images of C to F.
OsPID Is Not Exclusively Located in the Plasma Membrane
We used a transient expression system to investigate the subcellular localization of the OsPID-GFP fusion. In Arabidopsis, PID is localized at the cell periphery but not in the nucleus (Lee and Cho, 2006; Zegzouti et al., 2006; Michniewicz et al., 2007). In maize, PID is localized at the plasma membrane and in the nucleus (Skirpan et al., 2008). Interestingly, OsPID had a broad subcellular distribution. In addition to cytoplasmic signals, OsPID-GFP colocalized with a nuclear marker (Fig. 5, A–C). OsPID-GFP was also clearly colocalized with the plasma membrane marker (Fig. 5D). The subcellular localization pattern of OsPID was more similar to that of maize than to that of Arabidopsis. A caveat of the transient expression system is that the observed subcellular localization (Fig. 5) may not reflect situations under physiological conditions. Further studies are needed to conclusively determine the localization of OsPID and whether OsPID has a nuclear function.
![Subcellular localization of OsPID-GFP. A, Transient expression of OsPID-GFP and NLS-RFP (nuclear localization marker) in protoplasts. B, OsPID-GFP and CNX-RFP (endoplasmic reticulum [ER] localization marker) localization. C, OsPID-GFP and Man1-RFP fusion (Golgi localization marker) localization. D, OsPID-GFP in cells where FM4-64 was used to stain the plasma membrane of the OsPID-GFP expression protoplasts. For each localization experiment, more than 30 individual cells were analyzed using confocal imaging. The patterns shown were observed in more than 70% of the cells analyzed. DIC, Differential interference contrast. Bar = 10 μm.](https://oup.silverchair-cdn.com/oup/backfile/Content_public/Journal/plphys/180/2/10.1104_pp.18.01389/2/m_plphys_v180_2_926_f5.jpeg?Expires=1747882111&Signature=zLv4F9IqXvyMpxBQASHDvL2MR71vnQYWfmuWvfF-qf5f02qkuEhs1MLfrSFlwBO49GPofpnojaz7p5KDoVRYQLhw8tSEOzvChM~OKUot5b3sCZhe4GD5UR3LzuioefiSaGN3X-WUs2~4JfHECb195gQ0-RY-FRGEDtBmyAQ6E~3g8hMaSF3K7IfoCz~aCKJRvXRjMj4PmImazinMLgjgvjKsSuvE8VourjPFGPlBQXeN9MF7eWsWEyJVaR95IepxDVSzhSkbywagkEuEkD0QyMmgQqepaQWkB5fJ~jxlptTUw-Xd2ArSYM1~XoGyI4C2VI4F9a~GSD~YsOamP8DEeA__&Key-Pair-Id=APKAIE5G5CRDK6RD3PGA)
Subcellular localization of OsPID-GFP. A, Transient expression of OsPID-GFP and NLS-RFP (nuclear localization marker) in protoplasts. B, OsPID-GFP and CNX-RFP (endoplasmic reticulum [ER] localization marker) localization. C, OsPID-GFP and Man1-RFP fusion (Golgi localization marker) localization. D, OsPID-GFP in cells where FM4-64 was used to stain the plasma membrane of the OsPID-GFP expression protoplasts. For each localization experiment, more than 30 individual cells were analyzed using confocal imaging. The patterns shown were observed in more than 70% of the cells analyzed. DIC, Differential interference contrast. Bar = 10 μm.
Development of Pin-Like Inflorescences in ospid osnpy2 Double Mutants
The phenotypic differences between ospid and maize pid are likely caused by different levels of genetic redundancy between the two species. In addition to OsPIDb, there are at least three other genes that may have overlapping functions with OsPID (Fig. 4A). Construction of multiple mutants of OsPID-like genes will take a couple of years. Arabidopsis pid mutants genetically interact with naked pins in yuc mutants1 (npy) mutants in controlling Arabidopsis organogenesis (Cheng et al., 2007b). For example, npy1 npy3 npy4 triple mutants in Arabidopsis closely resembled the Arabidopsis pid mutants (Cheng et al., 2008). Moreover, npy1 pid double mutants completely abolished the formation of cotyledons (Cheng et al., 2008). NPY genes encode proteins that contain a Bric-a-brac, Tramtrack, Broad-complex/Poxvirus Zinc finger domain, but the biochemical functions of NPY proteins have remained unsolved. We hypothesized that we could overcome the genetic redundancy obstacle by knockout of NPY genes in the background of ospid.
We used CRISPR/Cas9-based gene-editing technology to generate several alleles of osnpy2 (Fig. 6A). Both osnpy2-c1 and osnpy2-c2 had a 1-bp insertion that led to a frame shift. Although these osnpy2 single mutants were very similar to wild-type plants (Fig. 6B), the npy2 ospid-1 double mutants completely abolished the formation of flowers and developed pin-like inflorescences (Fig. 6; Supplemental Fig. S7). The double mutants also had defects in vegetative growth and made fewer tillers (Fig. 6).
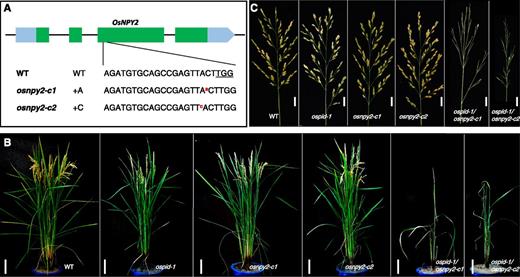
Analysis of the genetic interaction between ospid and osnpy2. A, Two independent mutants of OsNPY2 were generated using CRISPR/Cas9. osnpy2-c1 contains an insertion of A; osnpy2-c2 contains an insertion of C near the PAM site (underlined). The a and c in red and superscript refer to the insertions in the two osnpy2 mutants. B, Synergistic genetic interactions between ospid-1 and osnpy2. The osnpy2 single mutants do not display obvious developmental phenotypes. However, the ospid osnpy2 double mutants are dwarf with fewer tillers. More importantly, the double mutants fail to produce flowers and display naked pin-like inflorescences. C, Defects of the ospid osnpy2 double mutants in flower initiation. The double mutants still made first and secondary branches without flowers. WT, Wild type. Bars = 10 cm in B and 2 cm in C.
DISCUSSION
In this study, we isolated ospid mutants from a TILLING collection and generated additional alleles of ospid using CRISPR/Cas9-based gene-editing technology. We have clearly shown that rice plants did not develop any pistils when the OsPID gene was compromised, revealing a new role for the PID family of AGC kinases. We further investigated why ospid mutants were phenotypically different from the pid mutants in Arabidopsis and maize (Bennett et al., 1995; McSteen and Hake, 2001), even though both maize and rice are grasses. We conclude that there is likely extra genetic redundancy among OsPID and its homologs during inflorescence development. We demonstrate that OsPID is involved in several major developmental processes, including tillering and flower initiation, by analyzing the ospid osnpy2 double mutants. The finding that OsPID is necessary and sufficient for the formation of stigmatic tissues may have applications in producing hybrid rice.
A Conserved Genetic Network Controls Organogenesis in Plants
The ospid mutants did not have defects in flower initiation and did not develop pin-like inflorescences (Figs. 1 and 2), which are characteristics of pid phenotypes in Arabidopsis and maize (Bennett et al., 1995; McSteen and Hake, 2001). In contrast, ospid mutants did not form any styles and stigmas (Figs. 1 and 2), whereas pid mutants in Arabidopsis and maize do not have such defects. Taking these observations at face value may lead to the conclusion that OsPID has gained new functions and OsPID may use a different mechanism to control plant developmental processes. However, we still believe that OsPID and PID in other species share a conserved molecular mechanism by which plant development is regulated, for several reasons. First, the main defects in both Arabidopsis pid and ospid are failures of organogenesis, although the specific organs affected are not the same. Previous genetic studies in Arabidopsis demonstrated clearly that the formation of each organ is controlled by different levels of genetic redundancy (Cheng et al., 2007b; Furutani et al., 2007). For example, Arabidopsis pid mutants displayed strong pin-like inflorescence phenotypes, but pid mutants are quite normal during embryogenesis and vegetative development (Bennett et al., 1995). PID is dynamically expressed in embryos, seedlings, and young adults, suggesting that PID plays important roles in those processes (Christensen et al., 2000; Benjamins et al., 2001). PID and its closest homologs, WAVY ROOT GROWTH1 (WAG1), WAG2, and PID2, have overlapping functions during embryogenesis and vegetative growth (Cheng et al., 2007b). The pid wag1 wag2 pid2 quadruple mutants completely abolish cotyledon development (Cheng et al., 2007b). The quadruple mutants also make few true leaves, which have abnormal shapes and vascular patterns. These results indicate that the OsPID homologs (OsPIDb, OsPIDL, OsPIDL1, and OsWAG; Fig. 4A) likely have overlapping functions during organogenesis and that the homologs of OsPID may mask the phenotypes of ospid in inflorescence development. We hypothesize that proper combinations of ospid and mutations of OsPID homologs are likely to disrupt flower initiation and inflorescence development. It will be interesting to investigate the expression patterns of the OsPID homologs, which may provide guidance for generating high orders of ospid mutants.
Second, the observed synergistic genetic interaction between ospid and osnpy2 is indicative that PID in rice and PID in other species actually use a conserved pathway for organogenesis. Several Arabidopsis mutants, including pin-formed1 (pin1), pid, npy, and yucca (yuc), are known to be defective in organogenesis (Okada et al., 1991; Bennett et al., 1995; Cheng et al., 2006, 2007a, 2008). Previous genetic studies in Arabidopsis have placed PID, NPY, YUC, and PIN in the same auxin-mediated organogenesis pathway (Cheng et al., 2007b, 2008; Furutani et al., 2007). The same no-cotyledon phenotypes can be achieved in several ways as long as the final auxin signal output is decreased below the threshold of organ initiation. The pid wag1 wag2 triple mutants lack cotyledons (Cheng et al., 2008). The same no-cotyledon phenotypes were observed in npy1 pid double mutants (Cheng et al., 2007b). Moreover, pid yuc1 yuc4 triple mutants also failed to develop cotyledons, linking auxin to cotyledon initiation and development because both YUC1 and YUC4 encode the rate-liming enzymes in auxin biosynthesis (Cheng et al., 2008). The pin1 pid double mutants also fail to make any cotyledons (Furutani et al., 2004). The genetic enhancement between npy1 and yuc1 yuc4 during inflorescence development also supports the roles of NPY1 in flower initiation, although npy1 alone does not display any obvious developmental defects (Cheng et al., 2007b). The fact that the ospid osnpy2 double mutants made striking pin-like inflorescences (Fig. 6), which phenocopied pid and bif2 (Bennett et al., 1995; McSteen and Hake, 2001), demonstrate that OsPID is important beyond stigma development and that rice and other plant species use similar components and strategies to control various organogenesis processes. We noticed that YUC, PID, PIN, and NPY all belong to gene families with many members, providing a genetic foundation for complex and diversified genetic control of organogenesis.
Potential Applications of ospid in Hybrid Rice Seed Production
The hybrid rice breeding technology has had a major impact on improving food security (Van Nguyen and Ferrero, 2006), but the production of hybrid rice seeds is still labor intensive and expensive. The ospid mutants lacked stigma and style (Figs. 1 and 2), making them completely female-sterile. The frequency of stigma defects of ospid was 100%. The ospid mutants have extra stamens and produce viable pollen grains (Figs. 1 and 2; Supplemental Fig. S3). Furthermore, ospid appears quite normal during seedling and young adult stages (Figs. 1 and 2). Therefore, ospid mutants show potential for applications in hybrid rice production.
Nuclear male-sterile lines have been used for hybrid rice production (Chang et al., 2016). The male-sterile lines are maintained with a transgene that complements the male-sterile phenotypes (Chang et al., 2016). The transgene is coupled with a fluorescence marker and other morphology selection markers for the transgenes. Similar strategies can be used for maintaining and selecting ospid homozygous grains.
Because male-sterile rice lines are widely available, our identification of ospid mutants without stigma and style opens the door for direct seeding of two sterile parents in producing hybrids. Only hybrids are produced if a male-sterile parent is grown next to a female-sterile parent, greatly reducing labor and production costs.
MATERIALS AND METHODS
Plant Materials and Growth Conditions
Wild-type rice (Oryza sativa ssp. japonica) ‘Zhonghua 11’ (ZH11) was used in this study. Both ospid-1 and ospid-2 mutant lines were derived from a population of 6,000 ethyl methanesulfonate (EMS)-mutagenized M2 rice plants in the ZH11 background (Jiang et al., 2013). The ospid-c1, ospid-c2, ospidb-c1, ospidb-c2, osnpy2-c1, and osnpy2-c2 mutants were generated using CRISPR/Cas9-based gene-editing technology (Gao and Zhao, 2014; Sun et al., 2016; He et al., 2017; Zhang et al., 2017). All rice materials were grown in an experimental field in Wuhan or Hainan during the natural growing season. The spacing between every two plants was 26.6 cm × 16.7 cm in the field.
Isolation of ospid Mutants from an EMS-Mutagenized Population
The ZH11 EMS TILLING mutant library (Jiang et al., 2013) was screened for mutations in the OsPID gene. For the TILLING screening, the primer pair ospidtiF and ospidtiR was used to amplify the ORF region of the OsPID gene (Jiang et al., 2013), targeted to a conserved domain. Native CEL1 was homemade from celery (Apium graveolens) for the cleavage of mismatched double-stranded DNA (Jiang et al., 2013), and cleaved products were detected by capillary electrophoresis using AdvanCE FS96 (Advanced Analytical Technologies).
Any putative ospid mutants were subsequently sequenced to identify the exact molecular lesion. The ospid-1 and ospid-2 mutants studied in this work contained mutations in the conserved domains of the OsPID protein. The offspring of ospid-1 and ospid-2 and the backcrossed progeny were grown in the field for phenotypic analysis. The ospid mutants were genotyped using derived cleaved-amplified polymorphic sequence (dCAPS) markers. The dCAPS primer pairs for ospid-1 and ospid-2 were ospid-1F/ospid-1R and ospid-2F/ospid-2R, respectively (Supplemental Table S2). The PCR products of ospid-1 and ospid-2 mutant lines were resistant to BsmAI and Eam1105I digestion, respectively, whereas wild-type products were cleaved by the two enzymes.
Overexpression of OsPID
The primer pair ospidOEF/ospidOER (Supplemental Table S2) and wild-type rice ‘Nipponbare’ genomic DNA were used to amplify the genomic fragment of OsPID. The PCR fragment was cloned into the plasmid pU1301 (Sun et al., 2004) at the KpnI site through Gibson assembly (Gibson et al., 2009). The pUBIpro::OsPID construct was confirmed by sequencing and then introduced into wild-type rice ZH11 by Agrobacterium tumefaciens-mediated genetic transformation (Hiei et al., 1994). Transgenic plants of pUBIpro::OsPID were identified by PCR amplification with the primer pair hyg280F/hyg280R for the presence of the selection marker gene HPTII. The relative expression levels of the OsPID gene in the transgenic plants of pUBIpro::OsPID were determined by reverse transcription quantitative PCR using the primer pair ospid-qRT-F/ospid-qRT-R. The primer pair Ubq-qRT-F/Ubq-qRT-R was specific for the rice Ubiquitin gene, which served as the endogenous reference gene (Wu et al., 2008).
Genetic Complementation of ospid-1
The ospidCOMKF/ospidCOMBR primer pair and the wild-type rice cv ‘Nipponbare’ bacterial artificial chromosome plasmid DNA No. a0016K01 were used as templates to amplify a 12-kb genomic fragment that contains the promoter, ORF, and the terminator of OsPID. The fragment was cloned into the plasmid pCAMBIA2301 between the KpnI and BamHI sites using Gibson assembly. After the pOsPIDpro::OsPID construct was verified by sequencing, it was introduced into the homozygous ospid-1 mutant by A. tumefaciens-mediated genetic transformation. The homozygous ospid-1 callus was dedifferentiated from the progeny of heterozygous ospid-1 plants. The transgenic plants of pOsPIDpro::OsPID were screened by PCR amplification using the primer pair G418F/G418R for the presence of selection marker gene NPTII.
CRISPR/Cas9 Plasmid Construction and Genetic Transformation
The pCXUN-CAS9 (Sun et al., 2016; He et al., 2017) vector was used to construct the pOsPID-CR, pOsPIDb-CR, and pOsNPY2-CR gene-editing vectors. First, the target sequences of OsPID, OsPIDb, and OsNPY2 were selected using the CRISPR-P Web tool (http://crispr.hzau.edu.cn/CRISPR2/). The single guide RNA (sgRNA) was driven by the OsU3 promoter. Second, the sgRNA transcriptional unit was generated by overlapping PCR. The first round of PCR consisted of two PCRs (PCR1 and PCR2). For pOsPID-CR construction, the primer pair of PCR1 was pCXUNU3-F/ospid-U3R. The primer pair of PCR2 was pCXUNU3-R/ospid-U3F. For pOsPIDb-CR construction, the primer pairs pCXUNU3-F/ospidb-U3R and pCXUNU3-R/ospidb-U3F were used for PCR1 and PCR2, respectively. For pOsNPY2-CR construction, the primer pairs for PCR1 and PCR2 were pCXUNU3-F/osnpy2-U3R and pCXUNU3-R/osnpy2-U3F, respectively. The template for both PCR1 and PCR2 was the U3 sgRNA transcription cassette DNA (He et al., 2017). In the second round of PCR, 0.5 μL of PCR1 product and 0.5 μL of PCR2 product for each construction were mixed as the template. The pCXUNU3-F/pCXUNU3-R primer pair was used for amplification. Third, each PCR product from the second round was purified and then inserted into the pCXUN-CAS9 plasmid at the KpnI site through Gibson assembly. All of the CRISPR constructs were sequenced before transformation into A. tumefaciens strain EHA105. Subsequently, the pOsPID-CR plasmid was introduced into wild-type rice ZH11. The pOsPIDb-CR and pOsNPY2-CR constructs were transformed into the heterozygous ospid-1 calli.
Genetic Analysis of CRISPR Mutants
T0 generation plants were grown in the field. Transgenic plants of ospid-c were identified by PCR using the primer pair Cas9-377F/Cas9-377R for the presence of Cas9. The T0 plants were self-pollinated or backcrossed with the wild-type ZH11, and the T1 or F1 grains were harvested. The T1 or F1 plants were grown in the field, and the Cas9-free plants were identified with the primer pair Cas9-377F/Cas9-377R. The OsPID locus in the Cas9-free plants was sequenced. The T2 or F2 grains were harvested for genetic analysis based on the cosegregation between the genotype and the phenotype among the T2 or F2 plants. For ospid-c1, the PCR products generated from the primer pair ospid-crF/ospid-crR were directly distinguished by electrophoresis in 2% (w/v) agarose (ospid-c1 was a 47-bp deletion mutant). For ospid-c2, the PCR products generated from the primer pair ospid-crF/ospid-crR were distinguished by 2% (w/v) agarose electrophoresis after digestion with AatII. Wild-type PCR products were digested, whereas that of ospid-c1 was resistant to the enzyme digestion (ospid-c2 contained a 4-bp deletion). To genotype ospidb-c1 (a C deletion) and ospidb-c2 (a T insertion) mutants, a CAPS marker was used with BseNI digestion. The PCR product amplified by the primer pair ospidb-crF/ospidb-crR was easily distinguished using 1% (w/v) agarose electrophoresis after BseNI digestion. PCR products from the wild type were cut by BseNI, whereas mutants were resistant. In osnpy2-c1, an A insertion before the PAM site of the target sequence generated a HapI recognition site. HapI digestion of the PCR product amplified using the primer pair osnpy2-cr1F/osnpy2-cr1R was used to differentiate the mutant from the wild type. A dCAPS marker was used to genotype osnpy2-c2 (a T insertion). EcoRI was used to cut the PCR products generated by primer pair osnpy2-cr2F/osnpy2-cr2R. PCR products from the mutant were digested by EcoRI, whereas wild-type products were not digested.
Transient Expression in Protoplasts and Confocal Imaging
The transient expression plasmid CaMV35S::OsPID-GFP was constructed by inserting the OsPID ORF without the stop codon into the XbaI site of the pBI221-GFP. Protoplasts were isolated from dark-grown Arabidopsis (Arabidopsis thaliana). Transient expression of the OsPID-GFP fusion was conducted using a protocol described previously (Zeng et al., 2015). Confocal fluorescent images were acquired 16 to 18 h after transformation. Photographs were taken using the Leica SP8 confocal system. For cell membrane staining, the cells were treated with 5 μm FM4-64 for 5 min before observation. For each experiment or construct, more than 30 individual cells were analyzed for confocal imaging.
Scanning Electron Microscopy
The florets were collected from wild-type and ospid mutant plants by removing the palea and lemma. Then, the tissues were fixed in 2.5% (v/v) glutaraldehyde overnight at 4°C. The tissues were postfixed in 1% (w/v) osmium tetroxide for 2 h at 4°C and then critical-point dried with carbon dioxide. Subsequently, the dried samples were coated with platinum powder and imaged using the scanning electron microscope.
Iodine-Potassium Iodide Staining
Florets were collected and placed in 70% (v/v) alcohol and then stored at 4°C for a short time. To analyze pollen fertility, we removed the anthers from the flowers and placed them on a glass slide with tweezers. Approximately one to two drops of 1% (w/v) iodine-potassium iodide solution were added, and then the anthers were broken mechanically to release the pollen. After the slide was covered with a cover glass, pollen grains were visualized with a microscope. Fertile pollen grains were dyed very deeply and had a round shape. Pollen with light staining or wrinkling was considered sterile.
Phylogenetic Tree Analysis of PID and PID Homologs
The evolutionary history of rice, maize (Zea mays), and Arabidopsis PID and PID homologs was inferred using the neighbor-joining method (Saitou and Nei, 1987). The bootstrap consensus tree inferred from 1,000 replicates (Felsenstein, 1985) was taken to represent the evolutionary history of the taxa analyzed. Branches corresponding to partitions reproduced in less than 50% of bootstrap replicates were collapsed. The percentages of replicate trees in which the associated taxa clustered together in the bootstrap test (1,000 replicates) were shown next to the branches. The tree was drawn to scale, with branch lengths in the same units as those of the evolutionary distances used to determine the phylogenetic tree. The evolutionary distances were computed using the JTT matrix-based method (Jones et al., 1992) and were in units of the number of amino acid substitutions per site. The rate variation among sites was modeled with a gamma distribution (shape parameter = 1). All positions containing gaps and missing data were eliminated. There were 314 positions in the final data set. Evolutionary analyses were conducted in MEGA5 (Tamura et al., 2011).
Accession Numbers
Accession numbers of rice genes mentioned in this study are as follows: OsPID (LOC_Os12g42020), OsPIDb (LOC_Os03g44020), OsNPY2 (LOC_Os06g08550), OsWAG (LOC_Os03g14840), OsPIDL (LOC_Os01g07940), and OsPIDL1 (LOC_Os11g05320). Accession numbers of Arabidopsis genes are as follows: PID (AT2G34650), PID2 (AT2G26700), WAG1 (AT1G53700), WAG2 (AT3G14370), and D6PK (AT5G55910). The accession number of maize BIF2 is GRMZM2G171822. The accession numbers of the genes from Arabidopsis, rice, and maize were obtained from https://www.arabidopsis.org, http://rice.plantbiology.msu.edu, and https://www.maizegdb.org, respectively.
Supplemental Data
The following supplemental materials are available.
Supplemental Figure S1. Sequence alignment of PID and its close homologs from rice, maize, and Arabidopsis.
Supplemental Figure S2. Complementation of ospid-1 with a genomic DNA fragment of OsPID.
Supplemental Figure S3. ospid mutants produce extra glumes, enlarged lodicules, and viable pollen grains.
Supplemental Figure S4. Analysis of some agronomic traits of ospid-1 and ospid-2 mutants.
Supplemental Figure S5. Panicle traits of the OsPIDb mutants are similar to those of wild-type plants.
Supplemental Figure S6. Statistical analysis of the panicle traits of the OsPIDb CRISPR mutants in the ospid-1 background.
Supplemental Figure S7. Closeup view of the inflorescences of ospid osnpy double mutants, which failed to produce flowers.
Supplemental Table S1. Comparison of the number of floral organs between the wild type and ospid-1.
Supplemental Table S2. Primers used in this study.
ACKNOWLEDGMENTS
We thank the CAMBIA lab (https://cambia.org) for providing the pCAMBIA2301 plasmid.
LITERATURE CITED
Author notes
This work was supported by the Chinese Ministry of Agriculture and Rural Affairs (2018ZX0801003B and 2016ZX08010002), the Huazhong Agricultural University Scientific and Technological Self-Innovation Foundation (2012YB04), and a startup fund from the Huazhong Agricultural University.
Author for contact: [email protected].
Senior author.
The author responsible for distribution of materials integral to the findings presented in this article in accordance with the policy described in the Instructions for Authors (www.plantphysiol.org) is: Yunde Zhao ([email protected]).
Y.Z. conceived the original research plans; Y.H. performed most of the experiments; L.Y., C.G., X.-F.Y., X.H., and R.W. provided some experimental materials and conducted some experiments; L.X., L.J., and C.-M.L. supervised some of the experiments; Y.H. and Y.Z. wrote the article with contributions from all of the authors.
Articles can be viewed without a subscription.