-
PDF
- Split View
-
Views
-
Cite
Cite
Cuilan Shi, Yulong Ren, Linglong Liu, Fan Wang, Huan Zhang, Peng Tian, Tian Pan, Yongfei Wang, Ruonan Jing, Tianzhen Liu, Fuqing Wu, Qibing Lin, Cailin Lei, Xin Zhang, Shanshan Zhu, Xiuping Guo, Jiulin Wang, Zhichao Zhao, Jie Wang, Huqu Zhai, Zhijun Cheng, Jianmin Wan, Ubiquitin Specific Protease 15 Has an Important Role in Regulating Grain Width and Size in Rice, Plant Physiology, Volume 180, Issue 1, May 2019, Pages 381–391, https://doi.org/10.1104/pp.19.00065
- Share Icon Share
Abstract
Ubiquitination and deubiquitination are reversible processes that play crucial roles in regulating organ size in plants. However, information linking deubiquitination and seed size in rice (Oryza sativa) is limited. Here, we characterized a dominant large-grain mutant, large grain1-D (lg1-D), with a 30.8% increase in seed width and a 34.5% increase in 1,000-grain weight relative to the wild type. The lg1-D mutant had more cells oriented in the lateral direction of the spikelet hull compared with the wild type. Map-based cloning showed that LG1 encodes a constitutively expressed ubiquitin-specific protease15 (OsUBP15) that possesses deubiquitination activity in vitro. Loss-of-function and down-regulated expression of OsUBP15 produced narrower and smaller grains than the control. A set of in vivo experiments indicated that the mutant Osubp15 had enhanced protein stability relative to wild-type OsUBP15. Further experiments verified that OsDA1 directly interacted with OsUBP15. Genetic data indicated that OsUBP15 and GRAIN WIDTH 2 (GW2) were not independent in regulating grain width and size. In summary, we identified OsUBP15 as a positive regulator of grain width and size in rice and provide a promising strategy for improvement of grain yield by pyramiding OsUBP15 and gw2.
Rice (Oryza sativa), as one of the most important cereal crops, provides the caloric intake for more than half of the world population. Grain size and shape, made up of grain width, length, thickness, and grain length-to-width ratio, are key determinants of grain yield and quality in rice (Huang et al., 2013).
In rice, grain size and weight are influenced by numerous genes involved in regulation of endosperm development and grain filling. THOUSAND-GRAIN WEIGHT 6 (TGW6), encoding an indole-3-acetic acid-Glc hydrolase, limits the number of endosperm cell layers and grain length (Ishimaru et al., 2013). GRAIN INCOMPLETE FILLING1 (GIF1), encoding a cell wall invertase, has a crucial role in Suc unloading during grain development; loss-of-function mutation of GIF1 causes slow grain filling, resulting in decreased grain weight in gif1 mutant (Wang et al., 2008). In contrast to TGW6 and GIF1, which directly affect the endosperm, a number of genes controlling grain size and weight affect the size of the spikelet hull, thereby indirectly regulating endosperm development. Many studies reveal that cell number in the lateral or longitudinal direction of the hull is an important factor determining hull size. For example, GW6, a GNAT-like protein with intrinsic histone acetyltransferase activity (OsglHAT1), positively regulates grain weight by enlarging spikelet hulls through increased cell number in the longitudinal direction (Zhou et al., 2015). Loss-of-function mutation of XIAO generates short and small grain due to decreased cell numbers making up the spikelet hull (Jiang et al., 2012). FUWA, encoding an NHL (NCL-1, HT2A and Lin-41) domain-containing protein, negatively regulates grain width by limiting latitudinal cell numbers in the lemma and palea (Chen et al., 2015). Os-Protein Phosphatases with Kelch-like repeats 1 acts as a negative regulator of grain length by decreasing the cell number of spikelet hulls (Zhang et al., 2012). Apart from cell number, cell size and shape are two additional factors affecting hull size. Loss-of-function mutation of either DENSE PANICLE1 or SMALL AND ROUND SEED 1/DENSE PANICLE2 or SMALL AND ROUND SEED3 produces shorter and smaller grain due to inhibited cell elongation (Huang et al., 2009; Abe et al., 2010; Kitagawa et al., 2010). In contrast, SLENDER GRAIN7, which increases cell length longitudinally and decreases cell width transversely, acts independently of cell division (Zhou et al., 2015). SQUAMOSA PROMOTER BINDING PROTEIN-LIKE 13 positively regulates cell size in the grain hull, resulting in enhanced grain length and yield (Si et al., 2016). In some instances, both cell number and size are involved in the development of hull size. For example, BIG GRAIN 1 andGRAIN SIZE 5 (GS5) simultaneously affect cell number and size by acting as positive regulators of grain size. GS5 increases cell number and, to some extent, expands cell size, leading to enhanced latitudinal growth of grain (Li et al., 2011). Overexpression of BG1 increases both cell number and area of the spikelet hull, resulting in wider and longer grains (Liu et al., 2015).
The ubiquitin-proteasome pathway has been shown to play a crucial role in regulating organ size, including seed shape and size in plants (Song et al., 2007; Li et al., 2012; Huang et al., 2017; Wang et al., 2017b). Ubiquitin can be attached to appropriate substrates and processed by an ATP-dependent E1 (ubiquitin-activating enzyme)-E2 (ubiquitin-conjugating enzyme)-E3 (ubiquitin ligases) enzyme conjugation cascade (Sadanandom et al., 2012). Similar to many other biochemical reactions, ubiquitination is also a reversible process. Ubiquitin added to substrates can also be cleaved by deubiquitinating enzymes (DUBs) to modify the ubiquitination of substrate protein. Several studies have demonstrated the importance of DUBs in regulating seed development. WIDE AND THICK GRAIN 1, encoding an otubain-like protease with deubiquitination activity, determines grain size and shape of rice by influencing cell expansion (Huang et al., 2017; Wang et al., 2017b). Arabidopsis (Arabidopsis thaliana) ubiquitin-specific protease 15 (AtUBP15)/SUPPRESSOR2 OF DA1, acting as a deubiquitination enzyme, regulates organ and seed size in Arabidopsis by promoting cell proliferation (Liu et al., 2008; Du et al., 2014).
In this study, we identified a dominant gain-of-function rice mutant, named large grain1-D (lg1-D), which developed significantly enlarged grains. LG1 encodes a OsUBP15 and possesses deubiquitination activity. Down-regulated expression of LG1 produced narrower and smaller grain, whereas overexpression of LG1 significantly enhanced grain width relative to the wild type. Our studies established the function of LG1/OsUBP15 as a positive regulator of grain width and size in rice.
RESULTS
Phenotypic Characterization of the lg1-D Mutant
The lg1-D mutant was obtained as a tissue culture-induced mutant of japonica rice variety Kitaake. Compared with the wild type, plant height of lg1-D was slightly decreased, and plant architecture was more upright and compact (Fig. 1, A and E). The panicle of lg1-D was also more compact, erect, and shorter than in the wild type (Fig. 1, B and F). However, lg1-D has increased grain size (Fig. 1C); grain width, length, and thickness were increased by 30.8%, 13.23%, and 13.8%, respectively (Fig. 1, G–I). As a result, the 1,000-grain weight was increased by 34.5% (Fig. 1J). The width, length, and thickness of brown grain were also significantly increased in lg1-D (Fig. 1D; Supplemental Table S1). We therefore investigated the grain-filling rate of the wild type and lg1-D by measuring dry matter accumulation in brown grains from 3 d after fertilization (Supplemental Fig. S1). lg1-D had significantly faster filling rate from 9 d after fertilization and higher dry weight from 12 d after fertilization compared with the wild type. Grain yield per plant of lg1-D was largely comparable with that of the wild type, although with a significantly decreased number of grains per panicle (Supplemental Table S1).
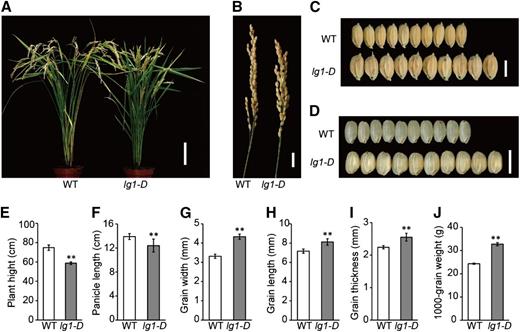
Phenotypic characterization of the lg1-D mutant. A, Wild-type (WT) and lg1-D mutant plants at the mature stage. Bar = 10 cm. B, Panicles of the wild type and lg1-D mutant. Bar = 1.5 cm. C, Grains of the wild type and lg1-D mutant. Bar = 5 mm. D, Brown rice of the wild type and lg1-D mutant. Bar = 5 mm. E to J, Comparisons of plant height (E), panicle length (F), grain width (G), grain length (H), grain thickness (I), and 1,000-grain weight (J) of the wild type and lg1-D mutant. All data are means ± sd (n = 20 in E–I and n = 3 in J). **, P < 0.01, determined by Student’s t test.
lg1-D Affects Grain Size by Influencing Cell Proliferation
The spikelet hull of the lg1-D mutant was wider and longer than that of the wild type before flowering (Fig. 2A). Scanning electron microscopy of the spikelet hull showed that epidermal cells of the lg1-D lemma were shorter than those of the wild type (Supplemental Fig. S2), indicating that increased grain length in the mutant resulted from the increase of cell number in the longitudinal direction in the spikelet hull. Cross-section observations of the middle part of the hull before flowering showed that lg1-D had a longer parenchyma cell layer compared with the wild type (Fig. 2, B, C, and F). The number of parenchyma cells in lg1-D was increased by 18.4%, whereas cell length was increased only by 5.1% (Fig. 2, G and H). These results suggested that the increased grain width in lg1-D mainly resulted from the increased cell number and partially from increased cell size. Supporting these results, expression levels of six cell cycle-related genes were significantly increased in young panicles of the lg1-D mutant (Fig. 2I). Thus, the increased grain size was caused by cell proliferation in the spikelet hull in both the latitudinal and longitudinal directions.
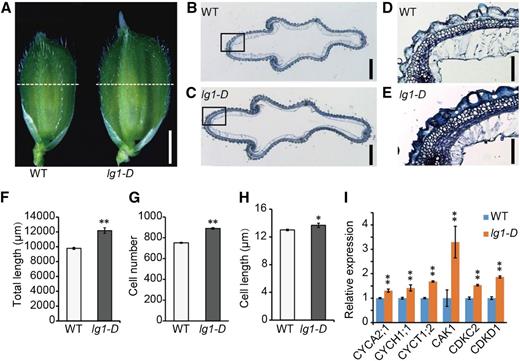
The lg1-D mutation affected grain size by increased cell proliferation. A, Spikelets of the wild type (WT) and lg1-D mutant prior to flowering. Dotted white lines indicate sites of cross sections in B and C. B and C, Cross sections of wild-type (B) and lg1-D mutant (C) spikelet hulls. Bars = 500 μm. D and E, Magnified images of the boxed areas in B and C, respectively. Bars = 50 μm. F to H, Comparison of total length (F) and cell number (G) of the spikelet hull and average length of each cell (H) in the outer parenchyma layer. All data are means ± sd (n = 10). **, P < 0.01 and *, P < 0.05, determined by Student’s t test. I, Transcript levels of cell cycle-related genes in 0.5-cm young panicles of lg1-D relative to the wild type. The rice Ubiquitin gene was used as an internal control. Data are means ± sd (n = 3). **, P < 0.01, determined by Student’s t test.
Molecular Cloning of the LG1 Gene
We performed a genetic analysis prior to cloning the LG1 gene. F1 plants from a wild type × lg1-D mutant cross had grains that were markedly wider than those of the wild type but slightly narrower than those of the lg1-D mutant parent, indicating that the lg1-D mutation was partially dominant (Supplemental Fig. S3, A and B). Segregation in the F2 population was 65 plants with normal (wild-type) grains, 110 intermediate, and 48 with larger grains, corresponding to a 1:2:1 ratio (χ2 = 2.63, χ2 0.05,2 = 5.99; Supplemental Fig. S3C). Gene mapping was performed using an F2 population derived from lg1-D mutant × indica variety Xieqingzao B. The LG1 locus was delimited to an 89-kb genomic region on rice chromosome 2S based on analysis of 569 plants with the recessive (wild-type) phenotype (Fig. 3A). The region contained six open reading frames (ORFs; Fig. 3A).
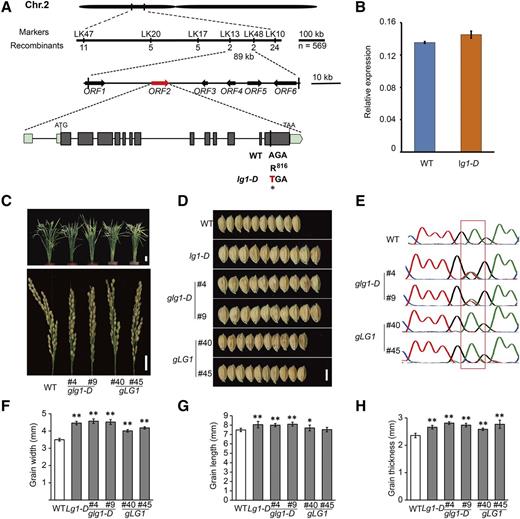
Map-based cloning of LG1. A, Fine-mapping of the lg1-D locus. Molecular markers and numbers of recombinants are labeled above and below the filled bars, respectively. The candidate ORF2 is marked in red. Exon/intron structure and the mutation site of ORF2 are also shown. ATG and TGA represent the start and stop codons, respectively. B, Expression levels of ORF2 in 1-cm-long young panicle of the wild type (WT) and lg1-D. The rice Ubiquitin gene was used as an internal control. Data are presented as means ± sd (n = 3). C to H, Phenotypic comparison of T3 homozygous glg1-D and gLG1 transgenic lines. C and D, Plant architecture (C, top), panicle (C, bottom), and grain (D) morphologies of transgenic lines. Bars = 10 cm (C, top), 2 cm (C, bottom), and 5 mm (D). E, Sequencing analyses of LG1 of transgenic lines in C. The box indicates the mutant site in lg1-D. F to H, Statistical analyses of grain width (F), length (G), and thickness (H) of transgenic lines. All data are means ± sd (n = 20 in F–H). **, P < 0.01 and *, P < 0.05, determined by Student’s t test.
A single-nucleotide substitution in the lg1-D mutant was identified in ORF2 (LOC_02g14730) by sequencing. The full-length complementary DNA (cDNA) of ORF2 obtained using 5′ and 3′ RACE showed that the coding region of ORF2 consisted of 13 exons and was 2,928 bp in length. The single-nucleotide substitution in lg1-D was located in the last exon and caused truncation of 160 amino acid residues at the C terminus (Fig. 3A). Quantitative reverse transcription (RT)-PCR analysis showed that the expression level of ORF2 in the lg1-D mutant was comparable with that in the wild type (Fig. 3B).
In order to verify that the single-nucleotide substitution in LOC_Os02g14730 was responsible for the grain phenotype of lg1-D, we transformed wild-type calli with genomic fragments of either the lg1-D mutant or wild-type LG1 alleles. Both constructs contained the putative coding sequence and regulatory region of LOC_Os02g14730. As expected, T3 transgenic plants carrying the homozygous lg1-D fragment had wider grains compared with wild-type plants and were overall phenotypically similar to the lg1-D mutant (Fig. 3, C–F). Overexpression of the wild-type LG1 allele in the wild-type background caused a moderate increase in grain width, length, and thickness (Fig. 3, D–H). These results suggested that LG1 acted positively in regulating the grain width of rice, and the dominant wider grain phenotype of the lg1-D mutant was likely caused by a gain-of-function mutation in LG1. To further confirm this hypothesis, we generated RNA interference (RNAi) transgenic lines in wild-type and lg1-D mutant backgrounds (Supplemental Fig. S4 and S5). As expected, reduced LG1 expression markedly decreased the grain width in both the wild-type and lg1-D backgrounds, accompanied by decreased grain length and thickness (Supplemental Fig. S4). Taken together, these results demonstrated that LG1 was a positive regulator of grain width in rice.
Sequence and Expression Analysis of the LG1 Gene
Sequence analysis showed that LG1 encodes a ubiquitin-specific protease containing a single ubiquitin carboxyl-terminal hydrolase (UCH) motif at the C terminus as well as myeloid, nervy, and DEAF1 (MYND)-type zinc finger (ZnF-MYND) motifs at the N terminus (Fig. 4A). Three genes (LOC_Os08g37350, LOC_Os09g28940, and LOC_Os06g44380) were identified as homologs of LG1 in the rice genome. Phylogenetic analysis showed that LG1 was most closely related to AtUBP15 in Arabidopsis and ZmUCH15 in maize (Zea mays; Supplemental Figs. S6 and S7). Therefore, the LG1 gene is hereafter referred to as OsUBP15 while the dominant mutant allele lg1-D is named Osubp15.
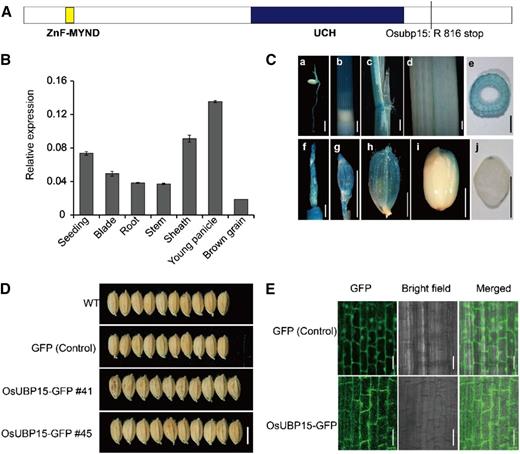
Expression pattern of OsUBP15 and subcellular localization of OsUBP15. A, Schematic domain structure of OsUBP15. OsUBP15 harbors a MYND-type zinc finger (ZnF-MYND) motif (in yellow box) at the N terminus and a UCH motif (in black box) at the C terminus. The line indicates the mutant site in Osubp15. B, Relative expression of OsUBP15 in various tissues including seedlings, leaf blades, stems, leaf sheaths, young panicles, and brown grain (3 DPA). The rice Ubiquitin gene was used as an internal control. Data are means ± sd (n = 3). C, GUS staining of pOsUBP15:GUS transgenic T1 plants. GUS activity was detected in roots at the germination stage (a), stems (b and e), sheaths (c), leaves (d), young panicles (f), glumes (g and h), and the caryopsis at the filling stage (i), the cross section of the caryopsis at filling stage (j). Bars = 5 mm (a) and 2 mm (b–j). D, Grain morphologies of the wild type (WT), empty GFP, and gOsUBP15-GFP transgenic T1 lines. Bar = 5 mm. E, Localization of OsUBP15-GFP in the cytoplasm and nucleus in young roots of a gOsUBP15-GFP transgenic T1 line. Bars = 20 μm.
We next investigated the spatial expression profile of OsUBP15 using quantitative RT-PCR and GUS staining. This gene was generally expressed in seedlings, leaf blades, roots, stems, sheaths, young panicles, and brown rice, with a pronounced expression in young panicles before heading (Fig. 4, B and C).
We generated gOsUBP15-GFP (green fluorescent protein) transgenic plants to analyze the subcellular localization of OsUBP15 in vivo. These plants produced wider and larger seeds than the wild-type and control GFP lines, indicating that gOsUBP15-GFP was biologically functional in transgenic plants (Fig. 4D). Fluorescence microscopy revealed that OsUBP15-GFP was mainly distributed in the cytoplasm and nucleus and was sporadically targeted to some unknown punctate compartments (Fig. 4E).
OsUBP15 Is a Functional Deubiquitination Enzyme
AtUBP15 was revealed to be a functional deubiquitination enzyme in Arabidopsis (Liu et al., 2008). To determine whether OsUBP15 and the truncated Osubp15 also possess deubiquitination activity, glutathione S-transferase (GST)-OsUBP15, GST-Osubp15, and GST alone were separately coexpressed with ubiquitin substrates His-UBQ1 (ubiquitin extension protein) or His-UBQ10 (hexameric polyubiquitin) in Escherichia coli. Immunoblot analysis showed that both OsUBP15 and Osubp15 were able to cleave His-UBQ1 and His-UBQ10 (Fig. 5, A and B). Thus, both OsUBP15 and Osubp15 had the deubiquitination activity in vitro. Our results also suggested that the C-terminal 160 amino acids were unnecessary for deubiquitination enzyme activity of OsUBP15.
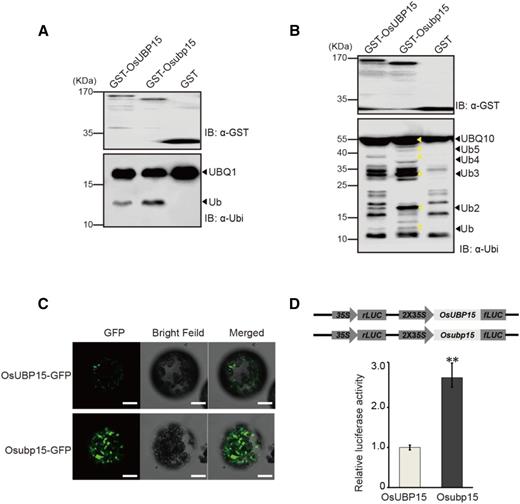
Characterization of OsUBP15 and Osubp15 proteins. A and B, Both OsUBP15 and Osubp15 cleaved substrates UBQ1 (A) and UBQ10 (B). Fused protein GST-OsUBP15 (lane 1A and B), GST-Osubp15 (lane 2A and B), and GST vector (lane 3A and B) were coexpressed with His-UBQ1 (A) and His-UBQ10 (B) in E. coli. IB, Immunoblot; Ub, ubiquitin. C, Fluorescence microscopy of OsUBP15-GFP and Osubp15-GFP in the N. benthamiana protoplasts that were isolated from the leaves at 3 d post agroinfiltration. Bars = 10 μm. D, Relative luciferase activity analysis of OsUBP15-LUC and Osubp15-LUC in wild-type rice protoplasts. OsUBP15 and Osubp15 were separately fused with firefly luciferase (fLUC) protein driven by 2×35S promoter; renilla luciferase (rLUC) was the reference protein. Data are presented as means ± sd (n = 3). **, P < 0.01.
Stability of the Osubp15 Protein Is Enhanced in the lg1-D Mutant
Genetic analysis and transgenic complementation tests verified the dominant nature of the Osubp15 mutant allele in lg1-D, and the deubiquitination assay further proved that Osubp15 retained deubiquitination enzyme activity. Previous studies showed that the C termini of proteins often play important roles in the regulation of protein stability (Maurice et al., 2001; Sun et al., 2018). Transient expression of P35S:OsUBP15-GFP and P35S:Osubp15-GFP was performed in leaf epidermal cells of Nicotiana benthamiana. The results showed that the mutant Osubp15-GFP fusion protein produced stronger fluorescence signals than the wild-type OsUBP15-GFP fusion protein, indicating that Osubp15-GFP might be more stable than OsUBP15-GFP (Fig. 5C). Quantitative luciferase activity assays in rice protoplast also further demonstrated that Osubp15-LUC fusion protein indeed had higher abundance than OsUBP15-LUC (Fig. 5D).
OsUBP15 Interacts with OsDA1
According to Du et al. (2014), Arabidopsis DA1 protein, containing two ubiquitin interaction motifs and a single zinc-binding LIM (Lin-1, Isl-1 and Mec-3) domain, could bind with the C terminus of AtUBP15 and thereby reduce its stability. We wondered if OsUBP15 could interact with DA1 homologs in rice. Four proteins (LOC_Os06g08400, LOC_Os12g40490, LOC_Os03g42820, and LOC_Os03g16090) sharing significant homology with DA1 were identified in rice. Phylogenetic analysis showed that LOC_Os06g08400 and DA1 were grouped into one branch; hence, the former was named OsDA1 (Fig. 6A). Like Arabidopsis DA1, OsDA1 showed high affinity to ubiquitin (Fig. 6B). Yeast two-hybrid assays indicated that OsDA1 physically interacted with both wild-type OsUBP15 and mutant Osubp15, although the mutant combination (OsDA1-Osubp15) appeared to be slightly weaker (Fig. 6C). The interactions were further confirmed by in vivo firefly luciferase complementation imaging assays (Fig. 6D).
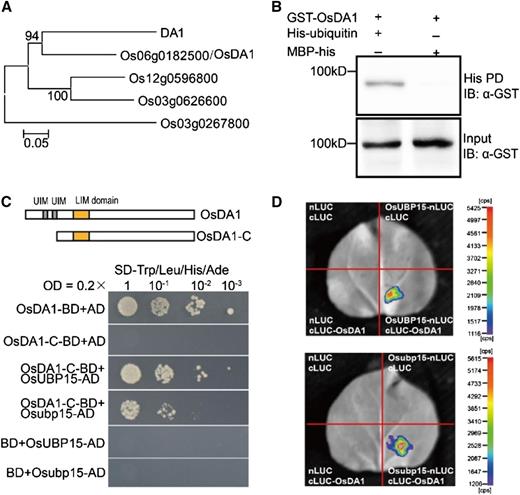
OsUBP15 physically interacted with OsDA1. A, Phylogenetic analysis of DA1 and DA1-like proteins in rice. The phylogenetic tree was constructed using the neighbor-joining method in MEGA 5.1. Bootstrap values are shown as percentages at the nodes. The scale bar at the bottom represents genetic distance. B, OsDA1 interacted with ubiquitin in vitro. OsDA1 expressed as a GST fusion protein in E. coli was pulled down (PD) by His-ubiquitin immobilized on Ni2+- nitrilotriacetic acid agarose and analyzed by immunoblotting (IB) using an anti-GST antibody. His protein fused with maltose-binding protein (MBP) was used as a negative control. C, Both OsUBP15 and Osubp15 interacted with OsDA1 in yeast. Yeast transformants were spotted on synthetic dropout (SD) selective medium (SD-Trp/Leu/His/Ade). AD, Activation domain; BD, binding domain; LIM, single zinc-binding LIM domain; OsDA1, full-length OsDA1 protein; OsDA1-C, C terminus of OsDA1 protein; UIM, ubiquitin interaction motifs. D, Firefly luciferase complementation imaging assay to detect physical interaction between OsDA1 and OsUBP5 (up) and Osubp15 (down) in leaf epidermal cells of N. benthamiana. Colored scale bars indicate the luminescence intensity in counts per second (cps). cLUC, C terminus of LUC; nLUC, N terminus of LUC.
OsUBP15/LG1 and GW2 Are Not Independent in Regulating Grain Width in Rice
Considering that OsUBP15 is a functional deubiquitination enzyme and that GW2 functions as a RING-type E3 ubiquitin ligase to negatively regulate grain size, we investigated their genetic relationship. Using CRISPR/CAS9, we generated single-locus loss-of-function mutants in GW2 (lines gw2-1 and gw2-2) and OsUBP15/LG1 (lines lg1-1 and lg1-2) as well as in LG1/GW2 double knockout mutants (lines lg1-2 gw2-4 and lg1-3 gw2-3; Fig. 7A). GW2 was also knocked out in the lg1-D mutant background to obtain pyramided lg1-D gw2 plants. Consistent with a previous study, knockout of GW2 generated wider and larger grain than in the control (Song et al., 2007; Fig. 7). As a positive regulator of grain size, the knockout mutant of LG1 showed reduced grain width, length, and thickness. LG1/GW2 double knockout mutants displayed an intermediate phenotype; the grains were wider than in lines lg1-1 and lg1-2 but narrower than in lines gw2-1 and gw2-2 (Fig. 7). Interestingly, knockout of LG1 in the gw2 mutant background caused an 8.3% decrease in grain width, whereas knockout of LG1 in the Kitaake background led to a more dramatic 16.5% reduction (Fig. 7, C–F). In contrast, the lg1-D gw2 double mutant displayed a 32.4% increase in grain width relative to Kitaake, whereas the single mutations of either gw2 or lg1-D increased grain width in Kitaake by 17.2% and 26.51%, respectively (Fig. 7, C–F). Hence, the genetic effect from the lg1-D gw2 double mutant was slightly less than the additive effect derived from gw2 and lg1-D. These overall results suggested that LG1 and GW2 are not completely independent in regulating grain width in rice.
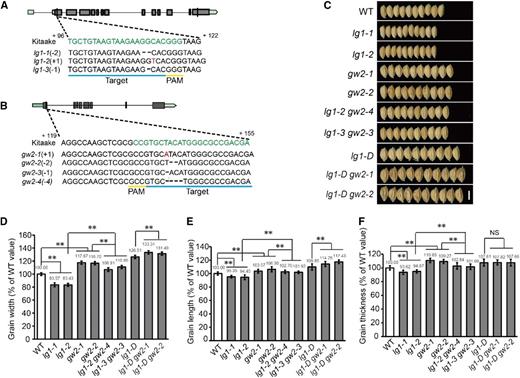
LG1 was not independent of GW2 in regulating grain size in rice. A, Diagram of the CRISPR target fragment (in green) in the first exon of LG1 and three lg1 alleles. The position of the protospacer adjacent motif sequence is underlined (in yellow). Plus and minus signs indicate base insertion (in red) and deletion (by hyphen), respectively, relative to Kitaake. Gray box denotes coding sequence of LG1, and green box is the untranslated region. B, Diagram of the CRISPR target fragment (in green) in the first exon of GW2 and three gw2 alleles detected. The position of the PAM sequence is underlined (in yellow). Plus and minus signs indicate base insertion (in red) and deletion (by hyphen), respectively, relative to Kitaake. Gray box denotes coding sequence of GW2, and green box is the untranslated region. C, Grains of the wild type (WT), lg1, gw2, lg1 gw2, lg1-D, and lg1-D gw2. Bar = 0.5 cm. D to F, Comparison of grain width (D), grain length (E), and grain thickness (F) of the wild type, lg1, gw2, lg1 gw2, lg1-D, and lg1-D gw2. All data are means ± sd (n = 20). **, P < 0.01; and NS, no significance, determined by Student’s t test.
DISCUSSION
OsUBP15 Positively Regulates Rice Grain Width and Size by Influencing Cell Proliferation
Grain weight, a key factor in the determination of grain yield in rice, is positively correlated with grain width, length, and thickness (Tan et al., 2000). In this study, we isolated a dominant and functional lg1-D mutant with wider and larger grain and identified the causative gene, LG1, which encodes a ubiquitin-specific protease15 with deubiquitination activity in vitro. Cytological observations of the middle part of the hull prior to flowering showed that the increased grain size in the lg1-D mutant resulted from an increase in cell number in the latitudinal direction (Fig. 2). In previous studies, a number of genes regulating grain size in rice were shown to influence cell proliferation in the latitudinal or longitudinal direction of spikelet hulls (Li et al., 2012; Wang et al., 2012, 2015; Duan et al., 2014; Song et al., 2015). It was reported that Arabidopsis UBP15 protein also promotes cell proliferation (Liu et al., 2008). Thus, OsUBP15 has a similar role in rice. Overexpression of OsUBP15 enhanced grain width and size (Fig. 3, D–H), whereas down-regulation led to narrower and smaller grain (Supplemental Fig. S4, F–J). Collectively, OsUBP15 positively regulates grain width and size by affecting cell proliferation in the spikelet hull.
Enhanced Accumulation and Stronger DUB Activity of Osubp15 May Be Responsible for the Phenotype of lg1-D
The C termini of proteins often play important roles in negative regulation of protein activity or stability (Maurice et al., 2001; Chiara et al., 2004; Wang et al., 2005; Mao et al., 2010; Sun et al., 2018). For example, deletion of the last 46 residues at the C terminus of the platelet-derived growth factor β-receptor increases its receptor kinase activity (Chiara et al., 2004). Brassinosteroid insensitive 1 is a protein involved in the phosphorylation of C-terminal Ser/Thr residues whose kinase activity is also regulated by its C-terminal domain (Wang et al., 2005). In GS3, a protein regulating grain and organ size in rice, deletion of the C terminus enhanced the protein stability (Mao et al., 2010; Sun et al., 2018). Similarly, C-terminal truncation of Smad4 protein in pancreatic tumors led to decreased stability by preventing Smad4 homomeric and heteromeric complex formation (Maurice et al., 2001). In this study, knockdown of Osubp15 in the lg1-D mutant decreased the grain size (Supplemental Fig. S4, A–E), suggesting that Osubp15 protein was functional in vivo. An in vitro deubiquitination assay also showed that the Osubp15 protein retained its deubiquitination enzyme activity (Fig. 5, A and B). Transgenic plants carrying the lg1-D fragment produced significantly wider grains than those with the wild-type LG1 fragment (Fig. 3, D, E, and G), indicating that lg1-D is a dominant gain-of-function mutant. The Osubp15 protein in lg1-D had a deletion of 160 amino acid residues at the C terminus (Fig. 4A) and was more stable than OsUBP15 (Fig. 5, C and D). Evidence from Arabidopsis showed that DA1 protein specially recognizes the C terminus of UBP15, leading to its proteasomal degradation (Du et al., 2014). Thus, the increased stability of the truncated Osubp15 relative to the wild type might in part result from weaker interaction between Osubp15 and OsDA1 (Fig. 6C). It seems that Osubp15 has a stronger DUB activity than OsUBP15, since the intensity of ubiquitin monomer cleavage from UBQ1 was stronger in the reaction with GST-Osubp15 than with GST-OsUBP15 (Fig. 5B). It is therefore plausible that the dominant gain-of-function phenotype of the lg1-D mutant may result from enhanced accumulation and increased DUB activity of Osubp15.
OsUBP15/LG1 and GW2 Are Not Independent in Regulating Grain Width in Rice
GW2 is a RING-type E3 ubiquitin ligase that can be self-ubiquitinated. It negatively regulates grain width and weight by restricting cell division (Song et al., 2007). We investigated the genetic interaction between OsUBP15 and GW2. Genetic analysis of OsUBP15/LG1 and GW2 indicated that the two genes are not completely independent in the regulation of grain width (Fig. 7). It is possible that GW2 and OsUBP15 share downstream substrates during ubiquitination and deubiquitination, in addition to their specific roles. Although several ubiquitin-proteasome pathway-related factors influencing grain size in rice have been characterized (Song et al., 2007; Li et al., 2012; Huang et al., 2017; Wang et al., 2017a, 2017b), much more work is needed to determine how grain size is regulated by the ubiquitin-proteasome pathway.
In conclusion, we identified a dominant lg1-D mutant with wider and larger grain than its wild type. LG1 encodes OsUBP15, which is homologous to AtUBP15 and possesses deubiquitination activity. Our combined genetic and biochemical data support the notion that LG1 acts positively in regulating grain size in rice, and its role is only partially independent of the GW2. Pyramiding of lg1-D and gw2 could be a promising strategy to enhance yield in rice.
MATERIALS AND METHODS
Plant Materials
The lg1-D mutant with wider grains was isolated from tissue culture of japonica rice (Oryza sativa) variety Kitaake. The plants were grown in an experimental field under natural long-day conditions in Beijing.
Map-Based Cloning of LG1
F2 populations were generated from crosses lg1-D mutant × Kitaake and lg1-D mutant × Xieqingzao B (an indica variety) for genetic analysis and map-based cloning of LG1, respectively. The LG1 gene was mapped to an interval between insertion/deletion markers LK13 and LK48 on chromosome 2S. Molecular markers are listed in Supplemental Table S2. Subsequent sequence analysis showed that a single-nucleotide substitution in ORF2 in the lg1-D mutant was present in the last exon. The full-length cDNA of ORF2 was amplified using a SMARTer PCR cDNA Synthesis Kit (Clontech) following the manufacturer’s instructions.
Vector Construction and Transformation
To generate gLG1 and glg1-D constructs, the genomic fragment spanning the putative coding sequence and regulatory region of LOC_02g14730 was cloned from genomic DNA of the wild type and the lg1-D mutant. The genomic fragments were separately cloned into vector pCAMBIA-1305.1 (Gao et al., 2014). Plants with knockdown expression of LG1 were generated by the vector pCUbi1390-FAD2 (Tan et al., 2014). Primers used to amplify the antisense and sense versions of the specific 153-bp fragment from the coding region are listed in Supplemental Table S2. To generate the loss-of-function mutants of LG1 and GW2, 20-bp gene-specific spacer sequences of target genes (Supplemental Table S2) were first cloned into the entry vector pOs-sgRNA and then inserted into binary vectors containing the CAS9 expression cassette using Invitrogen’s Gateway LR Clonase II Enzyme mix (Miao et al., 2013). Recombinant plasmids were transformed into wild-type or lg1-D plants by Agrobacterium tumefaciens-mediated transformation as described previously (Hiei et al., 1994).
RNA Extraction and Quantitative RT-PCR
Total RNA was extracted from different rice tissues using an RNA Prep Pure Kit (Zymo Research), and the RNA was reverse transcribed with the QuantiTect Reverse Transcription Kit (Qiagen) following the manufacturer’s protocol. We carried out the quantitative RT-PCR on the ABI 7500 with SYBR Premix Ex Taq Kit (Takara; RR041A) according to the operation manual. We analyzed the data from three biological replicates referring to the relative quantification method (Livak and Schmittgen, 2001).
GUS Staining
To analyze the promoter activity of OsUBP15, the promoter region, an about 3.6-kb fragment upstream of the translation start codon of OsUBP15, was cloned into the pCAMBIA-1305.1 vector to create a PROOsUBP15:GUS construct. The recombined vector was transformed into the wild type by an A. tumefaciens-mediated method (Hiei et al., 1994). GUS staining was performed using T1 generation transgenic plants (Jefferson et al., 1987).
Subcellular Localization of OsUBP15
The 9,697-bp genomic sequence containing the promoter region and the OsUBP15 gene without a stop codon was amplified to the upstream of the GFP coding region of the pCAMBIA1305-GFP vector (Ren et al., 2014) to generate the vector gOsUBP15-GFP. The vectors gOsUBP15-GFP and pCAMBIA1305-GFP (control) were transformed into the wild type. The young roots of transgenic plants were analyzed using a Leica TCS SP5 laser scanning confocal microscope.
Transient Expression in Nicotiana benthamiana
We constructed two binary vectors, pCAMBIA1305-OsUBP15-GFP and pCAMBIA1305-Osubp15-GFP, expressing OsUBP15-GFP and Osubp15-GFP fusions, respectively, and then introduced them into the A. tumefaciens strain EHA105. The transformed A. tumefaciens was used to infiltrate N. benthamiana leaves, according to a previous description (Waadt and Kudla, 2008). Prior to fluorescence observations, we isolated N. benthamiana protoplasts as described previously (Ren et al., 2014).
In Vitro Deubiquitination Assays
The coding sequences of OsUBP15 and osubp15 were cloned into vector pGEX4T-1, constructing MBP-OsUBP15 and MBP-osubp15 plasmids, respectively. The coding sequences of UBQ1 and polyubiquitin UBQ10 were cloned into the vector pET-28a-c (+) to construct His-UBQ1 and His-UBQ10 vectors, respectively. The two substrates, His-UBQ1 and His-UBQ10, were coexpressed with GST-OsUBP15, GST-osubp15, and GST in Transetta (DE3) cells under modified inducible conditions (37°C for 2 h and 16°C for 3 h). Lysates were analyzed by western blots with anti-GST (Medical and Biological Laboratories) and anti-ubiquitin (Cell Signaling) antibodies.
Luciferase Activity Assays
The coding regions of OsUBP15 and Osubp15 were cloned into the vector pGreenII 0800-LUC with the firefly luciferase gene (LUC) to generate OsUBP15-LUC and Osubp15-LUC under the control of the Cauliflower mosaic virus 35S promoter. These were transiently expressed in rice protoplasts. We performed relative luciferase activity assays according to the previous description (Zhou et al., 2013).
Yeast Two-Hybrid Assays
The coding regions of OsUBP15 and Osubp15 were cloned into pGADT7 (Clontech), while full-length and truncated OsDA1 were cloned into pGBKT7. Yeast transformation was performed according to the manufacturer’s instructions.
Firefly Luciferase Complementation Imaging Assays
The coding regions of OsUBP15 and Osubp15 were cloned into the pCAMBIA-NLuc vectors, and the coding region of OsDA1 was cloned into the vector fused downstream of C-Luc in the pCAMBIA-CLuc vector. The assays were performed according to the previous description (Hua et al., 2012).
Ubiquitin-Binding Assays
The fusion proteins GST-OsDA1, His-ubiquitin, and MBP-His were expressed in Transetta (DE3) cells. Ubiquitin-binding assays were performed as published (Peng et al., 2015).
Supplemental Data
The following supplemental materials are available.
Supplemental Figure S1. Grain filling in wild-type and lg1-D mutant seeds.
Supplemental Figure S2. Scanning electron microscopy of the papilla of the lemma in the wild type and the lg1-D mutant.
Supplemental Figure S3. Genetic analysis of the lg1-D mutant.
Supplemental Figure S4. LG1 RNAi transgenic T3 plants exhibit small grain size in lg1-D and wild-type backgrounds.
Supplemental Figure S5. Plant architecture and panicle performance of LG1 RNAi transgenic T3 plants.
Supplemental Figure S6. Phylogenetic analysis of the OsUBP15 subfamily and its homologs.
Supplemental Figure S7. Alignment of OsUBP15 and AtUBP15.
Supplemental Table S1. Agronomic trait measurements for wild-type and lg1-D mutant plants.
Supplemental Table S2. Primers used in this study.
LITERATURE CITED
Author notes
This work was supported by the National Natural Science Foundation of China (91735304, 31430008, and 31571629), the National Transformation Science and Technology Program (2016ZX08001006 and 2016ZX08009-003), and the Fundamental Research Funds for Excellent Young Scientists of CAAS (Y2017JC02 to Y.R.) as well as by the Key Laboratory of Biology, Genetics, and Breeding of Japonica Rice in Mid-lower Yangtze River, the Ministry of Agriculture, China, and the Jiangsu Collaborative Innovation Center for Modern Crop Production.
These authors contributed equally to the article.
Authors for contact: [email protected].
Senior author
The author responsible for distribution of materials integral to the findings presented in this article in accordance with the policy described in the Instructions for Authors (www.plantphysiol.org) is: Jianmin Wan ([email protected]).
J.M.W., Y.R., C.S., and H.Q.Z. conceived the project and designed the experiments; Z.C., C.L., J.L.W., Z.Z., and J.W. screened the mutant materials; C.S., Y.R., F.W., and H.Z. performed the subcellular localization assay; P.T. and T.L. constructed some vectors; C.S., T.P., Y.F.W., and R.N.J. performed all other experiments; F.Q.W., Q.L., X.Z., S.Z., and X.G. provided technical support; C.S., Y.R., and L.L. wrote and revised the article.
Articles can be viewed without a subscription.