-
PDF
- Split View
-
Views
-
Cite
Cite
Cong Wang, Jiyue Huang, Jun Zhang, Hongkuan Wang, Yapeng Han, Gregory P. Copenhaver, Hong Ma, Yingxiang Wang, The Largest Subunit of DNA Polymerase Delta Is Required for Normal Formation of Meiotic Type I Crossovers, Plant Physiology, Volume 179, Issue 2, February 2019, Pages 446–459, https://doi.org/10.1104/pp.18.00861
- Share Icon Share
Abstract
Meiotic recombination contributes to the maintenance of the association between homologous chromosomes (homologs) and ensures the accurate segregation of homologs during anaphase I, thus facilitating the redistribution of alleles among progeny. Meiotic recombination is initiated by the programmed formation of DNA double strand breaks, the repair of which requires DNA synthesis, but the role of DNA synthesis proteins during meiosis is largely unknown. Here, we hypothesized that the lagging strand-specific DNA Polymerase δ (POL δ) might be required for meiotic recombination, based on a previous analysis of DNA Replication Factor1 that suggested a role for lagging strand synthesis in meiotic recombination. In Arabidopsis (Arabidopsis thaliana), complete mutation of the catalytic subunit of POL δ, encoded by AtPOLD1, leads to embryo lethality. Therefore, we used a meiocyte-specific knockdown strategy to test this hypothesis. Reduced expression of AtPOLD1 in meiocytes caused decreased fertility and meiotic defects, including incomplete synapsis, the formation of multivalents, chromosome fragmentation, and improper segregation. Analysis of meiotic crossover (CO) frequencies showed that AtPOLD1RNAi plants had significantly fewer interference-sensitive COs than the wild type, indicating that AtPOL δ participates in type I CO formation. AtPOLD1RNAi atpol2a double mutant meiocytes displayed more severe meiotic phenotypes than those of either single mutant, suggesting that the function of AtPOLD1 and AtPOL2A is not identical in meiotic recombination. Given that POL δ is highly conserved among eukaryotes, we hypothesize that the described role of POL δ here in meiotic recombination likely exists widely in eukaryotes.
Meiosis produces four haploid gametes and is essential for eukaryotic sexual reproduction. One of the critical events during meiotic prophase I is meiotic recombination, which contributes to crossover (CO) formation, thereby ensuring the proper segregation of homologous chromosomes and increasing genetic variation among progeny (Mercier et al., 2015; Lambing et al., 2017; Wang and Copenhaver, 2018). Meiotic recombination is initiated by DNA double strand breaks (DSBs) generated by the topoisomerase-like SPORULATION11 (SPO11), as described in the double strand break repair (DSBR) model (Bergerat et al., 1997; Villeneuve and Hillers, 2001). Repair of DSBs leads to the formation of COs or noncrossovers (Allers and Lichten, 2001; Börner et al., 2004). Many organisms possess two types of COs: interference-sensitive COs (type I), which are dependent on the ZMM proteins (ZIP4, MSH4, MSH5, MER3, HEI10, etc.; Higgins et al., 2004); and interference-insensitive COs (type II), which depend on other enzymes such as MUS81 (Berchowitz et al., 2007) and FANCD2, a homolog of the Fanconi anemia D2 gene product (Kurzbauer et al., 2018).
Meiosis-associated DNA synthesis occurs during both meiotic DNA replication and the repair of meiotic DSBs. The former is largely similar to mitotic DNA replication and uses the DNA replication machinery, including DNA Polymerase α (POL α) and POL δ, both of which are required for lagging strand synthesis, while DNA POL ε is required for leading strand synthesis, as well as proliferating cell nuclear antigen (PCNA) and DNA Replication Factor1 (RFC1; Strich, 2004). Due to the essential role of DNA replication in the mitotic cell cycle, null alleles of these genes cause embryonic lethality. Consequently, the current understanding of their functions in meiosis is limited. At replication forks of Saccharomyces cerevisiae, replisome components recruit Dbf4-dependent Cdc7 kinase to phosphorylate Mer2, which is required for DSB formation, implying an association between DNA replication and meiotic recombination (Murakami and Keeney, 2014). Another factor, CDC45, is associated with the minichromosome maintenance protein complex and facilitates the initiation of replication by recruiting POL α (Kelly and Brown, 2000). Consistent with these observations, knockdown of the Arabidopsis (Arabidopsis thaliana) CDC45 homolog results in reduced fertility and SPO11-1-independent meiotic chromosome fragmentation, suggesting a role for CDC45 in meiotic replication (Stevens et al., 2004).
Recent studies, including those in Arabidopsis, have advanced our understanding of meiotic DSB-dependent DNA synthesis (Terasawa et al., 2007; Lu et al., 2012; Wang et al., 2012; Huang et al., 2016; Wang and Copenhaver, 2018). Replication protein A, a single-stranded DNA (ssDNA)-binding protein, is thought to protect DSB ends and plays an important role in meiotic recombination in both Arabidopsis and rice (Oryza sativa; Wang and Haber, 2004; Chang et al., 2009; Osman et al., 2009; Aklilu et al., 2014). A mutation in the budding yeast (S. cerevisiae) POL δ was shown to cause shorter gene conversion tracts and reduced meiotic CO frequency (Maloisel et al., 2004, 2008). In Arabidopsis, AtRFC1, AtPOL α, and AtPOL2A (the largest subunit of POL ε) are required for meiotic recombination with different roles (Wang et al., 2012; Huang et al., 2015, 2016; Osman et al., 2018). Meiosis-specific knockdown of each of these three genes causes chromosome fragmentation, indicative of a defect in meiotic DSB repair. Specifically, a C-terminal truncation of AtRFC1 results in the reduction of type I COs (Wang et al., 2012; Liu et al., 2013), while a hypomorphic mutation in the N-terminal region of POL2A causes a redistribution of COs among pathways (Huang et al., 2015). A nonlethal missense mutant of AtPOL α, icu2-1, has pleiotropic phenotypes, including SPO11-1-dependent chromosome fragmentation and bivalent entanglement at metaphase I (Osman et al., 2018). It is important to note that the identification of hypomorphic and/or conditional alleles has been a powerful tool for studying the role of essential genes that function during meiotic DNA synthesis. In yeast mitosis, leading strand synthesis is mediated by Pol ε, while Pol δ facilitates lagging strand synthesis (Nick McElhinny et al., 2008). However, under stress conditions, or when Pol ε is compromised, Pol δ also can carry out leading strand synthesis (Kunkel and Burgers, 2008). Therefore, we hypothesize that Pol δ also can replace Pol ε to promote leading strand synthesis during meiotic recombination.
To test the role of AtPOL δ in meiotic recombination, we examined an Arabidopsis T-DNA insertion allele of AtPOLD1, which encodes the catalytic subunit of POL δ, and found that it caused embryonic lethality, consistent with previous findings (Schuermann et al., 2009). To bypass the lethality hurdle, we used meiosis-specific knockdown of POL δ and found that AtPOLD1RNAi meiocytes had significantly lower expression of AtPOLD1 compared with the wild type. AtPOLD1RNAi plants had reduced fertility and meiotic defects, including incomplete synapsis, chromosome fragmentation, and unequal segregation in both anaphase I and II. We demonstrated that AtPOLD1 is important for the formation of type I COs. We also observed a synergistic phenotype in AtPOLD1RNAi atpol2a double mutants, suggesting that these polymerases have different roles during meiotic recombination. Taken together, these results strongly support the idea that meiotic recombination requires DNA lagging strand synthesis factors.
RESULTS
Characteristics of POLD1 in Arabidopsis
Arabidopsis, like animals and fission yeast, has four putative POL δ subunits (POLD1–POLD4), including the catalytic subunit POLD1 (Tahirov, 2012). Phylogenetic analysis of POLD1 homologs from representative fungi, plants, and animals shows three distinct clades that each include genes from plants, animals such as human (Homo sapiens), mouse (Mus musculus), nematode (Caenorhabditis elegans), and Drosophila melanogaster, and fungi (S. cerevisiae; Supplemental Fig. S1). Notably, POLD1 is highly conserved as a single copy among the species we examined, except for soybean (Glycine max) and Physcomitrella patens, which each have two copies, probably due to lineage-specific genome duplications. This phylogenetic analysis suggests functional conservation of POL δ among the major eukaryotic groups during evolution, with diversification of POL δ mainly following speciation. We examined our previously published Arabidopsis male meiocyte transcriptome data and found that POLD1, POLA1, and POL2A, which encode catalytic subunits of POL δ, POL α, and POL ε, respectively, have comparable expression levels compared with seedlings (Supplemental Table S1; Yang et al., 2011). POLD1 exhibits the highest expression compared with the other replicative polymerase subunits (Supplemental Table S1). Among these genes, we previously demonstrated meiotic functions for AtPOLA1 and AtPOL2A (Huang et al., 2015, 2016); however, the role of AtPOLD1 in meiosis has not been studied.
Meiosis-Specific Knockdown of AtPOLD1 Causes Reduced Fertility
As described above, AtPOLD1 is essential for plant growth and development (Schuermann et al., 2009). We adopted a meiosis-specific RNA interference (RNAi) approach (Supplemental Fig. S2) to study its function during meiosis. We created a total of 47 independent transgenic lines in which the RNAi transcript is expressed under the control of the meiosis-specific DMC1 promoter (pDMC1::AtPOLD1RNAi; Klimyuk and Jones, 1997). These transgenic lines have varying levels of reduced fertility. We performed reverse transcription quantitative PCR (RT-qPCR) to examine AtPOLD1 expression in stage 4 to 7 anthers of each transgenic plant and selected two representative independent T2 lines (AtPOLD1RNAi-1 and AtPOLD1RNAi-2) that had significantly reduced expression for further analyses (Supplemental Fig. S3C).
Phenotypic analyses showed that vegetative development was indistinguishable in AtPOLD1RNAi-1 and AtPOLD1RNAi-2 plants compared with the wild type, except for obviously reduced fertility (Fig. 1A). The siliques of AtPOLD1RNAi-1 and AtPOLD1RNAi-2 were shorter than wild-type siliques and contained fewer seeds (Fig. 1, B and C). Alexander Red staining for pollen viability confirmed a significant reduction in viable pollen grains (P < 0.01, two-tailed Student’s t test) in AtPOLD1RNAi-1 (249 ± 42 per anther, n = 16) and AtPOLD1RNAi-2 (285 ± 40 per anther, n = 15) compared with the wild type (527 ± 48 per anther, n = 16; Fig. 1, D and E). Light microscope analysis of Toluidine Blue-stained tetrad-stage meiocytes showed that AtPOLD1RNAi plants generated polyads with variably sized microspores, in contrast to wild-type tetrads, which had four similarly sized microspores (Fig. 1F). The frequency of polyads in AtPOLD1RNAi-1 and AtPOLD1RNAi-2 was 41% (n = 578) and 39% (n = 475), respectively. As the presence of polyads is indicative of a meiotic defect, these data indicated that AtPOLD1 is required for male fertility and meiosis in Arabidopsis. Nonetheless, the residual pollen grains in both transgenic lines should have allowed pollination, which does not explain the dramatically reduced fertility observed in AtPOLD1RNAi, suggesting a potential defect of female development. To test this hypothesis, we reciprocally crossed the wild type with both AtPOLD1RNAi lines. When wild-type pistils were pollinated with pollen of AtPOLD1RNAi-1 and AtPOLD1RNAi-2, 43.9 and 41.5 seeds per silique were produced, respectively, compared with 51.7 seeds when using wild-type control pollen (Supplemental Fig. S4; Supplemental Table S2). Pollination of AtPOLD1RNAi-1 and AtPOLD1RNAi-2 pistils with wild-type pollen produced only 18.3 and 20.7 seeds per silique, respectively. Consistent with this observation, AtPOLD1RNAi-1 and AtPOLD1RNAi-2 pistils pollenated with wild-type pollen produced siliques with 21 and 22 undeveloped ovules, respectively (Supplemental Fig. S4; Supplemental Table S2). Taken together, these results suggest an important role of AtPOLD1 in both male and female reproductive development.
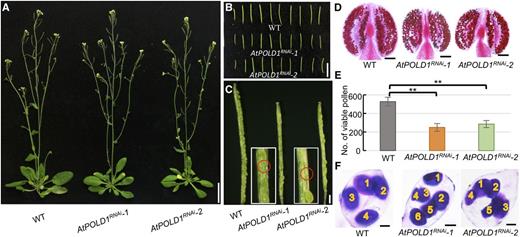
AtPOLD1RNAi plants display reduced fertility. A, Wild-type and two pDMC1::AtPOLD1RNAi F2 plants. B, The first 10 siliques of wild-type and AtPOLD1RNAi plants. C, Dissected siliques of the wild type and AtPOLD1RNAi. Red circles indicate undeveloped embryos. D, Alexander Red staining of wild-type and AtPOLD1RNAi anthers. E, Histogram of viable pollen in wild-type (n = 16), AtPOLD1RNAi-1 (n = 16), and AtPOLD1RNAi-2 (n = 15) anthers. Error bars represent sd for each group as described above. **, P < 0.01, two-tailed Student’s t test. F, Wild-type and AtPOLD1RNAi tetrad stage staining with Toluidine Blue. Bars = 3 cm (A), 1 cm (B), 1 mm (C), 0.1 mm (D), and 5 μm (F). WT, wild type.
AtPOLD1 Is Required for Normal Homolog Interaction during Meiosis
We examined chromosome spreads stained with 4′, 6-diamidino-2-phenylindole (DAPI) to test whether AtPOLD1RNAi plants have altered meiotic chromosome morphologies. AtPOLD1RNAi-1 and AtPOLD1RNAi-2 had similar chromosome phenotypes from leptotene to zygotene compared with the wild type (Fig. 2, A and B). At pachytene, an increased frequency of abnormal chromosome features, including bubbles and bifurcations, was observed in AtPOLD1RNAi-1 (38%, n = 47) and AtPOLD1RNAi-2 (44%, n = 36) compared with the wild type (10%, n = 49; Figs. 2C and 3A), suggesting that synapsis may be incomplete or delayed in AtPOLD1RNAi. At diakinesis in wild-type meiocytes (n = 56), the synaptonemal complex (SC) was disassembled and the chromosomes condensed to form five bivalents, which were organized by the spindle and aligned along the equatorial plane at metaphase I. In contrast, AtPOLD1RNAi-1 and AtPOLD1RNAi-2 meiocytes had abnormal multichromosome associations from diakinesis to metaphase I (Figs. 2, D and E, and 3, B and C). At diakinesis, 33% (27 of 83) of AtPOLD1RNAi-1 cells had no bivalent, another 30% (25 of 83) had one, 12% (10 of 83) had two, 16% (13 of 83) had three, and 10% (eight of 83) had five bivalents. AtPOLD1RNAi-2 meiocytes had a comparable distribution of bivalents (Supplemental Table S3). We also found multivalents at metaphase I in AtPOLD1RNAi with irregular alignment (Figs. 2E and 3C). At anaphase I, AtPOLD1RNAi-1 (40.6%, n = 32) and AtPOLD1RNAi-2 (43.2%, n = 37) had fragmented chromosomes and unequal chromosome segregation (Figs. 2, F and G, and 3, D–F), which is consistent with our observation of polyads and reduced fertility. We also observed aberrant chromosome phenotypes in female meiosis, including multivalents and chromosome fragmentation at metaphase I and anaphase I (Supplemental Fig. S5), consistent with the reduced female fertility (Fig. 1C; Supplemental Fig. S4). Together, these meiotic defects in AtPOLD1RNAi provide strong evidence that AtPOLD1 is essential for normal bivalent formation during meiotic prophase I.
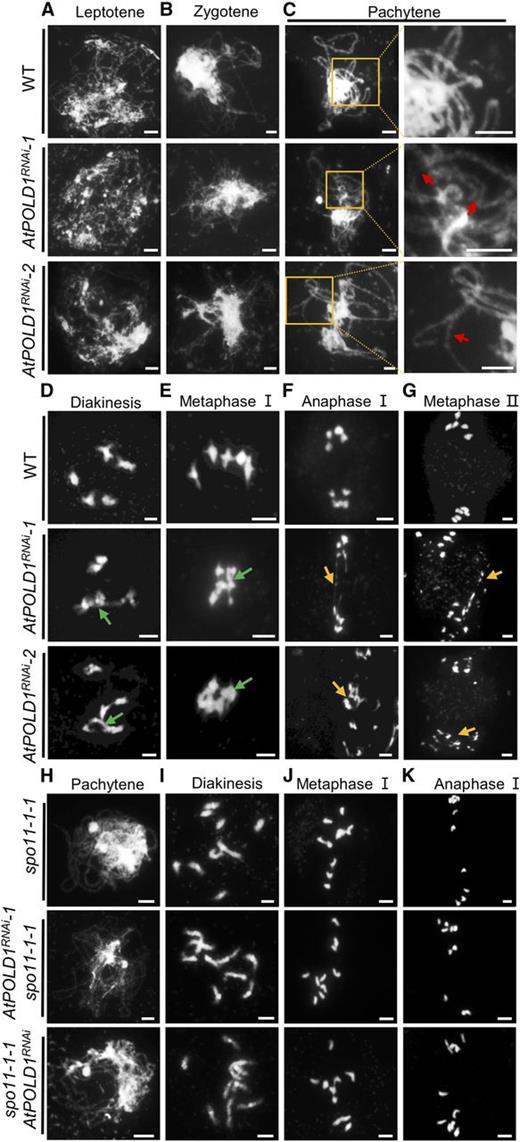
Male meiosis in the wild type and AtPOLD1RNAi assessed via chromosome spreads. A to G, Chromosome phenotypes of the wild type, AtPOLD1RNAi-1, and AtPOLD1RNAi-2 at leptotene (A), zygotene (B), pachytene (C), diakinesis (D), metaphase I (E), anaphase I (F), and metaphase II (G). H to K, Chromosome morphologies of spo11-1-1, AtPOLD1RNAi-1 spo11-1-1, and spo11-1-1 AtPOLD1RNAi. Red arrows indicate incomplete synapsis, green arrows indicate abnormal association of nonhomologs, and yellow arrows indicate chromosome fragments. In C, the regions of the yellow squares (left column) are enlarged in the right column. Bars = 5 μm. WT, wild type.
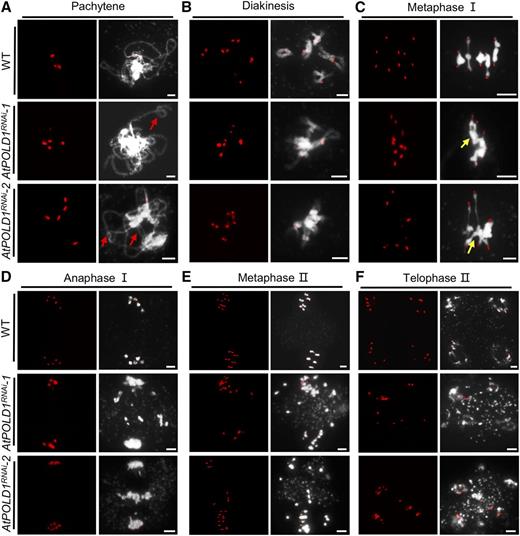
Meiotic chromosome morphologies of the wild type and AtPOLD1RNAi using fluorescence in situ hybridization with a centromere probe. Pachytene (A), diakinesis (B), metaphase I (C), anaphase I (D), metaphase II (E), and telophase II (F) are shown for the wild type, AtPOLD1RNAi-1, and AtPOLD1RNAi-2. Red arrows indicate incomplete synapsis and yellow arrows indicate the probable association of nonhomologs. Bars = 5 μm. WT, wild type.
The DMC1 promoter should be active after DSB formation, precluding the possibility that the observed meiotic defects, like chromosome fragmentation in AtPOLD1RNAi, are due to defects in meiotic DNA replication. To verify this, we analyzed spo11-1 AtPOLD1RNAi double mutants. We crossed AtPOLD1RNAi-1 with atspo11-1-1-/+ plants and identified atspo11-1-1−/− AtPOLD1RNAi-1 F2 progeny (designated AtPOLD1RNAi-1 spo11-1-1). We also transformed the pDMC1::AtPOLD1RNAi construct into atspo11-1-1−/+ plants and obtained 13 transgenic T1 atspo11-1-1−/− plants (designated spo11-1-1 AtPOLD1RNAi). The meiotic chromosome morphologies of AtPOLD1RNAi-1 spo11-1-1 (n = 50) and AtPOLD1RNAi-2 spo11-1-1 (n = 80) meiocytes were indistinguishable compared with the spo11-1-1 single mutant, which had 10 univalents and no chromosome fragmentation at metaphase I or anaphase I (Fig. 2, H–K). This result indicates that the chromosome fragmentation in AtPOLD1RNAi is dependent on DSBs produced by SPO11-1.
AtPOLD1 Is Important for Synapsis
As described above, the bubbles and bifurcations we observed on AtPOLD1RNAi pachytene chromosomes suggest a regional defect of synapsis. To test this hypothesis, we used immunofluorescence to examine the distribution of AtASY1, a primary axial protein and component of the SC lateral elements (Armstrong et al., 2002), and AtZYP1, a component of the SC transverse elements (Higgins et al., 2005). In the wild type, AtASY1 signals appeared to be continuous at leptotene (Fig. 4A) and extended linearly between homologous chromosomes at zygotene (Fig. 4B). At pachytene, AtASY1 began to disperse (Fig. 4C). AtZYP1 foci began to appear on chromosomes at leptotene, and they were entirely colocalized with the synapsed homologs by pachytene (Fig. 4, A–C). In AtPOLD1RNAi, the AtASY1 and AtZYP1 signals were similar to those of the wild type from leptotene to zygotene (Fig. 4, D and E). However, at pachytene, 41.5% (n = 82) of the AtPOLD1RNAi cells showed delayed dispersal of AtASY1 and discontinuous AtZYP1 signals (Fig. 4, F–I). Enlarged views of the chromosome regions with bubbles or bifurcations showed that they lacked AtZYP1 signal but had persistent AtASY1 signals. Some asynaptic regions lacked AtASY1 signal and had little or no AtZYP1 signal (Fig. 4, G and I), implying a partial defect of synapsis in AtPOLD1RNAi.
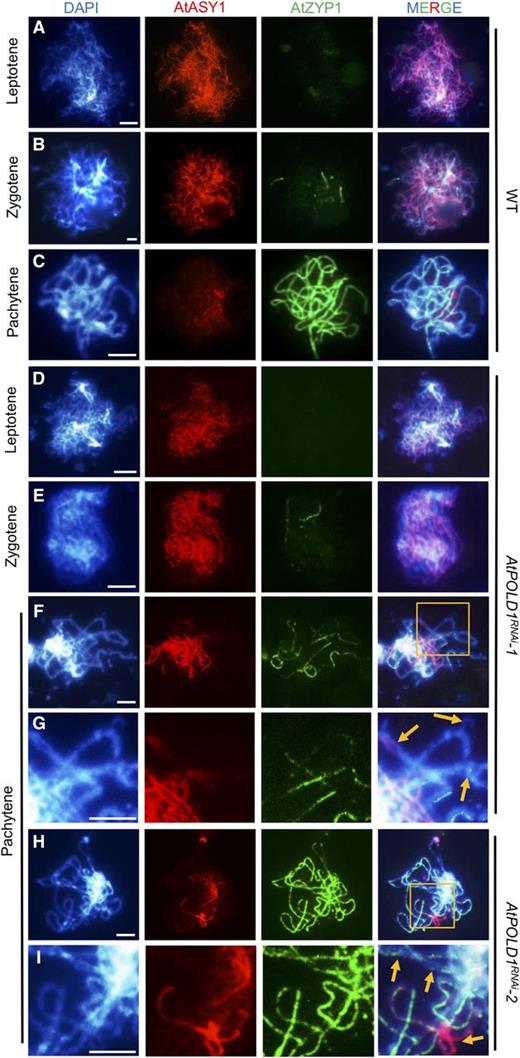
Immunolocalization of AtASY1 and AtZYP1 in the wild type and AtPOLD1RNAi. The distribution of AtASY1 and AtZYP1 in the wild type and AtPOLD1RNAi is shown at leptotene (A and D), zygotene (B and E), and pachytene (C and F–I). G and I show enlarged regions from F and H, respectively. DAPI staining is shown in blue, AtASY1 in red, and AtZYP1 in green. Yellow arrows indicate bubbles and bifurcations with aberrant AtZYP1. Bars = 5 μm. WT, wild type.
AtPOLD1 Is Dispensable for DSB Formation But Required for Its Repair
AtPOLD1RNAi meiocytes had a SPO11-1-dependent chromosome fragmentation phenotype that is indicative of a defect in meiotic DSB repair. To examine meiotic DSBs in AtPOLD1RNAi, we used immunostaining of two DSB markers, γ-H2AX, a phosphorylated histone H2AX (Lowndes and Toh, 2005), and RAD51, a recombinase that binds specifically to DSB ends to facilitate single end invasion (Li et al., 2004), in the wild type and AtPOLD1RNAi. Wild-type meiocytes had 178 ± 19 (n = 20) γ-H2AX foci at early zygotene, consistent with prior observations (Wang and Copenhaver, 2018), while AtPOLD1RNAi had 180 ± 17 foci (n = 20). Similarly, we did not observe any obvious difference in the number of AtRAD51 foci between AtPOLD1RNAi (173 ± 19, n = 19) and the wild type (171 ± 19, n = 19; Fig. 5, A, B, and E). Together, these results indicate that reducing meiosis-specific POLD1 expression does not influence DSB formation.
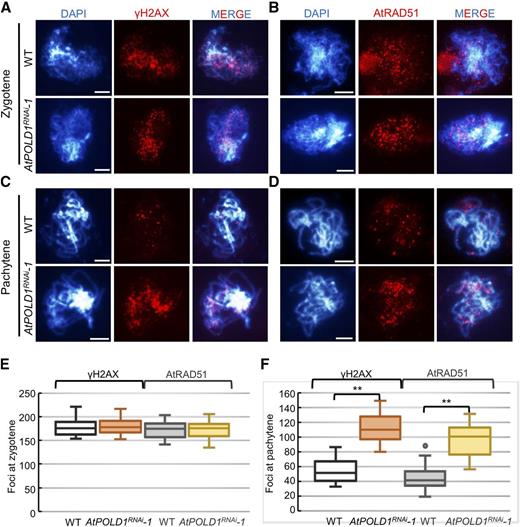
Immunolocalization of γ-H2AX and AtRAD51 in the wild type and AtPOLD1RNAi. A to D, Immunostaining of γ-H2AX and AtRAD51 at zygotene (A and B) and pachytene (C and D) in the wild type and AtPOLD1RNAi. Bars = 5 μm. E and F, Comparison of γ-H2AX and AtRAD51 foci counts in the wild type and AtPOLD1RNAi. **, P < 0.01, two-tailed Student’s t test. WT, wild type.
In contrast, we observed a significant difference in the number of γ-H2AX and AtRAD51 foci when comparing wild-type and AtPOLD1RNAi pachytene meiocytes. Typically, γ-H2AX and AtRAD51 foci decrease in number following DSB repair. In wild-type pachytene meiocytes, the number of γ-H2AX and AtRAD51 foci decreased to 54 ± 17 (n = 20) and 45 ± 19 (n = 20), respectively. However, AtPOLD1RNAi pachytene meiocytes had significantly more γ-H2AX (111 ± 21, n = 20) and AtRAD51 (95 ± 23, n = 20) foci (two-tailed Student’s t test, P < 0.01; Fig. 5, C, D, and F), suggesting compromised or delayed DSB repair. To confirm this observation, we used immunostaining of the meiosis-specific recombinase DMC1 (Bishop et al., 1992; Kurzbauer et al., 2012) in wild-type and AtPOLD1RNAi meiocytes. We found that AtDMC1 foci were elevated in AtPOLD1RNAi pachytene meiocytes compared with the wild type (Supplemental Fig. S6). The persistence of AtDMC1 foci in AtPOLD1RNAi suggests that the defect in DSB repair occurs after strand invasion, which is consistent with a role for AtPOLD1 in meiotic recombination-associated DNA synthesis.
AtPOLD1 Participates in Interference-Sensitive CO Formation
Previous studies have demonstrated that Arabidopsis RFC1 and POL2A are required for meiotic CO formation, specifically in the type I CO pathway (Huang et al., 2016). The reduced number of bivalents in AtPOLD1RNAi suggests a role for AtPOLD1 in CO formation as well. To test this hypothesis, we counted the number of chiasmata in the wild type (n = 31), AtPOLD1RNAi-1 (n = 30), and AtPOLD1RNAi-2 (n = 30) at early diakinesis. As described above, the chromosomes in AtPOLD1RNAi lines can form multivalents at diakinesis (Fig. 3B), which can hinder accurate chiasmata counting. To avoid ambiguity, we focused on AtPOLD1RNAi meiocytes at diakinesis with bivalents that allow more accurate chiasmata counting (Fig. 6A). The average number of chiasmata was 10.35 (n = 31) in the wild type, but a reduction in AtPOLD1RNAi-1 (9.13 per cell, n = 30; P < 0.01, Student’s t test; Fig. 6B) and in AtPOLD1RNAi-2 (9.17 per cell, n = 30; P < 0.01, Student’s t test; Fig. 6B) was apparent, supporting the conclusion that COs are reduced in AtPOLD1RNAi.
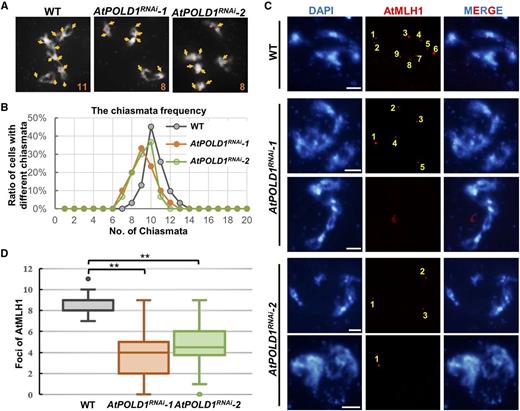
Estimation of meiotic recombination frequency in the wild type and AtPOLD1RNAi. A, Chiasmata (yellow arrows) on DAPI-stained chromosomes (white) at diakinesis in the wild type and AtPOLD1RNAi. B, Chiasmata counts per nucleus in the wild type and AtPOLD1RNAi. C, Immunostaining of AtMLH1 foci (red) on DAPI-stained chromosomes (blue) at diakinesis in the wild type and AtPOLD1RNAi. Yellow numbers indicate the number of AtMLH1 foci. Bars = 5 μm. D, Comparison of AtMLH1 foci counts in the wild type and AtPOLD1RNAi. **, P < 0.01, two-tailed Student’s t test. WT, wild type.
There are two CO pathways in Arabidopsis: the ZMM/type I/CO interference-sensitive pathway and the type II/CO interference-insensitive pathway that relies on various nuclease activities, like MUS81 (Copenhaver et al., 2002; Higgins et al., 2004; Berchowitz et al., 2007). We used immunostaining of AtMLH1, a marker of type I COs (Jackson et al., 2006), to determine whether the type I CO pathway is compromised in AtPOLD1RNAi. Compared with the wild type with an average of 8.92 MLH1 foci (n = 36), AtPOLD1RNAi-1 and AtPOLD1RNAi-2 had averages of 3.78 (two-tailed Student’s t test, P < 0.01; n = 37) and 4.73 (two-tailed Student’s t test, P < 0.01; n = 40), respectively (Fig. 6, C and D). The number of MLH1 foci ranged from zero to nine in AtPOLD1RNAi (Fig. 6D). These results indicate that AtPOLD1 is required for wild-type levels of type I CO formation.
AtPOLD1 and AtPOL2A May Have Divergent Roles during Meiotic Recombination
Our previous study on AtPOL2A hypothesized that AtPOLD1 and AtPOL2A may have different roles in meiotic recombination-associated DNA synthesis and that AtPOLD1 may be able to replace AtPOL2A in certain CO intermediates (Huang et al., 2015). To test this hypothesis, we transformed the pDMC1::AtPOLD1RNAi construct into the pol2a-1 − /+ plants (Huang et al., 2015) and obtained 71 transgenic T1 progeny, 11 of which were pol2a-1 −/−. AtPOLD1RNAi pol2a-1 plants had a stronger fertility defect than pol2a-1 single mutants and were almost completely sterile (Fig. 7A). We performed RT-qPCR to analyze the expression of AtPOLD1 in stage 4 to 7 anthers of wild-type, pol2a-1, AtPOLD1RNAi-1, AtPOLD1RNAi-2, and pol2a-1 AtPOLD1RNAi T2 plants and found significant reductions of AtPOLD1 expression (P < 0.01, two-tailed Student’s t test) in pol2a-1, AtPOLD1RNAi-1, AtPOLD1RNAi-2, and pol2a-1 AtPOLD1RNAi compared with the wild type (Supplemental Fig. S3C), indicating that POL2A may have a role in promoting AtPOLD1 expression. We also examined the expression of AtPOL2A in AtPOLD1RNAi and found a slight decrease (Supplemental Fig. S3D), indicating that they may have a reciprocal influence on gene expression, possibly indirectly.
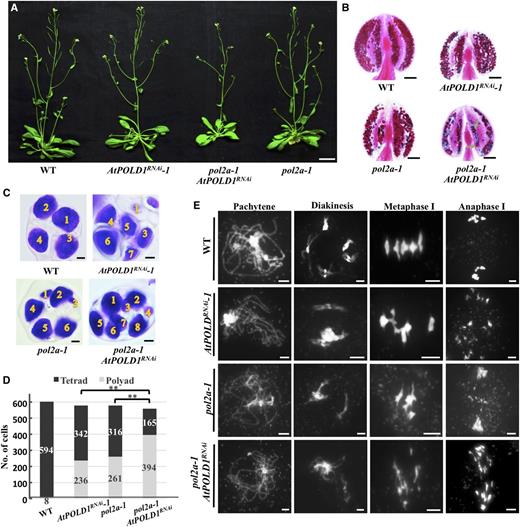
atpol2a-1 AtPOLD1RNAi has severe meiotic defects. A, Vegetative phenotypes of wild-type and mutant plants. B, Alexander Red staining of wild-type and mutant anthers. Green arrows indicate large-size pollen grains compared with the wild type. C, Tetrad-stage developing pollen from the wild type and mutants stained with Toluidine Blue. D, Histogram of tetrads and polyads in the wild type and mutants. **, P < 0.01, χ2 test. E, DAPI-stained chromosomes of the wild type and mutants. Bars = 3 cm (A), 0.1 mm (B), 5 μm (C), and 5 μm (E). WT, wild type.
Alexander Red staining showed that pol2a-1 AtPOLD1RNAi anthers contained fewer viable pollen grains (101 ± 31 per anther, n = 17) than AtPOLD1RNAi-1 (249 ± 42 per anther, n = 16) or pol2a-1 (212 ± 65 per anther, n = 16; Fig. 7B). Unlike the wild type, which had uniformly sized pollen grains, pol2a-1 AtPOLD1RNAi pollen grains varied in size, with some larger than those of the wild type (Fig. 7B). Consistent with this observation, wild-type plants had four equally sized microspores at the tetrad stage, while pol2a-1 AtPOLD1RNAi had polyads with various numbers of microspores (Fig. 7C). Moreover, the proportion of polyads in pol2a-1 AtPOLD1RNAi (70%, n = 559) was higher than that in AtPOLD1RNAi (40%, n = 578; P < 0.01) or pol2a-1 (45%, n = 577; P < 0.01), supporting the conclusion that the meiotic defect in the double mutants was more severe than that in the single mutants. We used chromosome spreads to examine chromosome morphology but observed no obvious difference in nonhomolog interactions at diakinesis or fragmentation after metaphase I between pol2a-1 AtPOLD1RNAi and the single mutants (Fig. 7E). However, chromosome fragmentation appeared more severe in the double mutants at anaphase I (Fig. 7E). Together, these observations imply that AtPOLD1 and AtPOL2A may have different functions during meiotic recombination.
DISCUSSION
POLD1 Is Required for Homologous Recombination during Both Mitosis and Meiosis
Semiconservative DNA replication is a conserved process and is orchestrated primarily by three multisubunit DNA polymerases that coordinate with other replication factors, such as the minichromosome maintenance complex, CDC45, RFC1, and PCNA (Waga and Stillman, 1998). The three major DNA polymerases are POL α, POL δ, and POL ε, in which Pol α is responsible for the initiation of DNA replication on both the leading and lagging strands and for the synthesis of Okazaki fragments during lagging strand synthesis, while POL δ and POL ε are required for the elongation of the lagging and leading strands, respectively. Null alleles of genes for these polymerases usually lead to embryonic lethality in eukaryotes. However, some viable weak mutant alleles have been identified, allowing the analyses of their roles in replication and other processes (Maloisel et al., 2004, 2008; Liu et al., 2010; Huang et al., 2015; Iglesias et al., 2015). For example, AtPOL δ functions in several DNA repair pathways, in the maintenance of genome stability, as well as in the epigenetic regulation of flowering (Branzei and Foiani, 2010; Prindle and Loeb, 2012; Iglesias et al., 2015). In yeast, pol3-ct (a mutant allele of POL δ with a deletion of the last four amino acids) has decreased CO number and shorter meiotic gene conversion tracts (Maloisel et al., 2004). In addition to pol3-ct, several other Pol3 mutants reveal a role in the repair of somatic DSBs and homologous recombination (HR; Giot et al., 1997; Pâques and Haber, 1997; Lydeard et al., 2007; Maloisel et al., 2008; Schuermann et al., 2009; Prindle and Loeb, 2012). During yeast mitotic DNA repair, POL δ, but not POL ε, is recruited to the 3′ end of the invading strand within the D loop for the formation of longer HR intermediates (Maloisel et al., 2008; Wilson et al., 2013). In mammals, mutants with lesions in the exonuclease domain lacking the proofreading activity in POL δ exhibit apparently normal development but have a higher incidence of cancer (Prindle and Loeb, 2012; Palles et al., 2013), suggesting defects in DNA repair. In Arabidopsis, viable mutants of pol α (POLA1) and pol ε (POLE1/POL2A) and a thermosensitive mutant (gis5) show defects in development and stress responses, consistent with abnormal mitotic HR (Schuermann et al., 2009; Yin et al., 2009; Liu et al., 2010; Iglesias et al., 2015). These results demonstrated that the DNA replication machinery, including POL δ, is important for genome stability, stalled replication forks, and somatic HR.
Meiotic recombination also requires the repair of programmed DSBs (Pastink et al., 2001; Marcon and Moens, 2005). Consistently, previous studies have shown that the DNA synthesis proteins AtPOL α, AtPOL ε, and AtRFC1 are required for meiotic recombination (Wang et al., 2012; Liu et al., 2013; Huang et al., 2015, 2016). Here, we showed that meiocyte-specific reduction of AtPOLD1 expression does not affect normal vegetative development in Arabidopsis but does cause reduced fertility (Fig. 1) and meiotic defects, including nonhomologous chromosome interactions, the formation of multivalents, chromosome fragmentation, and unequal chromosome segregation (Figs. 2 and 3). Moreover, homologs of AtPOLD1RNAi meiocytes were incompletely synapsed during prophase I (Fig. 4) and had normal numbers of DSBs, but the repair of these DSBs was delayed (Fig. 5; Supplemental Fig. S5). We also provided evidence that AtPOLD1 participates in type I CO formation and displays functional redundancy to AtPOL ε (Figs. 6 and 7). Together, these data provided direct evidence that AtPOL δ is required for normal meiosis and meiotic recombination.
Genes Required for DNA Lagging Strand Synthesis Are Essential for Meiotic Recombination
We found that chromosomes formed multivalents (Figs. 2 and 3) in AtPOLD1RNAi meiocytes, indicating that AtPOLD1 ensures the normal formation of bivalents between homologs. In the meiotic DSBR model, the recombinases RAD51 and DMC1 facilitate the invasion of the 3′ ssDNA end of the DSB into a nonsister chromatid to form a D-loop intermediate. The completion of DSB repair is accompanied by a dramatic reduction in RAD51 and DMC1 foci visualized by immunostaining. In AtPOLD1RNAi, greater than normal numbers of AtRAD51 and AtDMC1 foci were present at pachytene (Fig. 5; Supplemental Fig. S5), similar to the phenotype observed previously in atrfc1-2 mutants (Wang et al., 2012), suggesting that AtPOLD1 also is required for the dissociation of RAD51 and DMC1 from the 3′ ends and subsequent DSB repair. In yeast, POL δ functions during the removal of nonhomologous DNA ends and the invasion of the ssDNA 3′ tails during mitotic DSBR (Pâques and Haber, 1997), indicating that POL δ plays a role in single end invasion or D-loop formation. Based on these findings, we hypothesize that the lagging strand synthesis factors, like POL δ and RFC complex, are important for heteroduplex formation and that insufficient POL δ function might result in the disordered invasion of ssDNA 3′ ends during meiosis.
During Arabidopsis meiosis, recombination-defective mutants, such as atspo11-1, atrad51, and atdmc1, show clear defects in synapsis, which indicates that recombination has a role in promoting synapsis. The defects in the distribution of AtRAD51 and AtDMC1 in AtPOLD1RNAi also were accompanied by aberrant synapsis, as supported by the formation of abnormal bubbles and bifurcations at pachytene (Figs. 2 and 3). Additional evidence supporting a defect in synapsis in AtPOLD1RNAi includes the prolonged presence of AtASY1 along chromosomes and the discontinuous localization of AtZYP1 (Fig. 4). The associations of nonhomologous chromosomes in AtPOLD1RNAi also could be regarded as a complement to inefficient SC formation.
AtPOLD1RNAi plants had considerably fewer bivalents and significantly fewer type I COs compared with the wild type. Similarly, AtRFC1 also is required for type I CO formation (Wang et al., 2012). Since the modest reduction of bivalents might not mirror the more severe reduction of type I COs, as measured by MLH1 immunostaining, the closer to normal number of total COs in atrfc1-2 and AtPOLD1RNAi may be due to increases in type II COs. Alternatively, there might be an increase in COs generated by the recently described FANCD2-dependent pathway (Kurzbauer et al., 2018). Consequently, since meiotic recombination-associated DNA synthesis appears to function after single end invasion and prior to the formation of the double Holliday junctions, we propose that lagging strand synthesis factors are important for promoting the formation of type I COs.
A Potential Role of POL δ in Meiotic Recombination
Our previous study on AtPOL2A (Pol ε) supported the hypothesis that the meiotic type I CO pathway likely involves both short and long CO-associated conversion tracts (Huang et al., 2015). Here, we further hypothesized that AtPOLD1 likely coordinates with POL2A in meiotic recombination. We provided several lines of evidence to support this new hypothesis for Pol δ. First, meiosis-specific knockdown of AtPOLD1 in Arabidopsis resulted in reduced fertility and AtSPO11-1-dependent meiotic defects. Second, the AtPOLD1RNAi meiotic defects included the formation of multivalents, chromosome fragments, incomplete synapsis, and reduced type I COs, resembling previously characterized rfc1 and pol2a mutants, with more similarity to rfc1 than to pol2a. Third, the pol2a-1 AtPOLD1RNAi double mutant had more severe meiotic defects than either single mutant (Fig. 7).
Besides, studies in yeast found that POL δ, but not POL ε, promotes heteroduplex DNA extension during the repair of somatic DSBs (Maloisel et al., 2008) and that the recruitment of POL δ to the invading strand in the extension of D-loops is assisted by the PCNA-RFC1 complex (Li et al., 2009) and the DNA helicase Pif1 (Wilson et al., 2013; Buzovetsky et al., 2017). These results further strengthen the idea that POL δ is important for recombination.
Based on our results here and prior observations, we hypothesize that AtPOLD1 might function in the step of DNA synthesis during meiotic interference-sensitive recombination. During mitotic DNA replication, POL α-primase, RFC1, PCNA, and POL δ have been reported to participate in the initiation of DNA synthesis and the extension of D-loops (Lydeard et al., 2007; Li et al., 2009; Wilson et al., 2013). We propose that, during meiotic DSBR, following single end invasion, lagging strand factors might stabilize the resulting intermediate by facilitating DNA synthesis and heteroduplex DNA formation. This idea is supported by a recent study in budding yeast showing that POL δ contributes prominently to both leading and lagging DNA synthesis, while POL ε functions mainly in proofreading or is an alternative candidate for leading strand synthesis (Johnson et al., 2015). More recently, another study further verified that POL δ contributes to the initiation of both strand replication but is less efficient for leading-strand elongation (Garbacz et al., 2018). These data also imply that, due to the different lengths of recombination tracts, POL δ and POL ε also might function coordinately in meiotic recombination-associated DNA synthesis. Consequently, the stabilization and extension of the D-loop by lagging strand synthesis factors promote the formation of recombination intermediates, consistent with the biochemical data observed in yeast (Maloisel et al., 2004, 2008; Lydeard et al., 2007; Li et al., 2009; Buzovetsky et al., 2017), which can be resolved as type I COs. In contrast, in the absence of POL δ (POLD1), POL ε alone cannot promote type I formation, as the observed COs in pol2a are mostly type II dependent (Huang et al., 2015). Alternatively, the failure of type I CO formation might facilitate the 3′ end to search for a nonhomolog to form multivalents. Due to the conserved nature of meiotic recombination during sexual reproduction and the conservation of DNA polymerases in eukaryotes, we suggest that lagging strand synthesis factors play a role in the preferential formation of interference-sensitive COs by promoting more extensive DNA synthesis, and this is likely conserved in other organisms.
MATERIALS AND METHODS
Plant Materials and Growth Conditions
Wild-type Arabidopsis (Arabidopsis thaliana) Columbia, pol2a-1 (SALK_096341), spo11-1-1 (Grelon et al., 2001), and transgenic plants were grown in soil in an environmentally controlled greenhouse at 22°C under long-day conditions (16 h of light/8 h of dark).
Plasmid Construction and Plant Transformation
To generate transgenic AtPOLD1RNAi plants, a 315-bp region of the POL domain of POLD1 was amplified using PCR with the primers Omf10670 and Omf10671 (Supplemental Table S4), which contain SpeI/XbaI and ApaI/SalI restriction sites, respectively. The amplification product was ligated into the pMeioDMC1-intron vector using SpeI/ApaI for the sense fragment and SalI/XbaI for the antisense fragment. The pDMC1::AtPOLD1RNAi construct was introduced into Agrobacterium tumefaciens GV3101 for the transformation of Columbia and pol2a-1 heterozygous mutants (Clough and Bent, 1998). T1 seeds were germinated and grown on selective media, including 0.5× Murashige and Skoog medium with 25 mg L−1 hygromycin, for 14 d. The positive transgenic seedling plants then were transferred to soil and grown in a greenhouse under 22°C with 16 h of light/8 h of dark, and T2 seeds were harvested.
Morphological Analysis of Plants
Whole plants were photographed using a Canon SX20 IS digital camera. Images of dissected seed pods were taken using a Zeiss Stereo Discovery stereomicroscope. Pollen viability was assessed via Alexander Red staining (Alexander, 1969) at 65°C for 45 min. The analysis of tetrad-stage developing pollen was performed as described previously (Wang et al., 2014). Images of tetrad-stage and mature pollen were collected using a Zeiss Axio Scope A1 microscope.
Cytological Analysis
Chromosome spreads, fluorescence in situ hybridization, and immunofluorescence all were performed following the procedures described previously (Wang et al., 2014). Rabbit-sourced polyclonal antibodies raised against RAD51, ASY1, and MLH1 and rat-sourced ZYP1 antibodies were described previously (Huang et al., 2015). They were used at a 1:200 dilution in blocking buffer. The rabbit-sourced polyclonal DMC1 antibody was newly generated (Shanghai Ango Biotechnology) and used at a 1:500 dilution. The secondary antibodies Alexa Fluor 488 Goat Anti-Rat IgG (H+L; A-21208) and Alexa Fluor 555 Goat Anti-Rabbit IgG (H+L; A-21428; Invitrogen) were used at 1:500 and 1:1,000 dilutions, respectively. Chiasmata counting was performed as described previously (Sanchez Moran et al., 2001). All cytological images were photographed using a Zeiss Axio Scope A1 microscope.
Expression Analysis
Total RNA was extracted from stage 4 to 7 anthers using Trizol reagent (Invitrogen) following the manufacturer’s instructions. Reverse transcription was performed using PrimeScript RT with gDNA Eraser (Takara). Quantitative PCR was performed using iTaq Universal SYBR Green supermix (Bio-Rad) employing the ƊCt method (Livak and Schmittgen, 2001). Samples were normalized to AtTIP41 expression as reported previously (Czechowski et al., 2005). RT-qPCR primers are listed in Supplemental Table S4.
Accession Numbers
The Arabidopsis Genome Initiative locus identifiers for the genes analyzed in this study are as follows: POLD1 (At5g63960), POL2A (AT1G08260), SPO11-1 (AT3G13170), RFC1 (At5g22010), ASY1 (AT1G67370), ZYP1 (AT1G22260), RAD51 (At5g20850), DMC1 (AT3G22880), and MLH1 (AT4G09140).
Supplemental Data
The following supplemental materials are available.
Supplemental Figure S1. Phylogenetic analysis of POLD1 homologs.
Supplemental Figure S2. Schematic representation of AtPOLD1 and the structure of pDMC1::AtPOLD1RNAi.
Supplemental Figure S3. Transcriptional analyses of AtPOLD1 and AtPOL2A.
Supplemental Figure S4. Siliques and seeds of the reciprocal cross lines between the wild type and AtPOLD1RNAi.
Supplemental Figure S5. Chromosome morphologies of female meiosis in the wild type and AtPOLD1RNAi-1.
Supplemental Figure S6. Immunolocalization of AtDMC1 in the wild type and AtPOLD1RNAi-1.
Supplemental Table S1. Expression levels of DNA polymerase genes in male meiocytes and seedlings.
Supplemental Table S2. The reciprocal cross between AtPOLD1RNAi and the wild type shows the female fertility of AtPOLD1RNAi.
Supplemental Table S3. Bivalent frequency at diakinesis in AtPOLD1RNAi.
Supplemental Table S4. Primers used in this study.
ACKNOWLEDGMENTS
We thank Raphaël Mercier at the Institut National de la Recherche Agronomique for providing the MLH1 antibody.
LITERATURE CITED
Author notes
This work was supported by the National Science Foundation of China (31570314, 31870293, and 31600246) and funds from the State Key Laboratory of Genetic Engineering, Fudan University, and Rijk Zwaan.
These authors contributed equally to the article.
Author for contact: [email protected].
Senior Author.
The author responsible for distribution of materials integral to the findings presented in this article in accordance with the policy described in the Instructions for Authors (www.plantphysiol.org) is: Yingxiang Wang ([email protected]).
C.W., J.H., and Y.W. conceived the research; C.W., J.H., J.Z., H.W., and Y.H. performed the experiments; H.M. and Y.W. supervised the experiments and contributed to funding; C.W., J.H., and Y.W. analyzed the data and wrote the article; G.P.C. and H.M. contributed to article writing and editing.
Articles can be viewed without a subscription.