-
PDF
- Split View
-
Views
-
Cite
Cite
Qiang-Nan Feng, Xin Liang, Sha Li, Yan Zhang, The ADAPTOR PROTEIN-3 Complex Mediates Pollen Tube Growth by Coordinating Vacuolar Targeting and Organization, Plant Physiology, Volume 177, Issue 1, May 2018, Pages 216–225, https://doi.org/10.1104/pp.17.01722
- Share Icon Share
Abstract
Pollen tube growth is an essential step for successful plant reproduction. Vacuolar trafficking and dynamic organization are important for pollen tube growth; however, the key proteins involved in these processes are not well understood. Here, we report that the ADAPTOR PROTEIN-3 (AP-3) complex and its tonoplast cargo PROTEIN S-ACYL TRANSFERASE10 (PAT10) are critical for pollen tube growth in Arabidopsis (Arabidopsis thaliana). AP-3 is a heterotetrameric protein complex consisting of four subunits, δ, β, µ, and σ. AP-3 regulates tonoplast targeting of several cargoes, such as PAT10. We show that functional loss of any of the four AP-3 subunits reduces plant fertility. In ap-3 mutants, pollen development was normal but pollen tube growth was compromised, leading to reduced male transmission. Functional loss of PAT10 caused a similar reduction in pollen tube growth, suggesting that the tonoplast association of PAT10 mediated by AP-3 is crucial for this process. Indeed, the Ca2+ gradient during pollen tube growth was reduced significantly due to AP-3 loss of function, consistent with the abnormal targeting of CALCINUERIN B-LIKE2 (CBL2) and CBL3, whose tonoplast association depends on PAT10. Furthermore, we show that the pollen tubes of ap-3 mutants have vacuoles with simplified tubules and bulbous structures, indicating that AP-3 affects vacuolar organization. Our results demonstrate a role for AP-3 in plant reproduction and provide insights into the role of vacuoles in polarized cell growth.
The sexual reproduction of flowering plants is a complex, multistep process involving gametophytic development, interactions between male and female gametophytes, and interactions between male and female gametes (McCormick, 2004). Due to the immobility of sperm cells in angiosperms, the delivery of sperm into embryo sacs depends on pollen tubes, long cylindrical extensions initiated from the growing tip of pollen grains. Pollen tubes penetrate female sporophytic tissues, target the micropyle by sensing and responding to female guidance cues, and finally burst to release sperm cells into embryo sacs (Johnson and Preuss, 2002). Tip growth of pollen tubes is a specialized polar growth that involves complex intracellular activities, including a tip-focused Ca2+ gradient, spatial organization of endomembrane systems, and dynamic organization of the cytoskeleton (Hepler et al., 2001, 2012; Cheung and Wu, 2007, 2008; Cheung et al., 2008).
Pollen tube growth accompanies dynamic vacuolar organization and trafficking (Hicks et al., 2004). Vacuoles, as extensive tubular extensions, fill with growing pollen tubes, except at the very apex (Hicks et al., 2004; Wudick et al., 2014). Functional loss of VACUOLESS1 (VCL1), a gene essential for vacuolar biogenesis (Rojo et al., 2001), resulted in reduced male gametophytic transmission, although the vacuoles in vcl1 pollen tubes seemed normal (Hicks et al., 2004). Overexpression of PTEN, a phosphatase that down-regulates PtdIns3P, the phosphoinositide critical for vacuolar fusion, resulted in defective pollen tube growth by disrupting the vacuolar consumption of autophagic bodies in Arabidopsis (Arabidopsis thaliana; Zhang et al., 2011). In addition, mutations at VPS41, encoding a component of the homotypic fusion and vacuolar protein sorting complex that mediates vesicle-vacuole fusion, caused defective pollen tube growth in response to female cues (Hao et al., 2016). These results suggested that vacuolar trafficking and organization are critical for the polar and directional growth of pollen tubes. However, the key proteins targeted to vacuoles and critical for pollen tube growth are yet unknown.
The proper targeting of proteins within the endomembrane system is crucial for cell growth and responses to the environment. Adaptor proteins (APs) are key players mediating protein sorting; they recognize both cargo proteins and coat proteins during vesicle formation (Boehm and Bonifacino, 2001; Robinson, 2004; Bassham et al., 2008). APs are heterotetrameric protein complexes. There are five AP complexes in eukaryotes, differing in their subcellular targeting and functionalities: AP-1, AP-2, AP-3, AP-4, and AP-5 (Boehm and Bonifacino, 2001; Robinson, 2004; Bassham et al., 2008; Hirst et al., 2011). In plants, except for the newly discovered AP-5, the other types of APs have been characterized. AP-1 associates with the trans-Golgi network/early endosome to sort proteins to the plasma membrane, to the forming cell plate, or to vacuoles (Park et al., 2013; Teh et al., 2013). Functional loss of AP-1 reduced male and female fertility (Park et al., 2013; Teh et al., 2013; Wang et al., 2013). AP-2 participates in clathrin-mediated endocytosis and is required for reproductive organ development (Fan et al., 2013; Kim et al., 2013; Yamaoka et al., 2013). Another class of ancient adaptor complex termed TPLATE/TSET (Gadeyne et al., 2014; Hirst et al., 2014) was reported to mediate reproduction, as mutations in those components caused pollen developmental defects (Van Damme et al., 2006; Gadeyne et al., 2014). AP-4 was reported recently to mediate vacuolar trafficking (Fuji et al., 2016). Several studies indicated that AP-3 mediates the vacuolar targeting of several protein cargoes, including SUCROSE TRANSPORTER4 (SUC4; Wolfenstetter et al., 2012), the R-SNARE subfamily members VAMP713 (Ebine et al., 2014) and VAMP711 (Feng et al., 2017a), as well as PROTEIN S-ACYL TRANSFERASE10 (PAT10; Feng et al., 2017a). The functional loss of AP-3 resulted in reduced germination potential of seeds (Feraru et al., 2010; Zwiewka et al., 2011) and slight defects in gravitropic responses (Feraru et al., 2010). It is unclear whether AP-3 affects reproductive processes.
In this study, we analyze mutants of all four AP-3 subunits and show that AP-3 and its tonoplast cargo PAT10 are important for pollen tube growth. Functional loss of single AP-3 subunits reduced male transmission and caused reduced seed set. Pollen development was not affected by AP-3 loss-of-function. However, the rapid growth of pollen tubes both in vitro and in vivo was compromised in the ap-3 mutants. Functional loss of PAT10 caused a similar reduction in pollen tube growth, suggesting that the AP-3-mediated vacuolar targeting of PAT10 is crucial for this process. We further show that AP-3 loss-of-function affected the vacuolar organization of pollen tubes, as vacuoles of mutant pollen tubes were less complex tubules, with attached bulbous structures. Our results demonstrate a role for AP-3 in plant reproduction and provide insights into the role of vacuoles in polarized cell growth.
RESULTS
AP-3 Loss-of-Function Results in Reduced Seed Set
To determine the potential roles of AP-3 during plant reproduction, we characterized mutants of each AP-3 component (i.e. AP-3δ, AP-3β, AP-3µ, and AP-3σ; Fig. 1A). Each component is encoded by a single gene in Arabidopsis (Bassham et al., 2008). Mutants of AP-3δ, AP-3β, and AP-3µ used in this study were designated previously as protein affected trafficking4 (pat4-2), pat2-2, and ap-3µ-2, respectively, and were proven to be null mutants (Niihama et al., 2009; Feraru et al., 2010; Zwiewka et al., 2011). Only one T-DNA insertion mutant was available within the genomic region of AP-3σ, in which the full-length transcript of AP-3σ was below RT-qPCR detection (Feng et al., 2017a). To be consistent with their functional roles as AP-3 components, and for simplicity, we refer to these mutants as ap-3δ, ap-3β, ap-3µ, and ap-3σ.
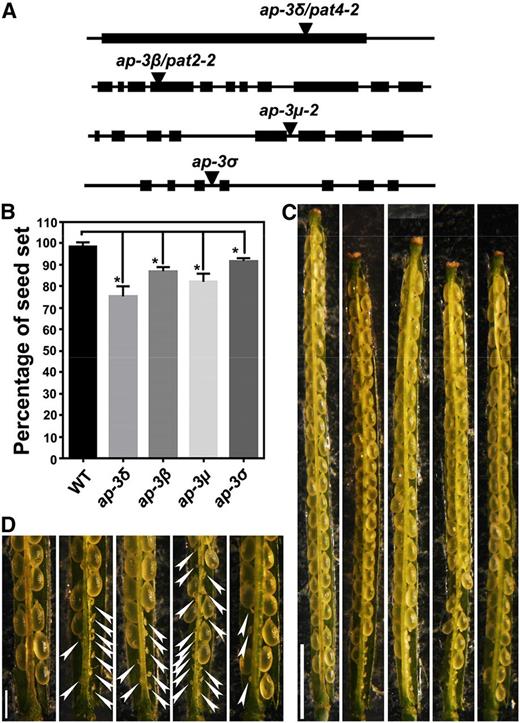
Mutations at AP‐3 subunits result in reduced seed set. A, Schematic illustration of T-DNA insertions within the genomic regions of AP-3 subunit-encoding genes, AP-3δ, AP-3β, AP-3µ, and AP-3σ. Arrowheads point at the T-DNA insertion sites. B, Quantitative analysis of seed set in the wild type (WT) and the ap-3 mutants. Results are means ± sd (n = 20). Each mutant is significantly different from the wild type in seed set, as indicated by asterisks (one-way ANOVA, Dunnett’s multiple comparison test, P < 0.05). C and D, From left to right: representative siliques from the wild type, ap-3δ, ap-3β, ap-3µ, and ap-3σ. Closeup images of the bottom part of C are shown in D. Arrowheads point at undeveloped ovules. Bars = 2 mm for C and 500 µm for D.
Each of the mutants was similar to the wild type during the vegetative stage, as reported (Feraru et al., 2010; Zwiewka et al., 2011). However, during the reproductive stage, each mutant showed significantly reduced seed set (Fig. 1B), with undeveloped ovules distributed predominantly at the bottom of the siliques (Fig. 1, C and D). These results showed that AP-3 is important for reproduction.
Functional Loss of AP-3 Reduces Male Transmission But Does Not Affect Pollen Development
To determine the reason for the reduced seed set, we performed reciprocal crosses between the wild type and each heterozygous mutant: ap-3δ, ap-3β, ap-3µ, or ap-3σ. Segregation ratios of the F1 progeny demonstrated that each mutant showed reduced male but not female transmission (Table I). These results demonstrated that AP-3 is involved in male gametophytic function.
AP-3 loss-of-function resulted in defective male transmission
Parents (Female × Male) . | F1 Progeny . | ||
---|---|---|---|
Genotypea . | Expected Ratio . | Observed Ratio . | |
ap-3δ +/− × wild type | ap-3δ +/+:+/− | 1:1 | 74:68 |
Wild type × ap-3δ +/− | ap-3δ +/+:+/− | 1:1 | 87:7b |
ap-3δ +/− × ap-3δ +/− | ap-3δ +/+:+/−:−/− | 1:2:1 | 13:20:2c |
ap-3β +/− × wild type | R:S | 1:1 | 94:87 |
Wild type × ap-3β +/− | R:S | 1:1 | 11:159b |
ap-3β +/− × ap-3β +/− | R:S | 3:1 | 369:211d |
ap-3μ +/− × wild type | ap-3μ +/+:+/− | 1:1 | 99:107 |
Wild type × ap-3μ +/− | ap-3μ +/+:+/− | 1:1 | 170:8b |
ap-3μ +/− × ap-3μ +/− | ap-3μ +/+:+/−:−/− | 1:2:1 | 42:38:16c |
ap-3σ +/− × wild type | R:S | 1:1 | 125:110 |
Wild type × ap-3σ +/− | R:S | 1:1 | 39:56b |
ap-3σ +/− × ap-3σ +/− | R:S | 3:1 | 409:150d |
Parents (Female × Male) . | F1 Progeny . | ||
---|---|---|---|
Genotypea . | Expected Ratio . | Observed Ratio . | |
ap-3δ +/− × wild type | ap-3δ +/+:+/− | 1:1 | 74:68 |
Wild type × ap-3δ +/− | ap-3δ +/+:+/− | 1:1 | 87:7b |
ap-3δ +/− × ap-3δ +/− | ap-3δ +/+:+/−:−/− | 1:2:1 | 13:20:2c |
ap-3β +/− × wild type | R:S | 1:1 | 94:87 |
Wild type × ap-3β +/− | R:S | 1:1 | 11:159b |
ap-3β +/− × ap-3β +/− | R:S | 3:1 | 369:211d |
ap-3μ +/− × wild type | ap-3μ +/+:+/− | 1:1 | 99:107 |
Wild type × ap-3μ +/− | ap-3μ +/+:+/− | 1:1 | 170:8b |
ap-3μ +/− × ap-3μ +/− | ap-3μ +/+:+/−:−/− | 1:2:1 | 42:38:16c |
ap-3σ +/− × wild type | R:S | 1:1 | 125:110 |
Wild type × ap-3σ +/− | R:S | 1:1 | 39:56b |
ap-3σ +/− × ap-3σ +/− | R:S | 3:1 | 409:150d |
R for Basta resistant and S for Basta sensitive. Basta sensitivity was used to distinguish the mutant copy of ap-3β or ap-3σ, resistant to Basta salt, from its wild-type copy.
Significantly different from the segregation ratio 1:1 (χ2, P < 0.01).
Significantly different from the segregation ratio 1:2:1 (χ2, P < 0.01).
Significantly different from the segregation ratio 3:1 (χ2, P < 0.01).
Parents (Female × Male) . | F1 Progeny . | ||
---|---|---|---|
Genotypea . | Expected Ratio . | Observed Ratio . | |
ap-3δ +/− × wild type | ap-3δ +/+:+/− | 1:1 | 74:68 |
Wild type × ap-3δ +/− | ap-3δ +/+:+/− | 1:1 | 87:7b |
ap-3δ +/− × ap-3δ +/− | ap-3δ +/+:+/−:−/− | 1:2:1 | 13:20:2c |
ap-3β +/− × wild type | R:S | 1:1 | 94:87 |
Wild type × ap-3β +/− | R:S | 1:1 | 11:159b |
ap-3β +/− × ap-3β +/− | R:S | 3:1 | 369:211d |
ap-3μ +/− × wild type | ap-3μ +/+:+/− | 1:1 | 99:107 |
Wild type × ap-3μ +/− | ap-3μ +/+:+/− | 1:1 | 170:8b |
ap-3μ +/− × ap-3μ +/− | ap-3μ +/+:+/−:−/− | 1:2:1 | 42:38:16c |
ap-3σ +/− × wild type | R:S | 1:1 | 125:110 |
Wild type × ap-3σ +/− | R:S | 1:1 | 39:56b |
ap-3σ +/− × ap-3σ +/− | R:S | 3:1 | 409:150d |
Parents (Female × Male) . | F1 Progeny . | ||
---|---|---|---|
Genotypea . | Expected Ratio . | Observed Ratio . | |
ap-3δ +/− × wild type | ap-3δ +/+:+/− | 1:1 | 74:68 |
Wild type × ap-3δ +/− | ap-3δ +/+:+/− | 1:1 | 87:7b |
ap-3δ +/− × ap-3δ +/− | ap-3δ +/+:+/−:−/− | 1:2:1 | 13:20:2c |
ap-3β +/− × wild type | R:S | 1:1 | 94:87 |
Wild type × ap-3β +/− | R:S | 1:1 | 11:159b |
ap-3β +/− × ap-3β +/− | R:S | 3:1 | 369:211d |
ap-3μ +/− × wild type | ap-3μ +/+:+/− | 1:1 | 99:107 |
Wild type × ap-3μ +/− | ap-3μ +/+:+/− | 1:1 | 170:8b |
ap-3μ +/− × ap-3μ +/− | ap-3μ +/+:+/−:−/− | 1:2:1 | 42:38:16c |
ap-3σ +/− × wild type | R:S | 1:1 | 125:110 |
Wild type × ap-3σ +/− | R:S | 1:1 | 39:56b |
ap-3σ +/− × ap-3σ +/− | R:S | 3:1 | 409:150d |
R for Basta resistant and S for Basta sensitive. Basta sensitivity was used to distinguish the mutant copy of ap-3β or ap-3σ, resistant to Basta salt, from its wild-type copy.
Significantly different from the segregation ratio 1:1 (χ2, P < 0.01).
Significantly different from the segregation ratio 1:2:1 (χ2, P < 0.01).
Significantly different from the segregation ratio 3:1 (χ2, P < 0.01).
Several steps lead to proper male gametophytic function, specifically, pollen development, pollen germination, polar and directional growth of pollen tubes, and pollen tube reception (McCormick, 2004). To determine the causes of compromised male gametophytic function in the ap-3 mutants, we first analyzed pollen development using Alexander staining for cytoplasmic viability, 4′,6-diamino-phenylindole staining for nuclear structure, and scanning electron microscopy (SEM) for pollen coat structure (Johnson-Brousseau and McCormick, 2004). The ap-3 mutants were no different from the wild type (Supplemental Fig. S1), indicating that AP-3 is not involved in pollen development.
AP-3 Loss-of-Function Causes a Reduction in Pollen Tube Growth But Not in Guidance
To determine the cause of reduced male transmission in the ap-3 mutants, we next performed in vitro pollen germination (Fig. 2, A–E). All ap-3 mutants were comparable to the wild type in germination potential (Fig. 2F), indicating that AP-3 is not essential for pollen germination. In the first 2 h of pollen germination, there was no difference in pollen tube length between the ap-3 mutants and the wild type (Supplemental Fig. S2). However, at later stages (4 and 6 h), pollen tube growth in the ap-3 mutants was reduced significantly (Fig. 2, B–E) compared with the wild type (Fig. 2A) at the same time points (Fig. 2G; Supplemental Fig. S2). In addition, the pollen tube width of the ap-3 mutants was slightly but significantly increased (Fig. 2H), suggesting compromised tube polarity.
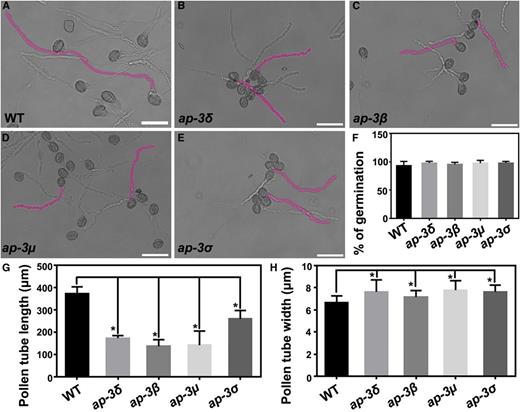
Functional loss of AP‐3 reduces pollen tube growth in vitro. A to E, In vitro pollen tubes from the wild type (WT; A), ap-3δ (B), ap-3β (C), ap-3µ (D), and ap-3σ (E) at 4 h after germination. Two representative pollen tubes from each genotype are highlighted in pink. Bars = 50 µm. F to H, Germination ratio (F), length (G), and apical width (H) of pollen tubes after 4 h of incubation in germination medium. Results shown are means ± sd (n = 300 for F, 100 for G, and 60 for H). All mutants are not significantly different from the wild type in the germination ratio (one-way ANOVA, Dunnett’s multiple comparison test, P > 0.05). Each mutant is significantly different from the wild type in the length or width of pollen tubes, as indicated by asterisks (one-way ANOVA, Dunnett’s multiple comparison test, P < 0.05).
To test whether pollen tube growth in the ap-3 mutants also was affected in vivo, we emasculated and hand pollinated wild-type pistils with pollen from the ap-3 mutants or the wild type. Using Aniline Blue staining of pistils at 9 h after pollination (HAP), we determined that wild-type pollen tubes could grow to the bottom of the pistils (Fig. 3A), whereas pollen tubes of the ap-3 mutants could not (Fig. 3, B–E). Among the ap-3 mutants, ap-3σ showed the least severe phenotypic defect in pollen tube growth in vivo (Fig. 3E), consistent with its seed set reduction (Fig. 1, D and E) and pollen tube growth in vitro (Fig. 2, E and G). Even at 24 HAP, pollen tubes of the ap-3 mutants could hardly reach the bottom of the pistils (Supplemental Fig. S3), which explains the incidence of unfertilized ovules at the bottom of the pistils (Fig. 1).
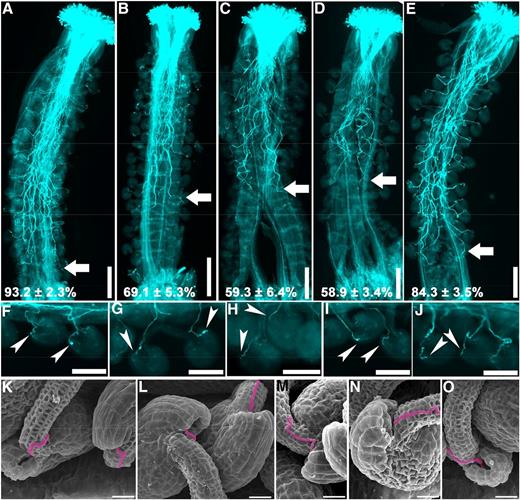
Functional loss of AP‐3 affects the growth but not the guidance of pollen tubes in vivo. A to J, Representative Aniline Blue staining of wild-type pistils emasculated and hand pollinated with pollen from the wild type (A and F), ap-3δ (B and G), ap-3β (C and H), ap-3µ (D and I), and ap-3σ (E and J) at 9 HAP. Arrows point at the front of in vivo growing pollen tubes; arrowheads point at the micropyle where pollen tubes arrive. The relative distance that pollen tubes travel within the transmitting tract is quantified with 15 pistils, and the results are shown at the bottom of A to E (means ± sd). Each mutant is significantly different from the wild type in the length of in vivo growing pollen tubes (one-way ANOVA, Dunnett’s multiple comparison test, P < 0.05). K to O, SEM images of wild-type ovules hand pollinated with pollen from the wild type (K), ap-3δ (L), ap-3β (M), ap-3µ (N), and ap-3σ (O) at 9 HAP. Pollen tubes growing toward the micropyle are false-colored pink. Bars = 200 μm for A to E, 100 μm for F to J, and 20 μm for K to O.
Despite the reduced growth, pollen tubes of ap-3 mutants were able to reach and properly target ovules that were accessible (Fig. 3, G–J), similar to the wild type (Fig. 3F). SEM of wild-type pistils emasculated and hand pollinated with ap-3 pollen also confirmed normal pollen tube guidance (Fig. 3, K–O). Because ap-3δ contained ProLAT52:GUS in the T-DNA, we could perform histochemical GUS staining of wild-type pistils pollinated with either ProLAT52:GUS pollen or ap-3δ pollen. All ovules accessible to mutant pollen tubes were targeted (Supplemental Fig. S4), confirming intact pollen tube guidance in the ap-3 mutants.
AP-3 Loss-of-Function Compromises the Vacuolar Organization of Pollen Tubes
Pollen tube growth requires dynamic vacuolar organization (Hicks et al., 2004; Wudick et al., 2014), which is likely crucial for turgor regulation and ion homeostasis. Functional loss of AP-3 affected vacuolar organization in seeds and root cells (Feraru et al., 2010; Zwiewka et al., 2011). Therefore, we were interested in determining the vacuolar organization of ap-3 mutant pollen tubes. To this purpose, we used a fluorescent fusion of the V-ATPase a3 subunit, VHA-a3-YFP, to label the tonoplast. VHA-a3 is targeted to the tonoplast via an AP-3-independent route (Viotti et al., 2013; Feng et al., 2017b). In wild-type pollen tubes, VHA-a3-labeled tubular vacuoles were extensive and penetrated to the subapical area right beneath the clear zone (Fig. 4A; Supplemental Movie S1). In contrast, VHA-a3-labeled vacuoles in ap-3σ pollen tubes were less complex and far behind the subapical region (Fig. 4B; Supplemental Movie S2). In addition to tubular structures resembling those in the wild type (Fig. 4A; Supplemental Movie S3), there were often VHA-a3-labeled ring-shaped or bulbous structures associated with tubular vacuoles in ap-3σ pollen tubes (Fig. 4B; Supplemental Movie S4). These results showed that functional loss of AP-3 compromises vacuolar organization in pollen tubes.

Vacuolar organization is compromised in ap‐3 pollen tubes. Growing wild-type (A) and ap-3δ (B) pollen tubes expressing VHA-a3-YFP are shown. From top to bottom: the YFP channel image at the midoptical plane; merge of the YFP and transmission channels; and 3D surface rendering of confocal laser scanning fluorescence micrography (CLSM) images. Dotted lines illustrate the silhouettes of the pollen tubes. Arrowheads point at the ring-shaped structures labeled with VHA-a3 and associated with the tubular vacuoles. Over 30 pollen tubes for each genotype were examined with similar results. Bars = 10 μm.
PAT10, Whose Tonoplast Association Depends on AP-3, Mediates Pollen Tube Growth
Because AP-3 functions through sorting vacuolar cargoes at the Golgi (Bassham et al., 2008; Uemura and Ueda, 2014), we questioned whether mistargeting of some AP-3 cargoes resulted in reduced pollen tube growth. In Arabidopsis, AP-3 was reported to mediate the tonoplast localization of SUC4 (Wolfenstetter et al., 2012), VAMP713 (Ebine et al., 2014), VAMP711 (Feng et al., 2017a), and PAT10 (Feng et al., 2017a). To determine whether these AP-3 cargoes were involved in pollen tube growth, we first examined their expression in mature pollen grains by RT-qPCR. We found that SUC4 was barely expressed in pollen, while PAT10 and the genes encoding R-SNAREs were expressed (Supplemental Fig. S5).
Functional loss of PAT10 showed reproductive defects, specifically reduced male transmission (Zhou et al., 2013). Although the role of PAT10 in sporophytically controlled pollen development has been characterized (Zhou et al., 2013), its roles during pollen tube growth are not known. First, we introduced PAT10g-GFP (Zhou et al., 2013) into ap-3δ and examined PAT10 targeting in ap-3δ pollen tubes. VHA-a3-RFP was coexpressed to label the tonoplast in both wild-type and ap-3δ pollen tubes. In the wild type, PAT10-GFP labeled dynamic tubular structures overlapping those of VHA-a3-RFP (Fig. 5A), indicative of the tonoplast (Hicks et al., 2004). However, PAT10-GFP was distributed into punctate vesicles in ap-3δ pollen tubes, whereas VHA-a3-RFP still showed tubular tonoplast localization (Fig. 5B). The tonoplast association of PAT10-GFP in ap-3δ pollen tubes, as well as reduced pollen tube length, were restored by introducing AP-3δg-RFP (Fig. 5, C and D), confirming AP-3δ-dependent tonoplast targeting of PAT10 and tube growth.
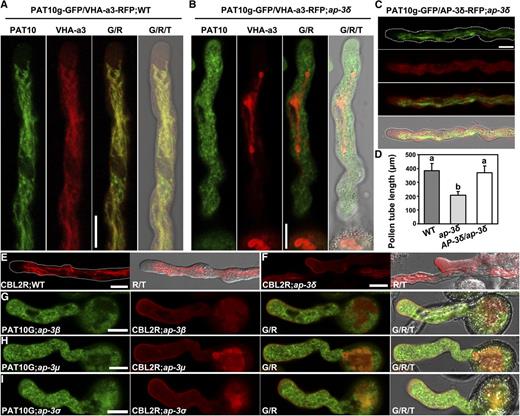
The tonoplast association of PAT10 and CBL2 relies on AP‐3 in pollen tubes. A and B, CLSM images of pollen tubes expressing PAT10g-GFP;VHA-a3-RFP in the wild type (WT; A) and in ap-3δ (B). C, CLSM images of a pollen tube expressing PAT10g-GFP in the complemented AP-3δ-RFP;ap-3δ. D, Pollen tube length. Results shown are means ± sd (n = 100). Means with different letters indicate significant differences of pollen tube length (one-way ANOVA, Dunnett’s multiple comparison test, P < 0.05). E and F, Pollen tubes stably transformed with ProUBQ10:CBL2-RFP in the wild type (E) and in ap-3δ (F). G and I, Pollen tubes stably transformed with ProUBQ10:CBL2-RFP;PAT10g-GFP in ap-3β (G), ap-3µ (H), and ap-3σ (I). G/T or R/T indicates merge of the GFP or RFP channel and the transmission channel; G/R/T indicates merge of the GFP channel, the RFP channel, and the transmission channel. Over 30 pollen tubes for each genotype were examined with similar results for data shown in A to C and E to I. Bars = 10 μm.
Next, we used PAT10g-GFP/-;pat10-2 plants for pollen tube growth studies in vitro, in which fluorescent pollen tubes were equivalent to the wild type and nonfluorescent ones were equivalent to pat10-2. Indeed, nonfluorescent pollen tubes from the PAT10g-GFP/-;pat10-2 plants were significantly shorter than GFP-expressing pollen tubes (Supplemental Fig. S6), demonstrating the role of PAT10 in pollen tube growth.
PAT10 mediates the S-acylation-dependent tonoplast association of CALCINEURIN B-LIKE2 (CBL2) and CBL3 (Zhou et al., 2013; Zhang et al., 2015). To determine whether CBL2 and CBL3 were mistargeted in the ap-3 pollen tubes due to the mistargeting of PAT10, we analyzed the subcellular localization of CBL2-RFP in each ap-3 mutant. In contrast to the tubular distribution of CBL2-RFP in wild-type pollen tubes (Fig. 5E), RFP signals were distributed into the plasma membrane and cytoplasmic vesicles in the ap-3 pollen tubes (Fig. 5, F–I). Because mutations at CBL2 and CBL3 cause defects in pollen tube growth (Steinhorst et al., 2015), these results further supported the idea that the mistargeting of PAT10 and its downstream components contributes to the reduced pollen tube growth of the ap-3 mutants.
AP-3 Loss-of-Function Compromises the Tip-Focused Ca2+ Gradient in Pollen Tubes
Pollen tube growth relies on a tip-focused Ca2+ gradient (Konrad et al., 2011; Steinhorst and Kudla, 2013), while CBL2 and CBL3 are calcium sensors functioning in pollen tube growth (Steinhorst et al., 2015). Thus, we hypothesized that ap-3 pollen tubes might be compromised in their Ca2+ gradient or signaling, leading to reduced pollen tube growth. To test this hypothesis, we introduced a ProUBQ10:YC3.6 transgene (Monshausen et al., 2008; Behera et al., 2015), which expresses the Förster (fluorescence) resonance energy transfer (FRET)-based genetically modified Ca2+ indicator Yellow Cameleon YC3.6, into ap-3δ. FRET analysis showed that, compared with wild-type pollen tubes (Fig. 6, A and C), growing ap-3δ pollen tubes exhibited a significantly reduced Ca2+ gradient at the tip (Fig. 6, B and C), consistent with the reduced growth of mutant pollen tubes.
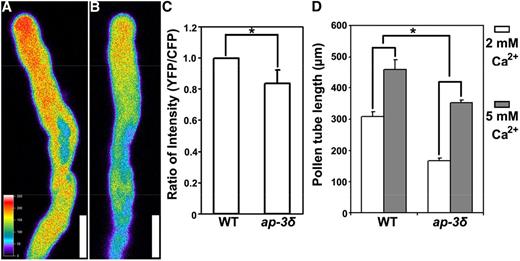
AP‐3 loss-of-function compromises the tip‐focused Ca2+ gradient. A and B, CLSM images of pollen tubes expressing the Ca2+ sensor YC3.6 in the wild type (A) and in ap-3δ (B). Images are representative of 20 pollen tubes for each genotype. Cytosolic Ca2+ levels were calibrated as described in “Materials and Methods” and pseudocolored according to the scale at the left. Bars = 10 μm. C, Quantification of fluorescence intensity as the ratio between YFP and CFP signals. Results are means ± sd (n = 20). The asterisk indicates a significant difference (Student’s t test, P < 0.01). D, Pollen tube length after 4 h of germination in medium containing 2 or 5 mm Ca2+. Results are means ± sd (n = 100). The asterisk indicates significantly different responses of pollen tube growth upon increased exogenous Ca2+ levels (Student’s t test, P < 0.01). WT, Wild type.
To provide further evidence that a reduced Ca2+ gradient resulted in compromised pollen tube growth in the ap-3 mutants, we tested the effect of enhanced Ca2+ concentration by supplementing the growth medium with a higher concentration of extracellular Ca2+ using 5 mm instead of 2 mm Ca2+ in the regular germination medium. Although both wild-type and ap-3δ pollen tubes responded to the increased Ca2+ by enhanced tube growth (Fig. 6D), ap-3δ pollen tubes were more sensitive than those of the wild type. The ap-3δ pollen tubes doubled their length after 4 h of germination, whereas those of the wild type only increased around 40% (Fig. 6D). This result supports the idea that there is reduced Ca2+ signaling in ap-3δ pollen tubes.
DISCUSSION
The roles of AP-1 and AP-2 in plants have been well characterized, especially during reproductive processes (Fan et al., 2013; Park et al., 2013; Wang et al., 2016). Here, we demonstrated that AP-3, the AP complex that sorts proteins at the Golgi to vacuoles, mediates pollen tube growth and male fertility (Figs. 1 and 2). Mutants in each AP-3 component showed reductions of pollen tube growth both in vitro and in vivo (Fig. 3), suggesting that AP-3 functions as a complex. The phenotypic defects of ap-3σ were the weakest (Figs. 2 and 3). Because the σ-subunit stabilizes AP complexes (Boehm and Bonifacino, 2001; Robinson, 2004), we speculate that this subunit is not essential for AP-3. Alternatively, the σ-subunits of other APs may perform redundant roles as a compensation mechanism.
Among the established tonoplast cargoes that are sorted by AP-3 during their vacuolar trafficking, PAT10 is an important player in pollen tube growth (Fig. 5; Supplemental Fig. S6); functional loss of PAT10 affected male fertility (Zhou et al., 2013). By using sporophytically complemented transgenic materials, we demonstrated that PAT10 functions in male gametophytes to promote pollen tube growth (Supplemental Fig. S6). CBL2 and CBL3, two calcium sensors, rely on PAT10 for their tonoplast association and functionality (Zhou et al., 2013; Zhang et al., 2015). Indeed, the functional loss of both CBL2 and CBL3 impaired male fertility (Steinhorst et al., 2015). The CBL2/CBL3 study was performed using a homozygous cbl2 cbl3 mutant in which sporophytic defects would have enhanced the defects of pollen tube growth (Steinhorst et al., 2015). Nevertheless, these results suggest a key role for the tonoplast calcium sensors in pollen tubes. Indeed, by FRET analysis of the YC3.6 probe, we demonstrated that growing pollen tubes with a functional loss of AP-3 contained a reduced Ca2+ gradient (Fig. 6), which is consistent with the reduced pollen tube growth (Hepler et al., 2012).
The functional loss of AP-3 caused not only mistargeting of PAT10, which is important for pollen tube growth, but also defects in dynamic vacuolar organization (Fig. 4). By using the AP-3-independent tonoplast protein VHA-a3, we demonstrated that pollen tubes of the ap-3 mutants contained a simplified structure of vacuoles (Fig. 4). Unlike the extensive tubular vacuoles in wild-type pollen tubes, which extended to the subapical region right behind the apex, those in ap-3 pollen tubes were excluded from the subapical zone (Fig. 4). Indeed, mutations in other AP-3 subunits also resulted in defects in the morphology and function of lytic vacuoles in root cells (Feraru et al., 2010; Zwiewka et al., 2011). Because of the key role of vacuoles in providing turgor pressure and facilitating ion homeostasis, such defective vacuolar organization might have contributed to the reduction in pollen tube growth.
Despite the role of AP-3 in pollen tube growth, defects of ap-3 mutants have low penetrance (Table I), whereas mutations at the homotypic fusion and vacuolar protein sorting complex, involved in vesicle-vacuole fusion, resulted in male gametophytic lethality (Hao et al., 2016). A likely explanation is that compensating pathways of vacuolar trafficking, such as Rab5-mediated (Cui et al., 2014; Ebine et al., 2014; Singh et al., 2014) and AP-4-mediated (Fuji et al., 2016) pathways, might help to maintain the dynamic organization of vacuoles and provide ion homeostasis.
MATERIALS AND METHODS
Plant Materials, Growth, and Transformation
The T-DNA insertion lines ap-3δ/pat4-2 (SALK_069881; Niihama et al., 2009; Zwiewka et al., 2011), ap-3β/pat2-2 (SAIL_1258_G03; Niihama et al., 2009; Feraru et al., 2010), ap-3µ (SALK_064486; Niihama et al., 2009), and ap-3σ (SAIL_269_F04) were obtained from the Arabidopsis Biological Resource Center. Other materials, including ProLAT52:GUS (Li et al., 2013), ProUBQ10:YC3.6 (Behera et al., 2015), PAT10g-GFP (Zhou et al., 2013), ProUBQ10:VHA-a3-YFP (Feng et al., 2017), and ProUBQ10:CBL2-RFP (Zhang et al., 2015), were described previously. The Arabidopsis (Arabidopsis thaliana) Columbia-0 ecotype was used as the wild type. Transgenic plants were selected on one-half-strength Murashige and Skoog medium supplemented with 30 µg mL−1 Basta salts (Sigma-Aldrich) or 50 µg mL−1 hygromycin (Roche).
RT-qPCR
The extraction of total RNA, reverse transcription, and RT-qPCR were performed as described (Zhou et al., 2013). Primers for all AP-3 mutants have been described (Feng et al., 2017a). Primers used for RT-qPCR were ZP5314/ZP5315 for VAMP711, ZP5316/ZP5317 for VAMP712, ZP5318/ZP5319 for VAMP713, ZP5363/ZP5364 for SUC4, and ZP691/ZP692 for PAT10. Primers for GAPDH and TUBULIN2 were as described (Zhou et al., 2013). All primers are listed in Supplemental Table S1.
DNA Constructs
Primers used for cloning of AP-3δ are ZP4427/ZP4428. The entry vector for AP-3δ was generated in the pENTR/D/TOPO backbone (Invitrogen). The entry vectors for VHA-a3 (Feng et al., 2017) and CBL2 (Zhang et al., 2015) were described previously. Expression vectors were generated by combining entry vectors and the destination vector ProUBQ10:GW-RFP (Zhang et al., 2015) in LR reactions using LR Clonase II (Invitrogen). PCR amplifications used Phusion hot-start high-fidelity DNA polymerase with the annealing temperature and extension times recommended by the manufacturer (Thermo Fisher). Entry vectors were sequenced, and sequences were analyzed using Vector NTI. All primers are listed in Supplemental Table S1.
Phenotypic Analysis of Pollen Development and Tube Growth
Methods to analyze pollen development, including Alexander staining, 4′,6-diamino-phenylindole staining, and SEM, were performed as described (Johnson-Brousseau and McCormick, 2004; Li et al., 2013). Methods to analyze pollen tube growth in vitro were performed as described (Boavida and McCormick, 2007). Methods to analyze pollen tube growth in vivo by Aniline Blue staining and by histochemical GUS staining were performed as described (Li et al., 2013).
Fluorescence Microscopy
Fluorescent images were captured using a Zeiss LSM 880 confocal laser scanning microscope with a 40/1.3 oil objective. GFP and YFP fusions were excited at 488 nm with a VIS-argon laser; RFP fusions were excited at 561 nm with a VIS-DPSS561 laser diode. Pollen tubes double labeled with GFP and RFP fusions were captured using alternate line switching mode with a multitrack function. Fluorescence was detected using a 505- to 550-nm band-pass filter for GFP/YFP/OG or a 575- to 650-nm band-pass filter for RFP. Z-stack images were recorded with a step size of 0.3 μm, image dimensions of 512 × 512, and pinhole at 1 airy unit. Time-lapse imaging for generating movie clips was performed as an interval of 0.5 s Each movie clip was generated from 100 slides of still images. Image processing was performed with the Zeiss LSM image-processing software. 3D surface renderings were performed with Imaris7.0 software.
Accession Numbers
Arabidopsis Genome Initiative locus identifiers for the genes mentioned in this article are as follows: AP-3δ, At1g48760; AP-3β, At3g55480; AP-3µ, At1g56590; AP-3σ, At3g50860; CBL2, At5g55990; PAT10, At3g51390; SUC4, At1g09960; VAMP711, At4g32150; VAMP712, At2g25340; VAMP713, At5g11150; and VHA-a3, At4g39080.
Supplemental Data
The following supplemental materials are available.
Supplemental Figure S1. Functional loss of AP-3 does not compromise pollen development.
Supplemental Figure S2. Functional loss of AP-3 reduces pollen tube growth in vitro.
Supplemental Figure S3. Functional loss of AP-3 reduces pollen tube growth in vivo.
Supplemental Figure S4. Functional loss of AP-3δ does not impair pollen tube guidance.
Supplemental Figure S5. Expression of genes encoding cellular cargos of AP-3 in pollen.
Supplemental Figure S6. AP-3-mediated tonoplast protein PAT10 is important for pollen tube growth.
Supplemental Table S1. Oligonucleotides used in this study.
Supplemental Movie S1. Vacuolar dynamics in a wild-type pollen tube.
Supplemental Movie S2. Vacuolar dynamics in an ap-3δ pollen tube.
Supplemental Movie S3. 3D surface rendering of VHA-a3-YFP-labeled vacuoles in a wild-type pollen tube.
Supplemental Movie S4. 3D surface rendering of VHA-a3-YFP-labeled vacuoles in an ap-3δ pollen tube.
ACKNOWLEDGMENTS
We thank the Arabidopsis Biological Resource Center for plant materials. We are grateful for language editing by Sheila McCormick.
LITERATURE CITED
Author notes
This work was supported by the Natural Science Foundation of China (31625003 and 31471304 to Y.Z., 31771558 to S.L.), by the Natural Science Foundation of Shandong Province (ZR2014CM027 to S.L.), and by the China Postdoctoral Science Foundation (2015M570605 and 2016T90643 to S.L.). Y.Z.’s laboratory is partially supported by the Tai-Shan Scholar Program of the Shandong Provincial Government.
Address correspondence to [email protected].
The author responsible for distribution of materials integral to the findings presented in this article in accordance with the policy described in the Instructions for Authors (www.plantphysiol.org) is: Yan Zhang ([email protected]).
Y.Z. conceived and supervised the project; Q.-N.F. performed the experiments with the assistance of X.L.; Y.Z., S.L., and Q.-N.F. designed the experiments and analyzed the data; Y.Z. and Q.-N.F. wrote the article.
Articles can be viewed without a subscription.